Abstract
The multitude of factors has contributed to the increasing number of fungal infections caused by species of difficult-to-treat opportunistic moulds, such as Fusarium, Scedosporium, and cryptic Aspergilli. Also, rare fungi sporadically encountered, such as Rasamsonia argillacea, Penicillium oxalicum, and melanized fungi, are now well recognized. The high mortality associated with these rare and uncommon fungi is primarily linked to the difficulty in diagnosis and limited therapeutic options, as many of them exhibit resistance to antifungals including azoles. Azole resistance in Aspergillus fumigatus has been increasingly reported because standardized methods for susceptibility testing and associated clinical breakpoints and epidemiological cutoff values became available. However, such advances in antifungal susceptibility testing (AFST) in non-Aspergillus moulds barring mucorales have been lacking. Notwithstanding the fact that the true incidence of these non-Aspergillus filamentous moulds in clinical settings is hitherto unknown, also data on AFST by standardized methods is largely lacking. Determination of minimum inhibitory concentration (MIC) by reference techniques is the gold standard to detect azole resistance in filamentous fungi. In recent years, some progress has been made toward the description of resistance mechanisms at molecular level especially in Aspergillus. This paper reviews the present state of azole resistance in Aspergillus and other filamentous mould species and discusses their relevance to clinical practice.
Similar content being viewed by others
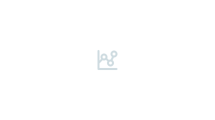
Avoid common mistakes on your manuscript.
Introduction
Filamentous fungi encompassing many genera are associated with a wide spectrum of diseases in humans ranging from superficial to life threatening invasive infections. The multitude of factors has contributed to the increasing number of fungal infections in the last two decades, especially caused by species of opportunistic moulds for which there is no reliable medical therapy. The factors mainly include increasing use of immunosuppressing agents, selection of these moulds in the setting of antifungal prophylaxis, natural disasters, and their better recognition due to advanced identification methods [1, 2, 3••]. The incidence of mould infections is much lower than candidiasis; however, infections due to filamentous fungi are a significant cause of morbidity and mortality especially among immunocompromised patients [4]. Also, the epidemiology of mycoses associated with several filamentous fungi has changed such that the most prevalent invasive mould infection is primarily due to Aspergillus fumigatus, but the global emergence of azole-resistant strains has been described in the last decade [5]. Also, infections caused by difficult-to-treat moulds, such as species of Mucorales [6], Fusarium [7], and Scedosporium [8], are increasingly reported, although true incidence of these non-Aspergillus filamentous moulds is hitherto unknown. Furthermore, rare fungi sporadically encountered, such as Rasamsonia argillacea, Penicillium oxalicum, and melanized fungi are now well recognized [9, 10].
The high mortality associated with the rare and uncommon fungi is primarily linked to the difficulty in diagnosis, limited therapeutic options, and a lack of knowledge of the most effective antifungal therapy. In recent years, the challenges pertaining to identification are significantly reduced by availability of molecular tools and mass spectrometry, which is particularly helpful for the species level identification of fungi with high accuracy and also for determining their resistance profile to antifungals [11, 12••]. However, the therapeutic options for invasive fungal infections remain limited and include only three structural classes of drugs: polyenes, azoles, and echinocandins. Among antifungals, the better tolerated azoles and echinocandins have emerged as first-line agents for most common invasive fungal infections [13]. The azoles, namely fluconazole (FLU), voriconazole (VRC), and posaconazole (POS), are the most widely used antifungal to treat invasive fungal infections. Azoles inhibit ergosterol biosynthesis and, in general, are fungistatic. However, VRC is fungicidal toward A. fumigatus. Notably, FLU has essentially no activity against moulds. In contrast, itraconazole (ITC), VRC, POS, and recently approved isavuconazole (ISA) all have activity against moulds. Therefore, the emergence of azole antifungal resistance in moulds jeopardizes the effective treatment. The other major challenge in this respect is that antifungal susceptibility testing (AFST) is not routinely performed in many centers in the world. The true rates of global azole resistance in these pathogens are enigmatic; therefore, detection and monitoring of azole resistance are of paramount importance for the effective management of the disease. The present review aims to provide synopsis of azole resistance in clinically significant opportunistic filamentous mould species and discusses the approach of detection of azole resistance in these fungi.
Global Emergence of Azole Resistance in Aspergillus Species
The last decade has witnessed shift in the etiology of aspergillosis and highlighted the emergence of cryptic and rare Aspergillus species in various clinical settings in both immunocompromised and immunocompetent hosts [14]. The application of multilocus DNA sequence analysis in various studies has indicated the prevalence of previously unknown “cryptic” Aspergillus species in clinical specimens [15, 16]. In two population based prospective studies in the USA and Spain, the prevalence of cryptic Aspergillus species detected in clinical specimens was found to be 10 and 12 %, respectively [17, 18]. Aspergillus species belonging to the section Fumigati (A. fumigatus complex) are often misidentified as they cannot be distinguished from A. fumigatus by conventional methods. Furthermore, these species often display intrinsic resistance to azoles and other antifungal drugs. A. lentulus, A. udagawae, A. viridinutans, and A. thermomutatus (Neosartorya pseudofischeri) have been associated with refractory cases of invasive aspergillosis (IA) [16, 19, 20]. Azole resistance in Aspergillus species is not restricted to section Fumigati but several species in other sections also exhibit elevated minimum inhibitory concentrations (MICs) for azoles and are listed section wise in Table 1.
Primarily, ITC is used in the treatment of chronic pulmonary aspergillosis (CPA) [21], while VRC is used as first line therapy of IA [22]. Recently another azole, ISA, has been approved for the primary treatment of IA [23]. POS is indicated as prophylaxis in high-risk patients, such as acute myeloid leukemia (AML) and stem cell transplant patients with graft-versus-host-disease (GVHD) [22]. However, in recent years, emergence of azole resistance in clinically relevant filamentous moulds due to prolonged azole exposure had led to increasing reports of treatment failure and breakthrough infections. Such development of azole resistance in chronic aspergillosis patients while on prolonged azole antifungals has been well documented [24]. Regarding azole resistance in A. fumigatus, it was first observed in collection of the late 1980s isolates in the USA from two patients treated with ITC [25]. Later, azole resistance in A. fumigatus was reported from the Netherlands in the 2000s with an annual prevalence ranging from 1.7 to 6 % [26•], followed by the UK reporting 28 % of azole-resistant A. fumigatus (ARAF) isolates in 2008 and 2009 corresponding to 14 and 20 % of patients, respectively [27]. Surveillance studies presently suggest the global presence of azole resistance in A. fumigatus and include reports from Europe, the Middle East, Asia, Africa, Australia, and most recently, North and South America [28••, 29••, 30]. Azole resistance in A. fumigatus is frequently the result of mutations in the cyp51A gene. Several non-synonymous point mutations at codons G54, M220, and G138 in this gene are primarily found in patients treated long-term with azoles [31]. Many of these mutations result in resistance to multiple anti-Aspergillus triazole antifungals [31]. However, in contrast to the point mutations observed in the host development route of azole resistance, in the environmental driven route A. fumigatus strains have in addition to the mutations in the cyp51A gene, a tandem repeat duplication in the promoter region, which increases the expression of the gene [31]. To date, two resistance mechanisms TR34/L98H and TR46/Y121F/T289A are reported to be associated with the environmental A. fumigatus isolates from soil and air samples [30, 31]. The patients directly acquire these azole-resistant isolates from the environment by inhalation, and in high-risk patients may result in life-threatening azole-resistant IA. The environmental selection of ARAF isolates is suggested to be a consequence of the wide use of triazole fungicides in agriculture, the latter being highly similar in their structures, as proven by homology modeling, to those used in medicine [3••, 32••]. Recently, point mutations, G54 and M220, in cyp51A gene of A. fumigatus environmental isolates were reported from India, Romania, Tanzania, and Germany [28••, 29••].
Detection of Azole Resistance in Aspergillus
The patients who have a positive Aspergillus culture and the clinical intention is to treat requires determination of the species level complex of the isolate. Morphological methods are not always useful in species identification of rare and uncommon Aspergillus isolates. Correct identification of species with in the section Fumigati is clinically relevant specifically in context of A. lentulus that appears to have higher in vitro MICs to azoles compared to A. fumigatus, thus altering the therapeutic decisions [19]. The major molecular taxonomic tool, ribosomal sequencing, is widely used for fungal identification. The use of internal transcribed spacer regions (ITS) for inter-section-level identification and the β-tubulin locus for identification of individual species within the various Aspergillus sections is recommended [16, 33, 34]. The AFST is very crucial in settings where antifungal therapy is to be initiated in determining not only the best clinically active antifungal agent but also to detect resistance in Aspergillus. The Clinical Laboratory Standard Institute (CLSI) and the European Committee on Antimicrobial Susceptibility Testing (EUCAST) methods based on broth microdilution are highly reproducible and recommended to detect in vitro resistance against triazoles in filamentous fungi. EUCAST has published MIC breakpoints for ITC (>2 μg/ml), VRC (>2 μg/ml), and POS (>0.25 μg/ml) for defining resistance in A. fumigatus [35]. Although break points are not available for CLSI method, epidemiological cutoff values (ECVs) of ITC (1 μg/ml), VRC (1 μg/ml), and POS (0.25 μg/ml) for A. fumigatus and other five clinically relevant Aspergillus species have also been established [36]. Recently, the ECVs for the ISA using CLSI M38-A2 broth microdilution method and EUCAST have been described as 1 and 2 μg/ml, respectively, for A. fumigatus [37, 38]. It is recommended to perform in vitro susceptibility testing on multiple colonies, as different azole susceptibility phenotypes might be present in a single culture [39].
The major lacuna in detecting azole resistance is the problem that MIC determination of Aspergillus is not performed routinely in many microbiological laboratories worldwide. A simple agar-based screening method containing four-well plate with a growth control and ITC, VRC, and POS added to the agar [40] (VIP Check TMBeneden-Leeuwen, the Netherlands) has the advantage of selecting ARAF strains. The incorporation of this screening approach in laboratories may result in isolating potential resistant isolates that could be sent to referral laboratories for AFST and to identify the resistant mechanisms.
Direct Detection of Azole Resistance in Culture Negative Clinical Samples
A few studies have reported direct detection of mutations in culture-negative clinical samples using real time PCR assays [41, 42]. Recently, a multiplex real-time PCR for detection of two environment associated resistance mechanisms, i.e., TR34/L98H and TR46/Y121F/T289A and for detection of Aspergillus species has become available (AsperGenius®, PathoNostics, Maastricht, the Netherlands). In the hematologic malignancies, the authors reported sensitivity and specificity of 88.9 and 89.3 %, respectively, and in intensive care unit patients 80 and 93.3 %, respectively [43]. Considering that the AsperGenius-PCR allows identification of only two mechanisms of resistance, therefore, a negative test result does not rule out the presence of azole resistance.
Molecular Mechanism of Azole Resistance in A. fumigatus
The characterization of two different sterol demethylase genes (Cyp51A and Cyp51B) in A. fumigatus have led to the description of mutations in cyp51A leading to azole resistance. A number of mutations have been described that confers different resistance profiles in Aspergillus. Various point mutations cause structural changes in enzyme’s active site causing decreased affinity for its ligands [44]. These mutations have been associated with resistance to one or two azoles (G54, M220) or may confer cross-resistance [29••, 45]. Resistance due to the tandem repeat coupled with mutation L98H or Y121F/T289A in cyp51A has been proven to upregulate the enzyme expression [46, 47•]. In addition, mutations at cyp51 gene have been related to disturbances in protein structure causing alteration in the active site of docking of the antifungal agent thereby leading to increase MICs. Until 2008, azole resistance in A. fumigatus was primarily attributed to mutations in cyp51 gene; however, in 2010, Manchester reference laboratory, UK demonstrated that 43 % of the ARAF isolates were without any mutation in the cyp51A gene [27]. Apart from the abovementioned mutations, the genetic disturbances, such as overexpression of ABC or MFS efflux pumps such as atrF, cdr1B, Cyp51B overexpression and incorporation of exogenous cholesterol into A. fumigatus plasma membranes, have been well studied in A. fumigatus [31]. Also, another mutation in CCAAT-binding transcription factor complex subunit HapE, resulting in azole resistance, have been reported in A. fumigatus [48•]. Furthermore, cyp51A expression modulated by insertion of an Aft1 transposon 370 bp upstream of the start codon has been reported [49]. A solitary study determined the azole MICs of 50 black Aspergilli (section Nigri) using modified EUCAST and Etest methods and compared the results with cyp51A sequences. ITC resistance was observed in 51 % of the clinical isolates, but azole cross-resistance was unusual. The authors found G427S and K97T mutations in cyp51A gene of black Aspergilli, which warrant further investigations [50].
Azole Resistance in Non-Aspergillus Moulds
The epidemiology of non-Aspergillus mould infections is changing probably due to the wide use of molecular and proteomic diagnostic methods; some moulds previously not reported in the literature are reported to cause invasive diseases suggesting the notion of emergence. In the last decade, environmental filamentous ascomycetes other than Aspergillus species are increasingly reported as agents of invasive diseases specifically in profoundly immunosuppressive patients, such as during prolonged neutropenia, GVHD, and in rejection episodes among solid organ transplant (SOT) patients. They include the relatively more commonly reported species of Fusarium and Scedosporium and rarely encountered species of Acremonium, Penicillium, and Rasamsonia [51, 52]. Infections with these fungi are lethal because the hosts they usually infect are incapable of mounting an effective immune response and because they tend to be resistant to antifungals. Table 2 lists species of filamentous moulds reported to exhibit elevated MICs of azoles and are discussed below:
Intrinsic Azole Resistance in Species of Fusarium
Fusarium spp. are widespread filamentous fungi that are primarily soil saprophytes and plant pathogens. In humans, 74 species of Fusarium are incriminated to cause infections, but the most commonly reported species include Fusarium solani complex, F. oxysporum complex, and F. (Giberella) fujikuroi complex, which include among others F. verticillioides and F. proliferatum. Also, to a lesser extent, both F. dimerum and F. incarnatum-equiseti species complex (SC) have been reported [52, 53]. They cause wide spectrum of infections, ranging from mildly superficial to fatally disseminated disease especially in patients with profound and prolonged neutropenia and/or T cell immunodeficiency [51, 53–57]. Fusariosis is highly fatal some reports suggesting 30 % survival rates, especially among patients with persistent neutropenia [51, 58, 59]. Fusarium species are intrinsically resistant to azole antifungals, and some clinically relevant species are also resistant to almost all currently used antifungals, including echinocandins and polyenes [60] (Table 2).
CLSI subcommittee on AFST in the M38-A2 document includes reproducible procedure for testing the antifungal susceptibilities of Fusarium spp. However, species-specific clinical breakpoints (BPs) have not been established for this pathogen due to lack of both clinical trials and knowledge about the molecular resistance mechanisms. Recently, ECVs have been established for important Fusarium species and azoles including the highest ECVs for the three triazoles and F. solani SC (32 μg/ml). Lower POS and VRC ECVs were reported for F. verticillioides (2 and 4 μg/ml, respectively) and F. oxysporum SC (8 and 16 μg/ml, respectively) [61]. These triazole ECVs are higher than the dose dependent trough levels of azole antifungals and highlight the intrinsic resistant nature of Fusarium spp. [62]. It is emphasized that ECVs are not BPs, therefore, cannot envisage clinical response to therapy but predict those isolates that are more likely to harbor acquired molecular mutations conferring resistance. The molecular resistance mechanism in Fusarium is not understood, but combination of CYP51A amino acid alteration or overexpression may be involved. Recently, Fan et al. showed that CYP51 in Fusarium has three paralogues (CYP51A, CYP51B, and CYP51C), with CYP51C being unique to the genus and CYP51A deletion increases the sensitivity of F. graminearum to azoles [63].
Scedosporium Species
Scedosporium spp. are distributed in the environment as inhabitants of soil, polluted water, and animal excreta [64]. Scedosporium apiospermum complex and Lomentospora prolificans (previously S. prolificans) account for most infections prevalent worldwide and are associated with poor clinical outcomes [65]. The three main species within S. apiospermum complex are S. apiospermum, S. boydii, and S. aurantiacum. The complex also encompasses uncommon species, such as S. minutispora and S. dehoogii [66]. The fungus affect diverse patient population with varied clinical manifestations and risk factors include chronic obstructive lung disease, hematologic malignancy, SOT or hematopoietic stem cell transplantation (HSCT), corticosteroid use, neutropenia, and diabetes mellitus [65]. Disseminated infections associated with high mortality are typically caused by L. prolificans in immunocompromised hosts [67–69]. Recently described S. aurantiacum colonizes or infect the respiratory tract of patients with cystic fibrosis and other chronic lung disease [68]. The species-specific differences in virulence and AFST patterns have been reported in S. apiospermum complex and L. prolificans necessitating species level identification of the causative agent [66]. Species identification requires sequencing of the β-tubulin, β-actin, and calmodulin gene targets [70•].
The majority of Scedosporium isolates exhibit multiple antifungal resistance including azoles, and data on species-specific susceptibility patterns is limited (Table 2). A comprehensive study on AFST of 332 molecularly identified Scedosporium isolates demonstrates that L. prolificans exhibits the highest GM MICs of all antifungal drugs including azoles (ITC GM MIC, 32 μg/ml; VRC GM MIC, 15.4 μg/ml; POS GM MIC, 32 μg/ml; and ISA GM MIC, 25.6 μg/ml) [71••]. All S. apiospermum and S. boydii were found to have high MIC values of AMB (MIC50 4 μg/ml), ITC (MIC50 16 g/ml), and ISA (MIC50 >4 μg/ml). POS and VRC are the most promising drugs against Scedosporium species other than L. prolificans. Limited in vitro activity of VRC was found only for L. prolificans and S. dehoogii. Furthermore, S. aurantiacum also exhibits elevated MICs for ITC, POS, and ISA. Overall, VRC has activity against S. apiospermum, S. boydii, and S. aurantiacum [71••]. The consequence of varied MICs for azoles reflects that the susceptibilities of individual isolates are difficult to predict, and thus, susceptibility testing of clinical isolates remains essential for targeted treatment. It is emphasized that clinical breakpoints, ECV, or molecular resistance mechanism for Scedosporium are not yet elucidated; therefore, interpretation of MIC testing remains difficult. Concordance among in vitro resistance profiles and in vivo outcome has been reported [72], and VRC treatment of L. prolificans infections showed a 40 % clinical response despite an MIC50 of 4 μg/ml [67].
Rare and Emerging Species of Rasamsonia, Paecilomyces, Penicillium, and Acremonium
Rasamsonia argillacea, previously known as Geosmithia argillacea, is an emerging pathogen that in the past has been misidentified as Penicillium or Paecilomyces species. Rasamsonia argillacea complex causes pulmonary infection in chronic granulomatous disease patients [73]. Also, fatal infection in stem cell transplant patients has been reported [74]. AFST reveals that species belonging to Rasamsonia complex are resistant to VRC (Table 2) and variably resistant to ITC, amphotericin B, and POS [9, 75]. Several studies highlight that R. argillacea infection to be ruled out in patients whose fungal infections worsen and whose cultures are reported as Penicillium species, especially if these patients are receiving VRC [9]. Similarly, antifungal susceptibility profiles of Paecilomyces variotii and other species of Paecilomyces including P. formosus, P. dactylethromorphus, and P. divaricatus are reported to be VRC resistant [76]. Paecilomyces infections are uncommon, but serious manifestations include pneumonia, sinusitis, osteomyelitis, disseminated infection, and fungemia [77, 78, 79•]. VRC-resistant P. oxalicum break through infections have recently been reported in AML and in chronic aspergillosis patients while on VRC therapy [10].
Another genus that seems to be emerging and show limited VRC activity as in the abovementioned moulds is Acremonium, which includes about 150 species but only a few implicated as human pathogens [80, 81]. The most common species are A. kiliense and A. falciforme, and others including A. roseogriseum, A. strictum, A. patronii, and A. recifei are reported as opportunistic pathogens mainly affecting immunocompromised hosts following HSCT, SOT, and hematologic malignancies. The fungus causes varied manifestations including pneumonia, arthritis, osteomyelitis, endocarditis, meningitis, peritonitis, and fungemia [82••]. The limited in vitro AFST data show high MICs for POS, VRC, and ITC (Table 2) when compared to those found for other hyaline molds, such as A. fumigatus [83].
Melanized Fungi and Azoles
Melanized fungi are ubiquitous saprophytic fungi in the environment. Their prevalence estimated in clinical samples showed only 10 % of them being clinically significant [84]. However, their clinical significance in the last few years is increasing not only in immunocompetent individuals but also in immunocompromised patients. Their clinical manifestations span from respiratory tract inflictions like allergies, superficial infections to fatal disseminated cases [85••]. In SOT subjects, dark pigmented fungi are recognized as most recent emerging opportunistic pathogens. In SOT recipients, species of Verrucosa and Ochroconis are mainly reported from lung and liver transplants patients. Emergence of melanized fungi in pathologies requires the use of accurate diagnostic tools, such as molecular methods. The limited data on the in vitro AFST of melanized fungi are available in the literature, mainly inferred from clinical cases, with potential variations due to the use of different methodologies [86]. It is emphasized that there are no defined BPs and no established correlation between MIC and clinical outcome, and also, the marked differences in the in vitro susceptibility results both at the genus and at the species levels reflect the phylogenetic diversity of these fungi. Therefore, presently exploring azole resistance in melanized moulds is challenging. Table 2 lists melanized fungi reported to exhibit elevated azole antifungal MICs.
Perspectives and Conclusions
Intrinsic or acquired antifungal resistance in pathogenic fungi may be encountered in both antifungal drug exposed and antifungal drug-naive patient. Furthermore, prior antifungal treatment confers a selection pressure and increases the possibility of resistance in patients failing therapy. Thus, in both scenarios, detection of resistant isolates requires appropriate and carefully performed susceptibility testing and endpoint interpretation. Intrinsically resistant species can be diagnosed through correct species identification, but their identification is challenging using phenotypic methods, as non-Aspergillus moulds may poorly sporulate, e.g., Fusarium and Scedosporium species complexes on routine media. As discussed above, molecular identification tests could reliably identify the isolates but are cumbersome and not performed in routine microbiology laboratories. Nevertheless, with the availability of isolate, less cumbersome mass spectrometric species identification is possible. Identification of fungi using matrix-assisted laser desorption ionization time-of-flight (MALDI-TOF) MS is rapid and potentially economical compared to sequence-based technologies, after equipment is purchased. However, current commercial MALDI-TOF MS reference databases contain a limited number of filamentous fungal spectra. Thus, substantial augmentation of the spectral library is required for routine laboratory and several studies have highlighted the importance of in-house database creation for species of Aspergillus, Fusarium, and Scedosporium filamentous fungi for reaching a consensus between proteomic and sequence-based identifications [12••, 34, 87••]. It is emphasized that without the creation of a highly stringent supplemental database, MALDI-TOF MS analysis is often unable to achieve species, and sometimes genus level identification compared to that of sequencing.
Regarding the progress made toward the description of azole resistance mechanisms at molecular level, barring A. fumigatus, the underlying mechanism remains unknown in a number of resistant filamentous moulds. MIC determination is still the most reliable procedure for surveillance of azole resistance in clinical isolates; however, molecular methods allowing detection of resistance warrants further standardization of techniques for effective integration in the routine laboratories. Also, standardized techniques detecting azole resistance in culture-negative specimens for rapid diagnosis and effective therapy need to be developed. Finally, regarding filamentous fungi, continued efforts to improve the reliability of AFST followed by analysis of the prevalence of resistance at molecular level are warranted.
References
Papers of particular interests, published recently, have been highlighted as: • Of importance •• Of major importance
Neblett Fanfair R, Benedict K, Bos J, Bennett SD, Lo YC, Adebanjo T, et al. Necrotizing cutaneous mucormycosis after a tornado in Joplin, Missouri, in 2011. N Engl J Med. 2012;367:2214–25.
Brandt ME, Park BJ. Think fungus—prevention and control of fungal infections. Emerg Infect Dis. 2013;19:1688–9.
Chowdhary A, Kathuria S, Xu J, Meis JF. Emergence of azole-resistant Aspergillus fumigatus strains due to agricultural azole use creates an increasing threat to human health. PLoS Pathog. 2013;9:e1003633. Highlights the role of fungicides in emergence of azole resistance in A. fumigatus strains.
Brown GD, Denning DW, Gow NA, Levitz SM, Netea MG, White TC. Hidden killers: human fungal infections. Sci Transl Med. 2012;4:165rv13.
Steinbach WJ, Marr KA, Anaissie EJ, Azie N, Quan SP, Meier-Kriesche HU, et al. Clinical epidemiology of 960 patients with invasive aspergillosis from the PATH Alliance registry. J Infect. 2012;65:453–64.
Lanternier F, Dannaoui E, Morizot G, Elie C, Garcia-Hermoso D, Huerre M, et al. A global analysis of mucormycosis in France: the RetroZygo Study (2005–2007). Clin Infect Dis. 2012;54:S35–43.
Muhammed M, Coleman JJ, Carneiro HA, Mylonakis E. The challenge of managing fusariosis. Virulence. 2011;2:91–6.
Cortez KJ, Roilides E, Quiroz-Telles F, Meletiadis J, Antachopoulos C, Knudsen T, et al. Infections caused by Scedosporium spp. Clin Microbiol Rev. 2008;21:157–97.
Doyon JB, Sutton DA, Theodore P, Dhillon G, Jones KD, Thompson EH, et al. Rasamsonia argillacea pulmonary and aortic graft infection in an immune-competent patient. J Clin Microbiol. 2013;51:719–22.
Chowdhary A, Kathuria S, Agarwal K, Sachdeva N, Singh PK, Jain S et al, Voriconazole resistant Penicillium oxalicum: an emerging pathogen in immunocompromised hosts. Open Forum Infect Dis. 2014; doi:10.1093/ofid/ofu029
Singh PK, Kathuria S, Agarwal K, Gaur SN, Meis JF, Chowdhary A. Clinical significance and molecular characterization of nonsporulating molds isolated from the respiratory tracts of bronchopulmonary mycosis patients with special reference to basidiomycetes. J Clin Microbiol. 2013;51:3331–7.
Sleiman S, Halliday CL, Chapman B, Brown M, Nitschke J, Lau AF, et al. Performance of matrix-assisted laser desorption ionization-time of flight mass spectrometry for the identification of Aspergillus, Scedosporium, and Fusarium spp. in the Australian clinical setting. J Clin Microbiol. 2016. A database was prepared for the identification of Aspergillus, Scedosporium , and Fusarium species using MALDI-TOF MS by combining in-house database with Bruker Fungal library, thereby improving the species identification significantly.
Roemer T, Krysan DJ. Antifungal drug development: challenges, unmet clinical needs, and new approaches. Cold Spring Harb Perspect Med. 2014;4
Kontoyiannis DP, Marr KA, Park BJ, Alexander BD, Anaissie EJ, Walsh TJ, et al. Prospective surveillance for invasive fungal infections in hematopoietic stem cell transplant recipients, 2001–2006: overview of the Transplant-Associated Infection Surveillance Network (TRANSNET) Database. Clin Infect Dis. 2010;50:1091–100.
Peterson SW. Phylogenetic analysis of Aspergillus species using DNA sequences from four loci. Mycologia. 2008;100:205–26.
Balajee SA, Baddley JW, Peterson SW, Nickle D, Varga J, Boey A, et al. Aspergillus alabamensis, a new clinically relevant species in the section Terrei. Eukaryot Cell. 2009;8:713–22.
Balajee SA, Kano R, Baddley JW, Moser SA, Marr KA, Alexander BD, et al. Molecular identification of Aspergillus species collected for the Transplant-Associated Infection Surveillance Network. J Clin Microbiol. 2009;47:3138–41.
Alastruey-Izquierdo A, Mellado E, Peláez T, Pemán J, Zapico S, Alvarez M, et al. Population-based survey of filamentous fungi and antifungal resistance in Spain (FILPOP Study). Antimicrob Agents Chemother. 2013;57:3380–7.
Alcazar-Fuoli L, Mellado E, Alastruey-Izquierdo A, Cuenca-Estrella M, Rodriguez-Tudela JL. Aspergillus section Fumigati: antifungal susceptibility patterns and sequence-based identification. Antimicrob Agents Chemother. 2008;52:1244–51.
Alastruey-Izquierdo A, Alcazar-Fuoli L, Cuenca-Estrella M. Antifungal susceptibility profile of cryptic species of Aspergillus. Mycopathologia. 2014;178:427–33.
Denning DW, Cadranel J, Beigelman-Aubry C, Ader F, Chakrabarti A, Blot S, et al. Chronic pulmonary aspergillosis: rationale and clinical guidelines for diagnosis and management. Eur Respir J. 2016;47:45–68.
Patterson TF, Thompson GR 3rd, Denning DW, Fishman JA, Hadley S, Herbrecht R, et al. Practice guidelines for the diagnosis and management of aspergillosis: 2016 update by the Infectious Diseases Society of America. Clin Infect Dis. 2016.
Maertens JA, Raad II, Marr KA, Patterson TF, Kontoyiannis DP, Cornely OA, et al. Isavuconazole versus voriconazole for primary treatment of invasive mould disease caused by Aspergillus and other filamentous fungi (SECURE): a phase 3, randomised-controlled, non-inferiority trial. Lancet. 2016;387:760–9.
Arendrup MC, Mavridou E, Mortensen KL, Snelders E, Frimodt-Møller N, Khan H, et al. Development of azole resistance in Aspergillus fumigatus during azole therapy associated with change in virulence. PLoS One. 2010;5:e10080.
Denning DW, Venkateswarlu K, Oakley KL, Anderson MJ, Manning NJ, Stevens DA, et al. Itraconazole resistance in Aspergillus fumigatus. Antimicrob Agents Chemother. 1997;41:1364–8.
Snelders E, van der Lee HA, Kuijpers J, Rijs AJ, Varga J, Samson RA, et al. Emergence of azole resistance in Aspergillus fumigatus and spread of a single resistance mechanism. PLoS Med. 2008;5:e219. Investigated the prevalence and spread of azole resistance in A. fumigatus isolates collected over a period of 14 years.
Bueid A, Howard SJ, Moore CB, Richardson MD, Harrison E, Bowyer P, et al. Azole antifungal resistance in Aspergillus fumigatus: 2008 and 2009. J Antimicrob Chemother. 2010;65:2116–8.
Bader O, Tünnermann J, Dudakova A, Tangwattanachuleeporn M, Weig M, Groß U. Environmental isolates of azole-resistant Aspergillus fumigatus in Germany. Antimicrob Agents Chemother. 2015;59:4356–9. The article describes that 12 % of the soil samples were positive for resistant A. fumigatus and harbored TR 34 /L98H and TR 46 /Y121F/T289A alleles, dispersed along a corridor across northern Germany.
Sharma C, Hagen F, Moroti R, Meis JF, Chowdhary A. Triazole-resistant Aspergillus fumigatus harbouring G54 mutation: is it de novo or environmentally acquired? J Global Antimicrob Resist. 2015;3:69–73. The authors describe the genetic heterogeneity of resistant A. fumigatus strains harboring the G54E mutation in the environment of India, Romania, and Tanzania anticipating that long-term exposure of A. fumigatus to fungicides may induce selection of G54 mutants in the environment.
Verweij PE, Chowdhary A, Melchers WJ, Meis JF. Azole resistance in Aspergillus fumigatus: can we retain the clinical use of mold-active antifungal azoles? Clin Infect Dis. 2016;62:362–8.
Chowdhary A, Sharma C, Hagen F, Meis JF. Exploring azole antifungal drug resistance in Aspergillus fumigatus with special reference to resistance mechanisms. Future Microbiol. 2014;9:697–711.
Snelders E, Karawajczyk A, Schaftenaar G, Verweij PE, Melchers WJ. Azole resistance profile of amino acid changes in Aspergillus fumigatus CYP51A based on protein homology modeling. Antimicrob Agents Chemother. 2010;54:2425–30. The authors gave an insight into the mutations in the cyp51A gene and correlated the mutations with the phenotype regarding their susceptibility to azole compounds.
Arabatzis M, Kambouris M, Kyprianou M, Chrysaki A, Foustoukou M, Kanellopoulou M, et al. Polyphasic identification and susceptibility to seven antifungals of 102 Aspergillus isolates recovered from immunocompromised hosts in Greece. Antimicrob Agents Chemother. 2011;55:3025–30.
Masih A, Singh PK, Kathuria K, Agarwal K, Meis JF, Chowdhary A. Identification by molecular methods and Matrix-assistedlaser desorption ionization–time of flight mass spectrometry and antifungal susceptibility profiles of clinically significant rare Aspergillus species in a referral chest hospital in Delhi, India. J Clin Microbiol. 2016, pii: JCM.00962-16.
Arendrup MC, Cuenca-Estrella M, Lass-Flörl C, Hope WW. Breakpoints for antifungal agents: an update from EUCAST focusing on echinocandins against Candida spp. and triazoles against Aspergillus spp. Drug Resist Updat. 2013;16:81–95.
Espinel-Ingroff A, Fothergill A, Fuller J, Johnson E, Pelaez T, Turnidge J. Wild-type MIC distributions and epidemiological cutoff values for caspofungin and Aspergillus spp. for the CLSI broth microdilution method (M38-A2 document). Antimicrob Agents Chemother. 2011;55:2855–9.
Espinel-Ingroff A, Chowdhary A, Gonzalez GM, Lass-Flörl C, Martin-Mazuelos E, Meis J, et al. Multicenter study of isavuconazole MIC distributions and epidemiological cutoff values for Aspergillus spp. for the CLSIM38-A2 broth microdilution method. Antimicrob Agents Chemother. 2013;57:3823–8.
Howard SJ, Lass-Flörl C, Cuenca-Estrella M, Gomez-Lopez A, Arendrup MC. Determination of isavuconazole susceptibility of Aspergillus and Candida species by the EUCAST method. Antimicrob Agents Chemother. 2013;57:5426–31.
Verweij PE, Ananda-Rajah M, Andes D, Arendrup MC, Brüggemann RJ, Chowdhary A, et al. International expert opinion on the management of infection caused by azole-resistant Aspergillus fumigatus. Drug Resist. 2015;21-22:30–40.
Van der Linden JWM, Arendrup MC, van der Lee HAL, Verweij PE. Azole containing agar plates as a screening tool for azole resistance of Aspergillus fumigatus. Mycoses. 2009;52 Suppl 1:19.
Denning DW, Park S, Lass-Florl C, Fraczek MG, Kirwan M, Gore R, et al. High-frequency triazole resistance found in nonculturable Aspergillus fumigatus from lungs of patients with chronic fungal disease. Clin Infect Dis. 2011;52:1123–9.
Zhao Y, Stensvold CR, Perlin DS, Arendrup MC. Azole resistance in Aspergillus fumigatus from bronchoalveolar lavage fluid samples of patients with chronic diseases. J Antimicrob Chemother. 2013;68:1497–504.
Chong GL, van de Sande WW, Dingemans GJ, Gaajetaan GR, Vonk AG, Hayette MP, et al. Validation of a new Aspergillus real-time PCR assay for direct detection of Aspergillus and azole resistance of Aspergillus fumigatus on bronchoalveolar lavage fluid. J Clin Microbiol. 2015;52:868–74.
Snelders E, Karawajczyk A, Verhoeven RJ, Venselaar H, Schaftenaar G, Verweij PE, et al. The structure-function relationship of the Aspergillus fumigatus cyp51A L98H conversion by site-directed mutagenesis: the mechanism of L98H azole resistance. Fungal Genet Biol. 2011;48:1062–70.
Mellado E, Garcia-Effron G, Alcazar-Fuoli L, Cuenca-Estrella M, Rodriguez-Tudela JL. Substitutions at methionine 220 in the 14alpha-sterol demethylase (Cyp51A) of Aspergillus fumigatus are responsible for resistance in vitro to azole antifungal drugs. Antimicrob Agents Chemother. 2004;48:2747–50.
Mellado E, Garcia-Effron G, Alcázar-fouli L, Melchers WJ, Verweij PE, Cuenca-Estrella M, et al. A new Aspergillus fumigatus resistance mechanism conferring in vitro cross-resistance to azole antifungals involves a combination of cyp51A alterations. Antimicrob Agents Chemother. 2007;51:1897–904.
van der Linden JW, Camps SM, Kampinga GA, Arends JP, Debets-Ossenkopp YJ, Haas PJ, et al. Aspergillosis due to voriconazole highly resistant Aspergillus fumigatus and recovery of genetically related resistant isolates from domiciles. Clin Infect Dis. 2013;57:513–20. The article describes the emergence of a new environmental resistance mechanism in A. fumigatus isolates from patients and their environment.
Camps SM, Dutilh BE, Arendrup MC, Rijs AJ, Snelders E, Huynen MA, et al. Discovery of a HapE mutation that causes azole resistance in Aspergillus fumigatus through whole genome sequencing and sexual crossing. PLoS One. 2012;7:e50034. The article reports a new non-cyp51A-mediated resistance mechanism using whole-genome sequencing in the A. fumigatus.
Albarrag AM, Anderson MJ, Howard SJ, Robson GD, Warn PA, Sanglard D, et al. Interrogation of related clinical pan-azole-resistant Aspergillus fumigatus strains: G138C, Y431C, and G434C single nucleotide polymorphisms in cyp51A, upregulation of cyp51A, and integration and activation of transposon Atf1 in the cyp51A promoter. Antimicrob Agents Chemother. 2011;55:5113–21.
Howard SJ, Harrison E, Bowyer P, Varga J, Denning DW. Cryptic species and azole resistance in the Aspergillus niger complex. Antimicrob Agents Chemother. 2011;55:4802–9.
Tortorano AM, Prigitano A, Esposto MC, Arsic Arsenijevic V, Kolarovic J, Ivanovic D, et al. European Confederation of Medical Mycology (ECMM) epidemiological survey on invasive infections due to Fusarium species in Europe. Eur J Clin Microbiol Infect Dis. 2014;33:1623–30.
Al-Hatmi AM, Van Den Ende AH, Stielow JB, Van Diepeningen AD, Seifert KA, Mc Cormick W, et al. Evaluation of two novel barcodes for species recognition of opportunistic pathogens in Fusarium. Fungal Biol. 2016;120:231–45.
Guarro J. Fusariosis, a complex infection caused by a high diversity of fungal species refractory to treatment. Eur J Clin Microbiol Infect Dis. 2013;32:1491–500.
Muhammed M, Anagnostou T, Desalermos A, Kourkoumpetis TK, Carneiro HA, Glavis-Bloom J, et al. Fusarium infection: report of 26 cases and review of 97 cases from the literature. Medicine (Baltimore). 2013;92:305–16.
Scheel CM, Hurst SF, Barreiros G, Akiti T, Nucci M, Balajee SA. Molecular analysis of Fusarium isolates recovered from a cluster of invasive mold infections in a Brazilian hospital. BMC Infect Dis. 2013;13:49.
van Diepeningen AD, Al-Hatmi AMS, Brankovics B, de Hoog GS. Taxonomy and clinical spectra of Fusarium species: where do we stand in 2014? Curr Clin Micro Rpt. 2014;1:10–8.
Garnica M, da Cunha MO, Portugal R, Maiolino A, Colombo AL, Nucci M. Risk factors for invasive fusariosis in patients with acute myeloid leukemia and in hematopoietic cell transplant recipients. Clin Infect Dis. 2015;60:875–80.
Vazquez JA, Miceli MH, Alangade G. Invasive fungal infections in transplant recipients. Ther Adv Infect Dis. 2013;3:85–105.
Stempel JM, Hammond SP, Sutton DA, Weiser LM, Marty FM. Invasive fusariosis in the voriconazole era: single-center 13-year experience. Open Forum Infect Dis. 2015;2:ofv099.
Al-Hatmi AM, van Diepeningen AD, Curfs-Breuker I, de Hoog GS, Meis JF. Specific antifungal susceptibility profiles of opportunists in the Fusarium fujikuroi complex. J Antimicrob Chemother. 2015;70:1068–71.
Espinel-Ingroff A, Colombo AL, Cordoba S, Dufresne PJ, Fuller J, Ghannoum M, et al. International evaluation of MIC distributions and epidemiological cutoff value (ECV) definitions for Fusarium species identified by molecular methods for the CLSI broth microdilution method. Antimicrob Agents Chemother. 2015;60:1079–84.
Abuhelwa AY, Foster DJR, Mudge S, Hayes D, Upton RN. Population pharmacokinetic modelling of itraconazole and hydroxyl-itraconazole for oral SUBA™-itraconazole and Sporanox® capsule formulations in healthy subjects in fed and fasted states. Antimicrob Agents Chemother. 2015;59:5681–96.
Fan J, Urban M, Parker JE, Brewer HC, Kelly SL, Hammond-Kosack KE, et al. Characterization of the sterol 14α-demethylases of Fusarium graminearum identifies a novel genus specific CYP51 function. New Phytol. 2013;198:821–35.
Harun A, Gilgado F, Chen SC, Meyer W. Abundance of Pseudallescheria/Scedosporium species in the Australian urban environment suggests a possible source for scedosporiosis including the colonization of airways in cystic fibrosis. Med Mycol. 2010;48:S70–6.
Subedi S, Chen SCA. Epidemiology of scedosporiosis. Curr Fungal Infect Rep. 2015;9:275–84.
Gilgado F, Cano J, Gene J, Sutton DA, Guarro J. Molecular and phenotypic data supporting distinct species statuses for Scedosporium apiospermum and Pseudallescheria boydii and the proposed new species Scedosporium dehoogii. J Clin Microbiol. 2008;46:766–71.
Troke P, Aguirrebengoa K, Arteaga C, Ellis D, Heath CH, Lutsar I, et al. Treatment of scedosporiosis with voriconazole: clinical experience with 107 patients. Antimicrob Agents Chemother. 2008;52:1743–50.
Heath CH, Slavin MA, Sorrell TC, Handke R, Harun A, Phillips M, et al. Population-based surveillance for scedosporiosis in Australia: epidemiology, disease manifestations and emergence of Scedosporium aurantiacum infection. Clin Microbiol Infect Off Publ Eur Soc Clin Microbiol Infect Dis. 2009;15:689–93.
Rodriguez-Tudela JL, Berenguer J, Guarro J, Kantarcioglu AS, Horre R, de Hoog GS, et al. Epidemiology and outcome of Scedosporium prolificans infection, a review of 162 cases. Med Mycol. 2009;47:359–70.
Ramsperger M, Duan S, Sorrell TC, Meyer W, Chen SC-A. The genus Scedosporium and Pseudallescheria: current challenges in laboratory diagnosis. Curr Clin Microbiol Rep. 2014;1:27–36. The review abridges the methods currently used to enable an informed choice of detection and/or identification techniques in the clinical mycology laboratory.
Lackner M, de Hoog GS, Verweij PE, Najafzadeh MJ, Curfs-Breuker I, Klaassen CH, et al. Species-specific antifungal susceptibility patterns of Scedosporium and Pseudallescheria species. Antimicrob Agents Chemother. 2012;56:2635–42. The article reports species-specific susceptibility patterns of Pseudallescheria boydii and P. apiosperma and found only voriconazole exhibited low MIC values for P. apiosperma and P. boydii.
Gosbell IB, Toumasatos V, Yong J, Kuo RS, Ellis DH, Perrie RC. Cure of orthopaedic infection with Scedosporium prolificans, using voriconazole plus terbinafine, without the need for radical surgery. Mycoses. 2003;46:233–6.
Machouart M, Garcia-Hermoso D, Rivier A, Hassouni N, Catherinot E, Salmon A, et al. Emergence of disseminated infections due to Geosmithia argillacea in patients with chronic granulomatous disease receiving long-term azole antifungal prophylaxis. J Clin Microbiol. 2011;49:1681–3.
Valentin T, Neumeister P, Pichler M, Rohn A, Koidl C, Haas D, et al. Disseminated Geosmithia argillacea infection in a patient with gastrointestinal GvHD. Bone Marrow Transplant. 2012;47:734–6.
Houbraken J, Spierenburg H, Frisvad JC. Rasamsonia, a new genus comprising thermotolerant and thermophilic Talaromyces and Geosmithia species. Antonie Van Leeuwenhoek. 2012;101:403–21.
Houbraken J, Verweij PE, Rijs AJ, Borman AM, Samson RA. Identification of Paecilomyces variotii in clinical samples and settings. J Clin Microbiol. 2010;48:2754–61.
Cohen-Abbo A, Edwards KM. Multifocal osteomyelitis caused by Paecilomyces variotii in a patient with chronic granulomatous disease. Infection. 1995;23:55–7.
Eloy P, Bertrand B, Rombeaux P, Delos M, Trigaux JP. Mycotic sinusitis. Acta Otorhinolaryngol Belg. 1997;51:339–52.
Salle VE, Lecuyer T, Chouaki F, Lescure X, Smail A, Vaidie A, et al. Paecilomyces variotii fungemia in a patient with multiple myeloma: case report and literature review. J Infect. 2005;51:e93–5. The authors report the first case of P. variotii fungemia along with review of literature suggesting substantial morbidity due to this fungus.
De Hoog GS, Guarro J, Gené J, Figueras MJ. Atlas of clinical fungi. 2nd ed. Utrecht, Netherlands/ University Rovira i Virgili, Reus, Spain: Centraalbureau voor Schimmelcultures; 2000.
Summerbell RC. Aspergillus, Fusarium, Sporothrix, Piedraia, and their relatives. In: Howard DH, editor. Pathogenic fungi in humans and animals. 2nd ed. New York: Marcel Dekker; 2003. p. 237–498.
Perdomo H, Sutton DA, García D, Fothergill AW, Cano J, Gené J, et al. Spectrum of clinically relevant Acremonium species in the United States. J Clin Microbiol. 2011;49:243–56. The study identified a large number of clinical isolates belonging to Acremonium species morphologically and confirmed the identifications by internal transcribed spacer region of the rRNA gene sequencing.
Pfaller M, Boyken L, Hollis R, Kroeger J, Messer S, Tendolkar S, et al. Use of epidemiological cutoff values to examine 9-year trends in susceptibility of Aspergillus species to the triazoles. J Clin Microbiol. 2011;49:586–90.
Revankar SG, Sutton DA. Melanized fungi in human disease. Clin Microbiol Rev. 2010;23:884–928.
Chowdhary A, Perfect J, de Hoog GS. Black molds and melanized yeasts pathogenic to humans. Cold Spring Harb Perspect Med. 2014;5:a019570. A comprehensive review describing melanized fungi involved in human infection.
Chowdhary A, Meis JF, Guarro J, de Hoog GS, Kathuria S, Arendrup MC, et al. ESCMID and ECMM joint clinical guidelines for the diagnosis and management of systemic phaeohyphomycosis: diseases caused by black fungi. Clin Microbiol Infect. 2014;20 Suppl 3:47–75.
Sitterlé E, Giraud S, Leto J, Bouchara JP, Rougeron A, Morio F, et al. Matrix-assisted laser desorption ionization-time of flight mass spectrometry for fast and accurate identification of Pseudallescheria/Scedosporium species. Clin Microbiol Infect. 2014;20:929–35. The authors investigated the potential of MALDI-TOF MS to discriminate Pseudallescheria/Scedosporium species that cannot be currently identified by morphological examination in the clinical setting by building a reference database library.
Escribano P, Peláez T, Muñoz P, Bouza E, Guinea J. Is azole resistance in Aspergillus fumigatus a problem in Spain? Antimicrob Agents Chemother. 2013;57:2815–20.
Peláez T, Alvarez-Pérez S, Mellado E, Serrano D, Valerio M, Blanco JL, et al. Invasive aspergillosis caused by cryptic Aspergillus species: a report of two consecutive episodes in a patient with leukaemia. J Med Microbiol. 2013;62:474–8.
Negri CE, Gonçalves SS, Xafranski H, Bergamasco MD, Aquino VR, Castro PT, et al. Cryptic and rare Aspergillus species in Brazil: prevalence in clinical samples and in vitro susceptibility to triazoles. J Clin Microbiol. 2014;52:3633–40.
Balajee SA, Gribskov J, Brandt M, Ito J, Fothergill A, Marr KA. Mistaken identity: Neosartorya pseudofischeri and its anamorph masquerading as Aspergillus fumigatus. J Clin Microbiol. 2005;43:5996–9.
Vinh DC, Shea YR, Sugui JA, Parrilla-Castellar ER, Freeman AF, Campbell JW, et al. Invasive aspergillosis due to Neosartorya udagawae. Clin Infect Dis. 2009;49:102–11.
Gonçalves SS, Stchigel AM, Cano J, Guarro J, Colombo AL. In vitro antifungal susceptibility of clinically relevant species belonging to Aspergillus section Flavi. Antimicrob Agents Chemother. 2013;57:1944–7.
Varga J, Houbraken J, Van Der Lee HA, Verweij PE, Samson RA. Aspergillus calidoustus sp. nov., causative agent of human infections previously assigned to Aspergillus ustus. Eukaryot Cell. 2008;7:630–8.
Panackal AA, Imhof A, Hanley EW, Marr KA. Aspergillus ustus infections among transplant recipients. Emerg Infect Dis. 2006;12:403–8.
Arendrup MC, Jensen RH, Grif K, Skov M, Pressler T, Johansen HK, et al. In vivo emergence of Aspergillus terreus with reduced azole susceptibility and a Cyp51a M217I alteration. J Infect Dis. 2012;206:981–5.
Wang H, Xiao M, Kong F, Chen S, Dou HT, Sorrell T, et al. Accurate and practical identification of 20 Fusarium species by seven-locus sequence analysis and reverse line blot hybridization, and an in vitro antifungal susceptibility study. J Clin Microbiol. 2011;49:1890–8.
Dalyan Cilo B, Al-Hatmi AM, Seyedmousavi S, Rijs AJ, Verweij PE, Ener B, et al. Emergence of fusarioses in a university hospital in Turkey during a 20-year period. Eur J Clin Microbiol Infect Dis. 2015;34:1683–91.
Ricna D, Lengerova M, Palackova M, Hadrabova M, Kocmanova I, Weinbergerova B, et al. Disseminated fusariosis by Fusarium proliferatum in a patient with aplastic anaemia receiving primary posaconazole prophylaxis—case report and review of the literature. Mycoses. 2016;59:48–55.
Nishimori M, Takahashi T, Suzuki E, Kodaka T, Hiramoto N, Itoh K, et al. Fatal fungemia with Scedosporium prolificans in a patient with acute myeloid leukemia. Med Mycol J. 2014;55:E63–70.
Lackner M, Rezusta A, Villuendas MC, Palacian MP, Meis JF, Klaassen CH. Infection and colonisation due to Scedosporium in Northern Spain. An in vitro antifungal susceptibility and molecular epidemiology study of 60 isolates. Mycoses. 2011;54 Suppl 3:12–21.
Homa M, Galgóczy L, Tóth E, Tóth L, Papp T, Chandrasekaran M, et al. In vitro antifungal activity of antipsychotic drugs and their combinations with conventional antifungals against Scedosporium and Pseudallescheria isolates. Med Mycol. 2015;5:890–5.
Bernhardt A, Seibold M, Rickerts V, Tintelnot K. Cluster analysis of Scedosporium boydii infections in a single hospital. Int J Med Microbiol. 2015;305:724–8.
Júnior MC, de Moraes AA, Silva HM, Costa CR, Silva MR. Acremonium kiliense: case report and review of published studies. Mycopathologia. 2013;176:417–21.
Herbrecht R, Letscher-Bru V, Fohrer C, Campos F, Natarajan-Ame S, Zamfir A, et al. Acremonium strictum pulmonary infection in a leukemic patient successfully treated with posaconazole after failure of amphotericin B. Eur J Clin Microbiol Infect Dis. 2002;21:814–7.
Houbraken J, Giraud S, Meijer M, Bertout S, Frisvad JC, Meis JF, et al. Taxonomy and antifungal susceptibility of clinically important Rasamsonia species. J Clin Microbiol. 2013;51:22–30.
Giraud S, Pihet M, Razafimandimby B, Carrère J, Degand N, Mely L, et al. Geosmithia argillacea: an emerging pathogen in patients with cystic fibrosis. J Clin Microbiol. 2010;48:2381–6.
De Ravin SS, Challipalli M, Anderson V, Shea YR, Marciano B, Hilligoss D, et al. Geosmithia argillacea: an emerging cause of invasive mycosis in human chronic granulomatous disease. Clin Infect Dis. 2011;52:e136–43.
da Cunha KC, Sutton DA, Fothergill AW, Gené J, Cano J, Madrid H, et al. In vitro antifungal susceptibility and molecular identity of 99 clinical isolates of the opportunistic fungal genus Curvularia. Diagn Microbiol Infect Dis. 2013;76:168–74.
Giraldo A, Sutton DA, Samerpitak K, de Hoog GS, Wiederhold NP, Guarro J, et al. Occurrence of Ochroconis and Verruconis species in clinical specimens from the United States. J Clin Microbiol. 2014;52:4189–201.
Badali H, Chander J, Gulati N, Attri A, Chopra R, Najafzadeh MJ, et al. Subcutaneous phaeohyphomycotic cyst caused by Pyrenochaeta romeroi. Med Mycol. 2010;48:763–8.
Cerar D, Malallah YM, Howard SJ, Bowyer P, Denning DW. Isolation, identification and susceptibility of Pyrenochaeta romeroi in a case of eumycetoma of the foot in the UK. Int J Antimicrob Agents. 2009;34:617–8.
Badali H, Yazdanparast SA, Bonifaz A, Mousavi B, de Hoog GS, Klaassen CH, et al. Veronaea botryosa: molecular identification with amplified fragment length polymorphism (AFLP) and in vitro antifungal susceptibility. Mycopathologia. 2013;175:505–13.
Sang H, Zheng XE, Kong QT, Zhou WQ, He W, Lv GX, et al. A rare complication of ear piercing: a case of subcutaneous phaeohyphomycosis caused by Veronaea botryosa in China. Med Mycol. 2011;49:296–302.
Author information
Authors and Affiliations
Corresponding author
Ethics declarations
Conflict of Interest
Anuradha Chowdhary, Aradhana Masih, Cheshta Sharma declare that they have no conflict of interest. Cheshta Sharma was supported by a research grant from University Grants Commission Research Fellowship, India (F.2-15/2003 SA-I).
Human and Animal Rights and Informed Consent
This article does not contain any studies with human or animal subjects performed by any of the authors.
Additional information
This article is part of the Topical Collection on Advances in Diagnosis of Invasive Fungal Infections
Rights and permissions
About this article
Cite this article
Chowdhary, A., Masih, A. & Sharma, C. Azole Resistance in Moulds—Approach to Detection in a Clinical Laboratory. Curr Fungal Infect Rep 10, 96–106 (2016). https://doi.org/10.1007/s12281-016-0265-2
Published:
Issue Date:
DOI: https://doi.org/10.1007/s12281-016-0265-2