Abstract
Tidal forcing is an important regulator of short-term variation in water properties and phytoplankton dynamics in estuaries. Tidal interactions with regulated freshwater discharge into estuaries that are affected by engineered structures such as sea embankments remain poorly understood. We examined tidal variations in the macrotidal (3–6 m) Yeongsan River estuary of South Korea, which receives regulated freshwater from a sea embankment. Semidiurnal variations in water properties and the phytoplankton community (size and taxonomic groups) were investigated fortnightly during four monitoring campaigns at a fixed station over daily cycles at neap and spring tides during both the dry (April–May 2012) and wet (August 2012) seasons. We calculated correlations and performed multivariate statistical analyses to identify the major factors affecting tidal variations. We found no consistent or predictable variations in water quality or the phytoplankton community over the fortnightly or semidiurnal tidal cycles. These parameters were more likely dependent on the extent of recovery from artificial freshwater discharge than on the tidal forcing, which is supported by the absence of any significant correlations between tidal height and water properties or phytoplankton features. Multivariate statistical analysis showed that tidal variation in the taxonomic community may be associated with freshwater discharge and was explained principally by water temperature and salinity, rather than tidal height. Diel tidal effects were in play only at a neap tide in the wet season after no freshwater had been discharged for 17 days, which is rare during the wet season. These findings imply that episodic anthropogenic freshwater discharge events disturb predictable macrotidal effects on water column processes, in turn enhancing the ecological complexity of estuaries such as the Yeongsan River estuary that have previously been altered by engineered structures.
Similar content being viewed by others
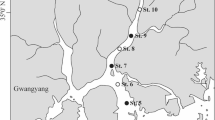
Explore related subjects
Discover the latest articles, news and stories from top researchers in related subjects.Avoid common mistakes on your manuscript.
Introduction
As marine environments where freshwater and saltwater interact, estuaries are valued as highly productive ecosystems (Costanza et al. 1997, 2014; Longhurst et al. 1995). The biological benefits of estuaries include their functions as critical nursery and recruitment habitats for numerous living resources (McLusky and Elliott 2004; Dauvin 2008). Estuaries are inherently complex marine systems, because their biotic and abiotic properties vary spatially and temporally, in turn regulating the dynamics of organisms. Phytoplankton communities, formed of primary producers that play important roles in the carbon (C) balance, are controlled by variations in the properties of estuaries (Cloern and Jassby 2012). Exploring the size and taxonomic structures of phytoplankton is especially important to improve our understanding of estuarine ecosystems. This is because the structures can determine the composition of the food web in aquatic systems (Froneman et al. 2004; Vargas and Gonzalez 2004). Fluctuations in phytoplankton size and taxonomic structure can be used as indicators to detect variation in the chemical and physical properties of habitats generated by environmental changes. Many studies have reported that phytoplankton communities respond to environmental disturbances; these responses are subject to phytoplankton cell size and taxonomic structure, especially when the disturbance is anthropogenic (Nayar et al. 2005; Alvarez-Gongora and Herrera-Silveira 2006; Bode et al. 2017).
Anthropogenic pressure is relatively high in estuaries, and human coastal settlements are expanding (Kennish 2002). Estuaries lie at the interface between land and sea and receive terrestrial pollutants via river discharge. River discharge is also a major source of nutrients that support primary production, especially phytoplankton in productive systems (Adolf et al. 2006; Smith 2006). However, excessive nutrient supply causes eutrophication and triggers harmful algal blooms (HABs) resulting in water quality problems such as hypoxia (Diaz and Rosenberg 2008) and the depletion of dissolved oxygen and release of toxins, which can cause fish kills (Landsberg 2002; Harrison et al. 2017). River discharge varies in estuaries at seasonal and inter-annual time scales; these variations generally produce low-frequency fluctuations in phytoplankton dynamics (Wu et al. 2016; Bharathi et al. 2018)
In addition to river discharge, tidal forcing (e.g., tidal mixing) produces high-frequency (fortnightly neap and spring) variations in estuarine phytoplankton communities via stratification and mixing (Cloern 1991; Angara et al. 2013; Zhou et al. 2016). Phytoplankton communities are also influenced by diel rhythm (high and low tides) in estuaries (Domingues et al. 2010; Olofsson et al. 2017). Phytoplankton biomass measured at a fixed location can vary significantly (3- to 5-fold) throughout the diel tidal cycle in estuaries (Cloern et al. 1989; Litaker et al. 1993).
Many estuaries in coastal areas of South Korea have been altered by the construction of sea embankments, which divide estuarine systems into freshwater and saltwater zones. Freshwater is discharged from sluice gates in the sea embankments, dramatically changing the water properties of these saltwater zones (Yang et al. 2014). The responses of phytoplankton to freshwater discharge in altered estuarine systems are short-lived (days) and episodic (Sin and Jeong 2015), in contrast to natural estuaries, which exhibit low-frequency changes. Few studies of diel or fortnightly variations in phytoplankton communities have been conducted, especially in altered estuaries. However, a recent report found that the physical properties of an altered estuary with macrotidal amplitude (6–9 m), including chl a and taxonomic composition, fluctuated according to the semidiurnal cycle (Sin et al. 2015a). Although fortnightly spring-neap tides were not considered, these phytoplankton responses were predictable based on the semidiurnal tidal cycle and their spatial distribution, which was affected by regulated freshwater discharge. Thus, although it is clear that altered estuaries are subject to freshwater events and tidal forcing, the diel and fortnightly rhythms of phytoplankton communities, including water properties and direct effects of the anthropogenic freshwater on their tidal rhythms, remain poorly understood.
The Yeongsan River estuary (Fig. 1) is located in a temperate region (36°–37° N, 126°–127° E) on the west coast of South Korea. The estuary is affected by a monsoon climate with very wet summers and dry conditions during the remaining seasons. The estuary experiences pressure from anthropogenic disturbances due to the construction of sea embankments in its coastal regions (Lee et al. 2009; Kim and Chang 2017). Freshwater from associated reservoirs is discharged through sluice gates built into the sea embankment; this discharge results in substantial changes in the water properties and phytoplankton community of the estuary (Sin et al. 2013; Sin and Jeong 2015). However, the estuary remains a semi-enclosed water body when no freshwater is introduced and tidal forcing, dominated by ebb tides with mean neap and spring tidal ranges of 3–6 m (Kang and Jun 2003; Byun et al. 2004). The tidal range increased by approximately 1 m after construction of the embankment (Kang 1999). In this context, tidal forcing may be a major regulator affecting the phytoplankton community and water properties. Tidal variations in the phytoplankton community have not yet been reported for this estuary.
Locations of monitoring stations along the axis of the Yeongsan River estuary. Station T was selected for semidiurnal monitoring, and samples were collected at Stations 1–3 to examine spatial variation before semidiurnal sampling during the neap-spring fortnightly tidal cycle during the dry and wet seasons. The aerial images were obtained from Daum (www.daum.net)
We posed the following research questions: (1) Is the variation in phytoplankton and water properties predictable over the tidal cycle in the estuary below the embankment? and (2) How do anthropogenic freshwater inputs interact with tidal forcing in terms of controlling phytoplankton dynamics and water quality? We measured diel and fortnightly variations in phytoplankton size and taxonomic structure in a macrotidal estuary as well as water properties during both the dry and wet seasons, before and after freshwater inputs. The results of this study will lead to a better understanding of the anthropogenic effects of regulated freshwater on semidiurnal and fortnightly phytoplankton dynamics and other properties of estuaries disturbed by engineered structures. A better understanding of anthropogenic effects on phytoplankton size and taxonomic structure is important to improve management of coastal estuarine ecosystems, the structure of which determines the estuarine food-web composition.
Methods
Study Site and Sampling Design
A sea embankment located 7 km from the mouth of the Yeongsan River estuary was built to reclaim tidal flat areas and provide agricultural water to extensive nearby paddy fields and physically transects the estuary into freshwater and saltwater zones (Fig. 1). The sea embankment (or sea dike) is a low concrete dam 19.5 m in height (H) and 4.45 km in length (L), fitted with sluice gates (height × length: 13.6 × 30.0 m). The Yeongsan River watershed covers 3470 km2, and the main channel of the river is 137 km in length. The freshwater reservoir approximately 24 km upstream from the sea embankment has a surface area of 34.6 km2 and storage capacity of 2.54 × 108 m3. Freshwater is discharged into the saltwater zone at low tide by opening sluice gates when the water level of the reservoir becomes high. Hypoxia (< 2 mg L−1) develops in the bottom water of the reservoir near the embankment during the warm season (Song et al. 2015). Tides in the estuary are semidiurnal with a macrotidal range of 3–6 m during fortnightly cycles.
Diel sampling and monitoring were conducted at Station T in the upper region of the saltwater zone in the Yeongsan River estuary (Fig. 1) every 1.5 h for two tidal cycles during neap and spring tides, respectively, during the dry and wet seasons (Table 1). The diel investigation was conducted on 28–29 April (neap tide [ND]) and 7–8 May (spring tide [SD]) during the dry season and on 12–13 August (neap tide [NW]) and 17–18 August 2012 (spring tide [SW]) during the wet season. We monitored continuously from high tide to high tide at neap tides and from low tide to low tide at spring tides over two semidiurnal tidal cycles; the monitoring periods were designated as E0 to E3 or E4 during ebbing and F0 to F3 or F4 during flooding over the first (S1) and second (S2) semidiurnal cycles, respectively. During the monitoring period, water samples were collected 0.5 m below the surface and 0.5 m from the bottom using a Niskin water sampler at water depths from 14.1 to 24.5 m. Samples and data were also collected from the three stations (Stations 1–3) to explore horizontal variation in water properties and phytoplankton size structure along the channel of the saltwater zone at neap tide (April 27 and August 11) before diel monitoring (Fig. 1). Primary productivity was estimated to examine the in situ growth of phytoplankton at the stations.
Measurement of Water Properties and Hydrology
Water temperature (°C), salinity (psu), turbidity (NTU), pH, and dissolved oxygen (DO, mg L−1) were measured using a YSI Model 6600 multiparameter probe. Water depth was estimated using a Hondex PS-7 digital sounder. Data for the freshwater discharge from the sluice gates of the sea embankment were provided by the Korean Agricultural Administration.
Determination of Phytoplankton Size, Taxonomic Composition, and Primary Productivity
Phytoplankton in the water samples were filtered (before the chl a measurements) through a 20-μm Nitex mesh (1–2 L) and 2-μm membrane filters (1 L) and classified into three size classes: micro (> 20 μm), nano (2–20 μm), and pico (< 2 μm). To determine chl a, 100 mL whole water, 200 mL 20-μm filtrate, and 300 mL 2-μm filtrate were filtered through Whatman 25-mm GF/F glass microfiber filters (0.7 μm nominal pore size). The filters were stored in dark test tubes prefilled with 8 mL of extraction solution (90% acetone and 10% distilled water). After storage for 24 h at 4 °C, the chl a was measured using a Turner Designs 10-AU fluorometer; chl a in each size fraction was estimated by consecutive subtraction of the < 2-μm and < 20-μm fractions from the chl a of the entire water sample.
To identify phytoplankton groups, samples were collected separately using 1-L bottles containing 5 mL of Lugol’s solution (final I2 concentration: 250 mg L−1). The samples were left undisturbed for at least 48 h to allow phytoplankton to settle. After settling, 800 mL of supernatant was removed from the bottle, and the remaining 200-mL sample was mixed. For quantification, a 1-mL concentrated sample was inserted into a Sedgewick-Rafter counting chamber (50 × 20 × 1 mm), and phytoplankton species were identified and cells, including cyanobacteria, counted using a Zeiss Axioskop 2 MAT microscope (× 200). Taxonomic compositions were determined by sorting the phytoplankton species into seven groups: Bacillariophyceae (diatoms), Chlorophyceae (green algae), Chrysophyceae, Cryptophyceae (cryptophytes), Cyanophyceae (cyanobacteria), Dinophyceae (dinoflagellates), and Euglenophyceae. Chrysophyceae and Euglenophyceae are denoted as “Others” in the results.
Vertical profiles of active fluorescence were determined by a Chelsea Technologies Group FASTtracka fast repetition rate fluorometer (FRRF) with dual light (L) and dark (D) chambers that used an in situ measurement protocol (Kolber et al. 1998). Data from the FRRF were processed by the FASTtracka post-processing software, which derives photosynthetic parameters from the induction curve. Because sampling depths in light- and dark-chamber measurements were different, all parameters gauged in the dark chamber were linearly interpolated according to the sampling depths of the light-chamber measurements. The FRRF-based photosynthetic rate (p) was estimated using the following equation (Smyth et al. 2004) and expressed in mg C m−3 h−1:
where 1.87 × 104 is a conversion factor (1020 m2 photons; 3600 s h−1; 6.02 × 1023 molecules mol−1; 12 g C mol−1 C; 892 g chl a mol−1). I is irradiance, Fv′/Fm′ is an index of the in situ operating efficiency of photosystem II, and σPSII is the average effective cross-section of the reaction centers. Corresponding values of chl a for FRRF measurement sampling depths were determined by nearest-neighbor interpolation of the discrete chl a measurements. Primary productivity within the euphotic zone was determined by depth integration. Phytoplankton doubling time was calculated to explore the relative importance of local phytoplankton growth and tidal forcing on its intratidal fluctuation, based on primary productivity measurements and the C:chl a ratio, i.e., 40 (Malone and Chervin 1979).
Statistical Analyses
Pearson’s correlation analysis was used to identify relationships among water properties and the phytoplankton community using PASW Statistics 22 software (SPSS). Simultaneous interactions among water properties were examined using a multivariate statistical tool (non-metric multidimensional scaling [nMDS]) in PRIMER ver. 6. Vectors of the water properties representing the direction of the greatest increase were superimposed on the nMDS results. The length of each vector indicates the magnitude of the correlation between the water properties and phytoplankton groups.
Results
Freshwater Discharge and Tidal and Water Properties
Freshwater was discharged through the sluice gates during April 24–26, 2012 (Table 1, Fig. 2) before ND (neap tide during the dry season) monitoring. Freshwater discharge was also recorded on May 2 and May 4 before SD (spring tide during the dry season) monitoring. No freshwater was discharged for 17 days before NW (neap tide during the wet season) monitoring. Freshwater was discharged daily before SW (spring tide during the wet season) monitoring and particularly during a period of 2 h 48 min during the middle of the monitoring period.
Tidal heights fluctuated with a range of − 0.53 to 5.17 m (Fig. 3) and water depths ranged from 14.1 to 24.5 m, following the tidal height pattern. Water temperature appeared to change in phase with tidal height except during SD monitoring, when it changed slightly out of phase with tidal height. The range of surface water temperatures during NW monitoring was narrow (± 1.2°C) compared with those of other monitoring periods. The temperature differences between the surface and bottom water were relatively high (1.5–6.8°C) during the entire monitoring period, indicating the development of strong thermal stratification of the water column during monitoring.
The pattern of salinity relative to tidal height was not clear during the monitoring period except during NW monitoring, when salinity varied out of phase with tidal height with a range of ± 1.0 psu (30.0–31.0 psu). The minimum salinity of the surface water (4.5 psu) was during SW monitoring while freshwater was being discharged (Fig. 3). Differences in surface and bottom salinity were relatively high (3.2–27.0 psu) and were consistent with those of temperature except during NW monitoring, when the minimum difference (< 1.9 psu) was observed. These results indicate the development of strong haline stratification of the water column, except during NW monitoring. Turbidity, DO, and pH did not display a clear relationship with tidal height; however, turbidity increased abruptly from 5.4 to 24.6 NTU, and DO decreased from 11.8 to 4.9 mg L−1, after freshwater was discharged during SW monitoring. pH exhibited a pattern (decreasing from 8.0 to 7.2) similar to that of DO (Fig. 3).
The surface water temperatures decreased downstream on April 27, whereas they increased downstream on August 11 (Table 2). The surface water salinity generally increased downstream. The DO values were lowest (5.9 mg L−1) at Station 1 and lower during the wet season than during the dry season.
Spatiotemporal Variation in Phytoplankton Parameters
Total chl a was generally out of phase with tidal height, with high concentrations at ebb or low tide except during the second semidiurnal cycles of NW and SW monitoring (Fig. 4). Extreme variation was observed during the first semidiurnal cycles of NW and SW monitoring, ranging from 6.40 to 117.36 μg L−1 (18.3-fold) and from 2.77 to 44.56 μg L−1 (16.1-fold), respectively. These results indicate that tidal variation in the total chl a level was greater during the wet season than the dry season. Concentrations remained low during the second semidiurnal cycle in the wet season, except for a peak at high tide on August 18 (SW). The relationship between micro-, nano-, and pico-sized chl a and tidal height was similar to that shown by total chl a. The low total chl a at neap tide on August 13 (NW) and its peak at spring tide on August 18 (SW) were attributed to a decrease and peak, respectively, in the chl a of micro-sized phytoplankton. This result was confirmed by the acute changes in the percent contribution by size classes (Fig. 4).
Semidiurnal variations in phytoplankton size structure, including chlorophyll a concentration (chl a, μg L−1) in all phytoplankton (total), and in micro-, nano-, and pico-sized phytoplankton; and percent (%) contributions by each size class to total chl a at neap and spring tides during the dry and wet seasons, respectively. S1 and S2: first and second semidiurnal tides, respectively
Phytoplankton taxonomic groups generally did not show a clear relationship with tidal height (Fig. 5). Diatom (Bacillariophyceae) abundance was high compared with those of the other groups, ranging from 741 to 1430 cells mL−1 during NW; cryptophyte (Cryptophyceae) abundance was also relatively high, ranging from 50 to 557 cells mL−1, and was slightly out of phase with tidal height during SD monitoring. Dinoflagellate (Dinophyceae) abundance increased rapidly from the beginning of the monitoring period and remained high compared with that of the other groups, reaching 1216 cells mL−1 at 16:38 (S1E2) during SW monitoring, whereas cyanobacteria (Cyanophyceae) abundance increased to 4533 cells mL−1 after dinoflagellates disappeared. The cyanobacteria peak observed at 02:34 (S2E0) during SW monitoring contributed greatly to an increase in micro-sized phytoplankton and total chl a (Fig. 5) because the cyanobacteria that peaked during the wet season had formed colonies.
Semidiurnal variations in abundance (cells mL−1) of phytoplankton groups including diatoms, green algae, cryptophytes, cyanobacteria, dinoflagellates, and others; and percent (%) contributions by each group to total phytoplankton abundance at neap and spring tides during the dry and wet seasons, respectively. S1 and S2: first and second semidiurnal tides, respectively
Total chl a and its concentration in nano- and pico-sized phytoplankton were highest in surface water at Station 2 on April 27, whereas total chl a (10.24 μg L−1) and concentrations in all size classes were highest at Station 2 on August 11 (Table 2). Phytoplankton primary productivity was highest (320 mg C m−2 day−1) downstream at Station 3 on April 27 and was highest (2635.6 mg C m−2 day−1) at Station 2 on August 11, 2012. Doubling time was longest (11.2 days) at Station 1 among the stations and decreased downstream on April 27, whereas it was longest at Station 2 (1.4 days) and shorter on August 11 than on April 27.
Relationships Between Water Properties and Phytoplankton Parameters
Tidal height was not correlated with salinity in the water column (Table 3). Temperature and turbidity were negatively correlated with salinity (both R < − 0.40, P < 0.05, n = 34). DO was negatively and significantly correlated with salinity during the dry season (R = − 0.73, P < 0.01, n = 34) but positively correlated during the wet season (R > 0.56, P < 0.01, n = 34). pH was positively correlated with salinity (R > 0.38, P < 0.05, n = 34). Total chl a and its concentration in micro-sized phytoplankton were positively correlated with salinity in the surface water during the wet season (R > 0.38, P < 0.05, n = 34). Pico-sized chl a was positively correlated with salinity in the surface water during the dry season (R = 0.37, P < 0.05, n = 34), whereas it was negatively correlated in the bottom water (R < − 0.52, P < 0.01, n = 34). Diatom abundance was significantly and positively correlated with salinity (R > 0.35, P < 0.05, n = 34).
Tidal height did not correlate with temperature, turbidity, DO, pH, or any phytoplankton parameter (data not shown). However, tidal height was significantly and negatively correlated with a surface water salinity of ± 1.0 psu (R = − 0.84, P < 0.01, n = 17) during NW monitoring when no freshwater had been discharged for 17 days. Turbidity was also positively correlated with tidal height (R = 0.43, P < 0.1, n = 17) during NW monitoring. Total and micro-sized chl a levels were significantly and negatively correlated with tidal height (R = − 0.84 and − 0.83, respectively, P < 0.01, n = 9) during the first semidiurnal cycle (S1) of NW monitoring, whereas the total chl a level was positively correlated with tidal height (R = 0.76, P < 0.05, n = 8) during the second semidiurnal cycle (S2).
nMDS analysis showed that the phytoplankton community was similar during SD monitoring when freshwater had been discharged 3 days prior, and during NW monitoring when water had been discharged 17 days previously (Fig. 6). The phytoplankton community changed abruptly during the second semidiurnal tide (S2) following the freshwater discharge during SW; the community was distinct from that recorded during other monitoring periods. The community varied by tidal stage during ND, when freshwater had been discharged 2 days prior. The vectors of water properties indicated that the variation was explained principally by changes in surface water turbidity and salinity. Temperature was mainly responsible for the difference in phytoplankton groups between the dry and wet seasons.
Non-metric multidimensional scaling (nMDS) analysis of phytoplankton abundances during monitoring performed at neap (ND, NW) and spring (SD, SW) tides during the dry and wet seasons; vectors indicate the strength and direction of the water properties, including surface water temperature (T), salinity (S), turbidity (Turb), pH, and tidal height (TH). Monitoring time is denoted by F0 at low tide to F3 or F4 and by E0 at high tide to E3 or E4 for the first (S1) and second (S2) semidiurnal tides
Discussion
Fortnightly Variation in Water Column Processes
In estuaries, stratification and destratification of the water column occur at neap and spring tides, respectively (Li and Zhong 2009). In this study, we did not observe fortnightly destratification because the thermal stratification was strong over the entire monitoring period. Strong haline stratification was also evident, except during NW monitoring after no freshwater had been discharged for a long time. When freshwater was discharged before or during monitoring of the spring tides (SD, SW), haline and thermal stratification were observed. Solar radiation may have affected the thermal stratification during NW monitoring. Coastal stability is affected by solar radiation, freshwater input, and tidal mixing (Garrett et al. 1978; Barcena et al. 2016). Therefore, in the Yeongsan River estuary, water column stability is affected by radiation and regulated by anthropogenic freshwater discharge through the sluice gates of the embankment more than by tidal mixing.
No consistent fortnightly variation was evident in the turbidity, DO, pH, or phytoplankton parameters. Surface water DO concentrations, pH, and phytoplankton biomass were higher at spring tide (SD) (associated with higher salinity) than at neap tide (ND) (associated with lower salinity) in the dry season, but higher at neap tide (NW) (associated with higher salinity) than at spring tide (SW) (associated with lower salinity) in the wet season. Cryptophyte levels were higher when the surface water salinity increased (SD) during the dry season, whereas diatom levels were higher when salinity increased (NW) during the wet season. These results imply that fortnightly variations in DO, pH, algal biomass, and the community may depend more on the extent of recovery from freshwater discharge impacts than on the strength of tidal mixing. A previous study found that freshwater inputs contributed to decreases in DO and chl a, especially for large phytoplankton (> 20 μm) in the Yeongsan River estuary (Sin et al. 2013). This finding supports a scenario in which fortnightly variation in the water column processes is more closely associated with the timing of episodic freshwater discharge than with the fortnightly tidal cycle.
Semidiurnal Variation in Water Quality and the Phytoplankton Community
In semidiurnal tidal cycles, flood and ebb flows produced by the tide affect physical parameters including salinity, water temperature, and turbidity (Wong 1995; Arndt et al. 2007; Azhikodan and Yokoyama 2016; Morelle et al. 2017). In the current study, salinity, temperature, turbidity, DO, and pH were not correlated with tidal height during either the dry or wet season, suggesting that diel variations in water properties are not predictable during the intratidal cycle. However, salinity was correlated significantly with water temperature, turbidity, DO, and pH (especially during the wet season). Thus, diel variations in water properties were affected by freshwater discharge from the reservoir, where the surface water temperature and turbidity were higher, and the DO and pH lower, than in the saltwater zone during the wet season (Sin et al. 2015b; Song et al. 2015).
The effect of tidal forcing was evident only during NW monitoring, when no freshwater was discharged for 17 days before monitoring. This is supported by the significant correlation between tidal height and surface water salinity and the positive relationship between tidal height and surface water turbidity. This result implies that physical properties are influenced principally by tidal forcing (including semidiurnal flooding and ebbing) for only a limited period, when freshwater discharge is minimal. However, a weak anomaly in tidal salinity variation was observed, in which salinity peaked at low tide but decreased at high tide during NW monitoring. In Asan Bay, a macrotidal (6–9 m) estuary on the north-west coast of Korea that also features a sea embankment, a positive relationship was evident between tidal height and salinity (Sin et al. 2015a), consistent with patterns observed in other estuaries (Lauria et al. 1999; Domingues et al. 2010). The horizontal distribution of salinity may explain this discrepancy, since salinity was lower at Station 2 than at Station 1 of the present study. Estuarine circulation in the Yeongsan River estuary has been described as complicated (Cho et al. 2004), as a result of three-layered water column circulation during spring and summer, when freshwater is frequently discharged, instead of the two-layered circulation typically observed in natural estuaries. These complex circulation patterns, combined with local freshwater inputs, may have contributed to the physical properties observed in the current study; however, further study of estuarine circulation including various sources of freshwater inputs is required to understand the mechanism responsible. Assessment of the complex circulation associated with anthropogenic activities such as the construction of engineered structures in a highly altered estuary will improve our understanding of estuarine function and our management of estuarine ecosystems.
Generally, the chl a level varied out of phase with tidal height, primarily due to longitudinal tidal advection during the semidiurnal cycle (Wetz et al. 2006; Helbling et al. 2010). In Asan Bay, chl a levels also generally varied out of phase with tidal height, a pattern generally observed in natural estuaries (Sin et al. 2015a). A similar pattern (out of phase) was apparent during the dry season. However, the pattern was not as clear as in other estuaries, and the correlation between chl a and tidal height was not significant in our present study. Neither phytoplankton taxonomic structure nor size was correlated significantly with tidal height, indicating that semidiurnal variations in phytoplankton biomass (reflected by chl a levels), size, and taxonomic structure were not predictable in the macrotidal estuary that we evaluated. The absence of any consistent intratidal variation may reflect freshwater discharge 2–3 days before monitoring and (probably) variations in the diel light intensity cycle. This hypothesis was supported by the nMDS analysis showing that diel variations in the phytoplankton community were explained principally by surface water turbidity and salinity, which were in turn affected by freshwater discharge.
At neap tide during the wet season (NW), chl a variation with tidal height differed depending on the semidiurnal scale. Micro-sized phytoplankton levels were correlated negatively with tidal height during the first semidiurnal cycle (S1) of NW monitoring. This size class increased 2-fold and peaked at low tide (13:48) during ebbing over a 5-h period, but it later rapidly decreased to 1.68 μg L−1 (at 19:05) during flooding. This high variation may have been due mainly to tidal advection of the water mass with algal blooms rather than in situ algal growth. This scenario is supported by our finding that the doubling time (1.3 days) estimated near the monitoring site was longer than 5 h.
Chl a levels remained low (6.40–14.68 μg L−1) during the second semidiurnal cycle (S2) of NW monitoring but were correlated positively with tidal height, in contrast to the situation during S1, implying that algal blooms upstream were likely sporadic and patchy as a result of the inherently complex circulation described above. This hypothesis is partly supported by the horizontal distributions of total and size-class chl a lower than 5 μg L−1 for samples collected upstream 1 day before monitoring. This suggests that Lagrangian monitoring or simultaneous monitoring at various locations may be required to better understand the dynamics of phytoplankton especially in highly altered estuaries.
During SW monitoring, the total chl a level increased to 44.56 μg L−1 until 16:38 (S1E2), after which it began to decrease. Freshwater was discharged from 17:42 to 20:30, impacting phytoplankton as well as water properties. The levels of chl a, salinity, DO, and pH decreased acutely, whereas turbidity increased greatly, probably due to inputs of turbid freshwater with low levels of chl a, DO, and pH. The chl a levels of micro-, nano-, and pico-sized phytoplankton decreased during discharge; however, the impact was greater for micro- and nano-sized phytoplankton. Taxonomic composition was also influenced by discharge; dinoflagellates were replaced by cyanobacteria, which have been reported to be dominant in the freshwater zone of the Yeongsan River estuary (Malazarte et al. 2017). This suggests that freshwater phytoplankton are introduced to the saltwater zone via discharge, shifting the taxonomic structure, as revealed by nMDS analysis. The effect of regulated freshwater discharge (from a reservoir) on taxonomic composition has been investigated in a temperate estuary of Europe (Bode et al. 2017). However, freshwater species began to decrease in abundance following the peak at 02:34 during the ebb tide (S2E0) and were no longer dominant at the end of ebbing (S2E4), such that these species appear to survive for only a limited time in the saltwater zone. The results of this study imply that the collection and analysis of freshwater discharge data including the date, starting time, duration, and magnitude of discharge, as well as in situ field data, are important to understanding the behavior of estuaries that have been altered by the construction of sea embankments.
Conclusions
Despite the variation in fortnightly and semidiurnal cycles observed in this study, the effects of tidal forcing on water quality and the phytoplankton community were not predictable. In contrast, freshwater discharged 1–3 days before or during monitoring greatly affected water properties and phytoplankton dynamics. Semidiurnal variations in physical properties and the phytoplankton community were evident only when freshwater input was minimal. However, the pattern differed from those of other estuaries, probably because estuarine circulation was very complex due to the sea embankment. This anthropogenic feature dominated the short-term dynamics, although the estuary is macrotidal and would thus be expected to be dominated by tidal forcing. We thus show that anthropogenic disturbance imposed by engineered structures can be severe in estuaries. In addition, the frequency and magnitude of freshwater discharge into saltwater zones will increase in wet areas in the future, due to climate change. Holistic analysis of hydrodynamic and phytoplankton predation, and Lagrangian rather than Eulerian monitoring, will improve our understanding of phytoplankton dynamics in such complex systems.
References
Adolf, J.E., C.L. Yeager, W.D. Miller, M.E. Mallonee, and J.L.W. Harding. 2006. Environmental forcing of phytoplankton floral composition, biomass, and primary productivity in Chesapeake Bay, USA. Estuarine, Coastal and Shelf Science 67 (1-2): 108–122.
Alvarez-Gongora, C., and J.A. Herrera-Silveira. 2006. Variations of phytoplankton community structure related to water quality trends in a tropical karstic coastal zone. Marine Pollution Bulletin 52 (1): 48–60.
Angara, E.V., G.S. Rillon, M.L. Carmona, J.E.M. Ferreras, M.I. Vallejo, A.C.G.G. Amper, and M.L.D.G. Lacuna. 2013. Diversity and abundance of phytoplankton in Casiguran waters, Aurora Province, Central Luzon, N. Philippines. AACL Bioflux 6: 358–377.
Arndt, S., J.P. Vanderborght, and P. Regnier. 2007. Diatom growth response to physical forcing in a macrotidal estuary: coupling hydrodynamics, sediment transport, and biogeochemistry. Journal of Geophysical Research 112: 1–23.
Azhikodan, G., and K. Yokoyama. 2016. Spatio-temporal variability of phytoplankton (chlorophyll-a) in relation to salinity, suspended sediment concentration, and light intensity in a macrotidal estuary. Continental Shelf Research 126: 15–26.
Barcena, J.F., J. García-Alba, A. García, and C. Álvarez. 2016. Analysis of stratification patterns in river-influenced mesotidal and macrotidal estuaries using 3D hydrodynamic modelling and K-means clustering. Estuarine, Coastal and Shelf Science. 181: 1–13.
Bharathi, M.D., V.V.S.S. Sarma, and K. Ramaneswari. 2018. Intra-annual variations in phytoplankton biomass and its composition in the tropical estuary: influence of river discharge. Marine Pollution Bulletin 129 (1): 14–25.
Bode, A., M. Varela, R. Prego, F. Rozada, and M.D. Santos. 2017. The relative effects of upwelling and river flow on the phytoplankton diversity patterns in the ria of A Coruña (NW Spain). Marine Biology 164: 93.
Byun, D.S., X.H. Wang, and P.E. Holloway. 2004. Tidal characteristic adjustment due to dyke and seawall construction in the Mokpo Coastal Zone, Korea. Estuarine, Coastal and Shelf Science 59 (2): 185–196.
Cho, Y.K., L.H. Park, C. Cho, I.T. Lee, K.Y. Park, and C.W. Oh. 2004. Multi-layer structure in the Youngsan estuary, Korea. Estuarine, Coastal and Shelf Science 61 (2): 325–329.
Cloern, J.E. 1991. Tidal stirring and phytoplankton bloom dynamics in an estuary. Journal of Marine Research 49 (1): 203–221.
Cloern, J.E., and A.D. Jassby. 2012. Drivers of change in estuarine-coastal ecosystems: discoveries from four decades of study in San Francisco Bay. Reviews of Geophysics 50: RG4001.
Cloern, J.E., T.M. Powell, and L.M. Huzzey. 1989. Spatial and temporal variability in south San Francisco Bay (USA). 2. Temporal changes in salinity, suspended sediments, and phytoplankton biomass and productivity over tidal time scales. Estuarine, Coastal and Shelf Science 28 (6): 599–613.
Costanza, R., R. d’Arge, R. de Groot, S. Farber, M. Grasso, B. Hannon, K. Limburg, S. Naeem, R.V. O’Neill, J. Paruelo, R.G. Raskin, P. Sutton, and M. van den Belt. 1997. The value of the world’s ecosystem services and natural capital. Nature 387 (6630): 253–260.
Costanza, R., R. de Groot, P. Sutton, S. van der Ploeg, S.J. Anderson, I. Kubiszewski, S. Farber, and R.K. Turner. 2014. Changes in the global value of ecosystem services. Global Environmental Change 26: 152–158.
Dauvin, J.C. 2008. Effects of heavy metal contamination on the macrobenthic fauna in estuaries: the case of the Seine estuary. Marine Pollution Bulletin 57 (1-5): 160–169.
Diaz, R.J., and R.R. Rosenberg. 2008. Spreading dead zones and consequences for marine ecosystems. Science 321 (5891): 926–929.
Domingues, R.B., T.P. Anselmo, A.B. Barbosa, U. Sommer, and H.M. Galvão. 2010. Tidal variability of phytoplankton and environmental drivers in the freshwater reaches of the Guadiana estuary (SW Iberia). International Review of Hydrobiology 95 (4-5): 352–369.
Froneman, P.W., E.A. Pakhomov, and M.G. Balarin. 2004. Size-fractionated phytoplankton biomass, production and biogenic carbon flux in the eastern Atlantic sector of the Southern Ocean in late austral summer 1997–1998. Deep-Sea Research (Part II, Topical Studies in Oceanography) 51: 2715–2729.
Garrett, C.J.R., J.R. Keeley, and D.A. Greenberg. 1978. Tidal mixing versus thermal stratification in the Bay of Fundy and Gulf of Maine. Atmosphere–Ocean 16 (4): 403–423.
Harrison, P.J., S. Piontkovski, and K. Al-Hashmi. 2017. Understanding how physical–biological coupling influences harmful algal blooms, low oxygen and fish kills in the Sea of Oman and the Western Arabian Sea. Marine Pollution Bulletin 114 (1): 25–34.
Helbling, E., D.E. Perez, C.D. Medina, M.G. Lagunas, and V.E. Villafane. 2010. Phytoplankton distribution and photosynthesis dynamics in the Chubut River estuary (Patagonia, Argentina) throughout tidal cycles. Limnology and Oceanography 55 (1): 55–65.
Kang, J.W. 1999. Changes in tidal characteristics as a result of the construction of sea-dike/sea-walls in the Mokpo Coastal Zone in Korea. Estuarine, Coastal and Shelf Science 48 (4): 429–438.
Kang, J.W., and K.S. Jun. 2003. Flood and ebb dominance in estuaries in Korea. Estuarine, Coastal and Shelf Science 56 (1): 187–196.
Kennish, M. 2002. Environmental threats and environmental future of estuaries. Environmental Conservation 29 (1): 78–107.
Kim, Y.-G., and J.H. Chang. 2017. Long-term changes of bathymetry and surface sediments in the dammed Yeongsan River estuary, Korea, and their depositional implication. Journal of Korean Society of Oceanography 22: 88–102.
Kolber, Z.S., O. Prasil, and P.G. Falkowski. 1998. Measurements of variable chlorophyll fluorescence using fast repetition rate techniques: defining methodology and experimental protocols. Biochimica et Biophysica Acta 1367 (1-3): 88–106.
Landsberg, J.H. 2002. The effects of harmful algal blooms on aquatic organisms. Review in Fisheries Science 10 (2): 113–390.
Lauria, M.L., D.A. Purdie, and J. Sharples. 1999. Contrasting phytoplankton distributions controlled by tidal turbulence in an estuary. Journal of Marine Systems 21 (1-4): 189–197.
Lee, Y.G., K.-G. An, P.T. Ha, K.-Y. Lee, J.-H. Kang, S.M. Cha, K.H. Cho, Y.S. Lee, I.S. Chang, K.-W. Kim, and J.H. Kim. 2009. Decadal and seasonal scale changes of an artificial lake environment after blocking tidal flows in the Yeongsan Estuary region, Korea. Science of the Total Environment 407 (23): 6063–6072.
Li, M., and L. Zhong. 2009. Flood–ebb and spring–neap variations of mixing, stratification and circulation in Chesapeake Bay. Continental Shelf Research 29 (1): 4–14.
Litaker, W., C.S. Duke, B.E. Kenney, and J. Ramus. 1993. Short-term environmental variability and phytoplankton abundance in a shallow tidal estuary. II. Spring and fall. Marine Ecology Progress Series 94: 141–154.
Longhurst, A., S. Sathyendranath, T. Platt, and C. Caverhill. 1995. An estimate of global primary production in the ocean from satellite radiometer data. Journal of Plankton Research 17 (6): 1245–1271.
Malazarte, J.M., H. Lee, H.-W. Kim, and Y. Sin. 2017. Spatial and temporal dynamics of potentially toxic cyanobacteria in the riverine region of a temperate estuarine system altered by weirs. Water 9 (11): 819.
Malone, T.C., and M.B. Chervin. 1979. The production and fate of phytoplankton size fractions in the plume of the Hudson River, New York Bight. Limnology and Oceanography 24 (4): 683–696.
McLusky, D.S., and M. Elliott. 2004. The estuarine ecosystem: ecology, threats, and management. New York: Oxford University Press.
Morelle, J., M. Schapira, and P. Claquin. 2017. Dynamics of phytoplankton productivity and exopolysaccharides (EPS and TEP) pools in the Seine Estuary (France, Normandy) over tidal cycles and over two contrasting seasons. Marine Environmental Research 131: 162–176.
Nayar, S., B.P.L. Goh, and L.M. Chou. 2005. Dynamics in the size structure of Skeletonema costatum (Greville) Cleve under conditions of reduced photosynthetically available radiation in a dredged tropical estuary. Journal of Experimental Marine Biology and Ecology 318 (2): 163–182.
Olofsson, M., M. Karlberg, S. Large, and H. Ploug. 2017. Phytoplankton community composition and primary production in the tropical tidal ecosystem, Maputo Bay (the Indian Ocean). Journal of Sea Research 125: 18–25.
Sin, Y., and B. Jeong. 2015. Short-term variations of phytoplankton communities in response to anthropogenic stressors in a highly altered temperate estuary. Estuarine, Coastal and Shelf Science 156: 83–91.
Sin, Y., B. Hyun, B. Jeong, and H. Soh. 2013. Impacts of eutrophic freshwater inputs on water quality and phytoplankton size structure in a temperate estuary altered by a sea dike. Marine Environmental Research 85: 54–63.
Sin, Y., B. Jeong, and C. Park. 2015a. Semidiurnal dynamics of phytoplankton size structure and taxonomic composition in a macrotidal temperate estuary. Estuaries and Coasts 38 (2): 546–557.
Sin, Y., E. Lee, Y. Lee, and K. Shin. 2015b. The river–estuarine continuum of nutrients and phytoplankton communities in an estuary physically divided by a sea dike. Estuarine, Coastal and Shelf Science 163: 279–289.
Smith, V.H. 2006. Responses of estuarine and coastal marine phytoplankton to nitrogen and phosphorus enrichment. Limnology and Oceanography 51 (1part2): 377–384.
Smyth, T.J., K.L. Pemberton, J. Aiken, and R.J. Geider. 2004. A methodology to determine primary production and phytoplankton photosynthetic parameters from fast repetition rate fluorometry. Journal of Plankton Research 26 (11): 1337–1350.
Song, E.-S., K.-A. Cho, and Y.-S. Shin. 2015. Exploring the dynamics of dissolved oxygen and vertical density structure of water column in the Youngsan Lake. Journal of Environmental Science International 24 (2): 163–174.
Vargas, C.A., and H.E. Gonzalez. 2004. Plankton community structure and carbon cycling in a coastal upwelling system. I. Bacteria, microprotozoans and phytoplankton in the diet of copepods and appendicularians. Aquatic Microbial Ecology 34: 151–164.
Wetz, M.S., K.C. Hayes, A.J. Lewitus, J.L. Wolny, and D.L. White. 2006. Variability in phytoplankton pigment biomass and taxonomic composition over tidal cycles in a salt marsh estuary. Marine Ecology Progress Series 320: 109–120.
Wong, K.-C. 1995. The hydrography at the mouth of Delaware Bay: tidally averaged distribution and intratidal variability. Estuarine, Coastal and Shelf Science 41 (6): 719–736.
Wu, X., H. Duan, N. Bi, P. Yuan, A. Wang, and H. Wang. 2016. Interannual and seasonal variation of chlorophyll a off the Yellow River mouth (1997–2012): dominance of river inputs and coastal dynamics. Estuarine, Coastal and Shelf Science 183 (Part B): 402–412.
Yang, E.R., B.K. Jeong, E.J. Lee, D.K. Ryu, and Y.S. Shin. 2014. Size and species composition of phytoplankton related to anthropogenic environmental changes in Doam Bay. Journal of Environmental Science International 23 (6): 1183–1197.
Zhou, W., J. Gao, J. Liao, R. Shi, T. Li, Y. Guo, and A. Long. 2016. Characteristics of phytoplankton biomass, primary production and community structure in the Modaomen Channel, Pearl River estuary, with special reference to the influence of saltwater intrusion during neap and spring tides. PLoS One 11 (12): e0167630.
Acknowledgments
We thank the two anonymous reviewers for their constructive comments, which helped us to improve the manuscript.
Funding
This research was supported by the Basic Science Research Program of the National Research Foundation of Korea (NRF) funded by the Ministry of Education (grant no. 2018R1D1A1B05049865).
Author information
Authors and Affiliations
Corresponding author
Additional information
Communicated by Dennis Swaney
Rights and permissions
About this article
Cite this article
Sin, Y., Jeong, B. Anthropogenic Disturbance of Tidal Variation in the Water Properties and Phytoplankton Community of an Estuarine System. Estuaries and Coasts 43, 547–559 (2020). https://doi.org/10.1007/s12237-019-00557-7
Received:
Revised:
Accepted:
Published:
Issue Date:
DOI: https://doi.org/10.1007/s12237-019-00557-7