Abstract
As a ‘star molecule’, graphene is widely used in technical fields such as environmental pollutant treatment due to its special properties such as ultra-high carrier mobility, π–π conjugated structure and unique electrochemical properties. This article reviews the recent applications of graphene and its derivatives for adsorption and enrichment of pollutants in the aqueous environment, atmosphere and soil, including the adsorption mechanism of graphene in the treatment of some representative contaminants and the influencing factors of adsorption performance. We hope to use this article as a glimpse of the prospects of graphene applications in environmental pollutants.
Similar content being viewed by others
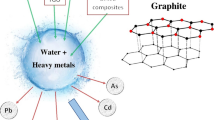
Explore related subjects
Discover the latest articles, news and stories from top researchers in related subjects.Avoid common mistakes on your manuscript.
1 Introduction
The structure and morphology of graphene are very free to change. They can be wrapped in layers or folded directly to form zero-dimensional fullerene, rolled to form one-dimensional graphene carbon nanotubes, or they can be stacked to form three-dimensional graphite [1] (see figure 1). These materials of different dimensions together form a complete family of carbon materials. Graphene and its derivatives have a hydrophobic surface and possess a very high theoretical surface area. The delocalized π-electrons on the surface provides stability to graphene over other nanosystems and makes it easily bind to other atoms with a strong adsorption capacity. Besides, the properties of graphene can be fully utilized due to its abundant surface groups. Among its numerous notable features, its pore structure is the most beneficial feature for sewage treatment, with pores that allow water molecules to pass through while filtering out macromolecular contaminants. Therefore, it is used as a good adsorbent material, and because of its high mechanical strength, it can also be used as a support for nanomaterial complexes [2,3,4,5,6]. Graphene and its derivatives are very similar in structure. They are all carbonaceous materials with a single layer of carbon atoms in thickness and the carbon atoms are compactly arranged into a honeycomb pattern with sp2 hybridization [7,8]. The researchers have made them as nanoscale particles to improve their adsorption performance due to the small particle size (large specific surface area). The nano-carbon material was applied to the remediation of environmental pollutants and found that it has excellent catalytic degradation efficiency [9,10].
The basic building block for graphene materials of all other dimensionalities [1].
The pristine graphene is the first 2D carbon crystal with honeycomb-like structure and free π–π electrons, contributing to the cleaning of pollutants via π–π stack interaction and hydrophobic effects. However, it is also due its large π-conjugated structure and strong hydrophobic performance that limits its application in removing contaminants from water. Subsequently, graphene oxide (GO) and reduced graphene oxide (rGO), as the derivatives of the graphene, show better performance towards adsorption of various pollutants. GO can be synthesized from graphite using the Hummers and Offeman method [11] or its advanced modification [12,13]. Compared to graphene, GO and its reduced form contain C:O ratio of 2-4:1 and 8-246:1, respectively [13]. The presence of oxygen functionalities in graphene derivatives makes them suitable materials for the adsorption application. The oxygen-containing function groups, like carboxyl group, epoxy group and hydroxyl group, are more attractive and have been extensively employed for the cleaning of numerous pollutants from waters, solid pollutants and air contamination. Besides, GO also can be functionalized with inorganic particles like metal, non-metals and their oxide nanoparticles.
Graphene has excellent physical and chemical properties, and its composites have broad application prospects in the fields of materials, biomedicine, water purification, nanoelectronic devices and so on [14,15]. The preparation of graphene has always been the focus of materials science research. Researchers are also working to find more efficient, convenient and cheaper ways to fabricate graphene. After decades of research, graphene preparation methods are becoming more and more diverse, and the main preparation methods include mechanical exfoliation [16], chemical oxidation-reduction [17], chemical vapour deposition [18], SiC epitaxial growth [19], and electrochemistry [20], as shown in table 1.
For the past few years, different graphene derivatives were reported in the treatment of environment contamination. This review mainly combines three types of typical environmental pollutants: (i) water pollutants; (ii) solid pollutants and (iii) air contamination. Besides, the adsorption and enrichment mechanism of graphene and its derivative materials on environmental samples will be introduced here. Finally, the current challenges of graphene materials in practical applications are described, and the prospect of graphene nanomaterials in pollutant analysis is presented.
2 Adsorption and enrichment of water pollutants by graphene and its derivative materials
At present, the adsorption effect of nanomaterials on pollutants in water can be roughly divided into two types: one is physical adsorption without producing any new substances, and the other is chemical adsorption with chemical reactions. Physical adsorption mainly refers to the adsorption of heavy metals by nanomaterials through intermolecular force, electrostatic attraction, hydrophobic interaction, and π-bond interaction. Among the many interactions, the electrostatic interaction is the most important factor that physical adsorption can achieve, and at the same time also related to the recovery performance of the adsorbent after use. Li et al. [21] found that the core principle of composite nanomaterials made of graphene and Fe3O4 for immobilized adsorption of Cu(II) in water is mainly physical adsorption. Wang’s research team revealed how the above nanoparticle–graphene complexes adsorb Co(II) and U(IV) when multiple heavy metal ions coexist in the water [22,23]. The core of the process is the co-adsorption of heavy metal ions by the composite nanoparticles. This adsorption follows the Langmuir isotherm adsorption model, and the process is very mild, without the introduction of energy, but a spontaneous endothermic process.
However, any material has inevitable shortcomings. For pure graphene, although its structure is intact and chemically stable, its solubility in water and most solvents (such as methanol, ethanol, etc.) is low and the strong π–π stacking between its layers leads to a decrease in particle dispersion in water and irreversible agglomeration. These unfavourable aspects restrict the binding of pure graphene with foreign molecules to a certain extent, and narrow its application range. Therefore, it is imperative to modify or functionalize the surface of graphene, so that this material can shine in the environmental field and realize more abundant applications.
Graphene oxide (GO) is the most in-depth study of many derivatives of graphene. Due to oxidation by strong oxidants, the vacant sites will carry a large number of active oxygen-containing groups on the surface of graphene. Hydroxyl, carboxyl, epoxy, etc., most of these groups are polar, so the solubility of GO in water is further improved. The electrostatic interaction between GO and other metal cations is relatively strong and can exchange with anions and cations in water due to the presence of the oxygen-containing groups [24,25]. These properties are unmatched by ordinary graphene. The dispersibility of GO in water and the ability of compatibility with organisms are better than ordinary pure graphene. And the existence of these newly introduced groups provides a large number of active sites for deep chemical modification or functionalization. Since the groups on the GO surface can complex with the metal cations through shared electron pairs to form stable polymers, it is possible for the metal ions in the water to be adsorbed on them through the complexation reaction.
On this basis, new groups can be further introduced so that polymers and hydrophilic molecules can be inserted into the GO layer by ionic bonding and other interactions, giving it more excellent properties. Another important reason why GO has a wider range of applications than pure graphene is that its synthesis conditions are relatively mild, and the raw materials are relatively cheap and easy to obtain, and the cost is low. Wan et al. [26] prepared a new type of composite adsorbent (HMO@GO), which is obtained by the in-situ reaction on GO nanosheets. It can be seen that when the content of Ca2+ in the solution is high, HMO@GO shows an obvious selectivity for adsorption of Pb2+, and the sites occupied by Ca2+ can be completely replaced by Pb2+ instead of other metal cations through ion exchange. This specificity greatly improves the utilization efficiency of the adsorbent, and its adsorption capacity can reach up to 500 mg g–1, which is much higher than that of ordinary GO [26] (figure 2).
Schematic diagram of HMO@GO selective adsorption of Pb2+ [26].
As mentioned earlier, due to the strong hydrophobicity of graphene, the surface of graphene is easy to agglomerate, which limits its application of adsorption performance. In order to solve these shortcomings, many scholars have modified them to prepare many composites with superior properties. Chen et al. [27] used Pickering emulsion as a raw material to prepare high-strength three-dimensional macroporous polystyrene microsphere/graphene oxide (PS/GO) composites with a pore diameter of 4–20 μm. This composite material has a strong adsorption capacity for tetracycline (TC), which varies with the pH of the solution. When the pH of the solution is 6, the maximum adsorption capacity of TC is 197.9 mg g–1 [27] (figure 3). Most researchers used polysorbates as a template to prepare a hollow adsorption material. Other scholars such as Yang et al. [28] reported an hollow nanocomposite. NH2-MIL-53(Al)/rGO grown from the surface of the hollow graphene microspheres were prepared by using reduced rGO-wrapped polystyrene microspheres as template. After 210 min of continuously visible-light irradiation, the degradation efficiency of methylene blue (MB) can reach up to 59%, and the degradation rate constant of MB towards NH2-MIL-53(Al)/rGO (k = 0.0039 min–1) is higher than that of P25-TiO2 (k = 0.0019 min–1) and NH2-MIL-53 (k = 0.0021 min–1) 2 times and 1.85 times [28]. Prabhakarrao et al. [29] synthesized Zr-doped TiO2/rGO (ZTG) nanocomposites under relatively mild conditions and adopted a new modified sol–gel method. Among this kind of nanocomposite material, ZTG5 (5 wt% GO) exhibits the best performance for efficient photocatalytic degradation of eosin blue (EB) dye under visible-light irradiation. The degradation rate of EB reaches 96% within 90 min, which is nearly complete [29].
Illustration of the preparation process of microporous PS/GO composite monolith [27].
In recent years, some environmental pollutants like pharmaceuticals and personal care products (PPCPs) widely used in human society have emerged in water bodies [30]. Although the half-life of PPCPs is not very long, the large and frequent use by humans and livestock has led to a false persistence phenomenon known as ‘pseudo-persistent organics pollutants’, widespread in the water environment [31]. They will remain in the environmental media for a very long time through various pathways and cannot be decomposed by photocatalysts. Moreover, they are inherently toxic, and the transfer of this toxicity through the food chain will amplify this toxicity step by step, ultimately posing a risk to human health. It is because they are some polymeric organic substances that are difficult to be degraded and have an effect of biomass accumulation that this phenomenon is gaining more and more attention. Moussavi et al. [32] described a double-oxidized graphene oxide (DGO) with a much higher oxidability than its raw materials. They used this modified material for the adsorption of acetaminophen drug (ACT) in water. The adsorption of DGO on ACT can be regarded as single-layer adsorption. The maximum adsorption capacity at equilibrium can achieve to 690 mg g–1 according to the Langmuir’s model, which is far higher than the surface adsorption of conventional activated carbon due to the high density of oxygen functional groups in DGO [32]. Jauris et al. [33] carefully explored the adsorption effect of rGO on sodium diclofenac drug (s-DCF) and the complex adsorption principle. It was found that the principle of s-DCF adsorption by rGO is hydrogen bond and π–π stacking interactions, and the maximum adsorption capacity is 59.67 mg g–1. Besides, further research tells us that the adsorption of s-DCF by rGO belongs to physical adsorption. There is no chemical bond breaking in the process, and this process is reversible under appropriate conditions [33].
3 Adsorption and enrichment of atmospheric pollutants by graphene and its derivative materials
Industrial emissions that are released into the atmosphere, particularly through rainfall deposition, volatilization (input from diffuse sources) and accidental spills, constitute a cumulative, persistent and harmful terror to the survival of flora, fauna and environmental substrates (water and soil). It is well known that adsorption is a selective and simple gas infiltration and purification technique. Recently, graphene-based materials are popular for the removal of various gaseous air pollutants, such as ammonia (NH3), hydrogen sulphide (H2S), sulphur dioxide (SOx) or nitrogen oxides (NOx), as well as for the purification of natural gases, such as methane, CO2, N2 and O2 [34].
At present, the research on the application of carbonaceous materials in air purification mainly focuses on two optimized materials, GO and rGO. The results show that the properties and existing forms of graphene and its derivatives are seriously affected by the preparation methods. The functionalised GO has a larger specific surface area and more stable structure with surprising adsorption capacity to remove particulate and chemical pollutants effectively. The application of graphene materials and their derivatives in air purification mainly focuses on the adsorption of graphene materials in air purification, and there are also some studies on filtration, bacteriostatic and photocatalysis [34,35]. Some scientists are also working on further catalytic activation of graphene materials to improve the porosity and pore volume of the material, and the resulting solid adsorbents are mainly used for the adsorption and capture of volatile organic compound (VOC) gas [36].
As a major class of air pollutants, VOCs, like methanol, acetone, acetaldehyde, formaldehyde, THF, benzene and toluene, consist of various hydrocarbons with high vapour pressure. They can exist ubiquitously in both outdoor and indoor air due to their emissions from various source processes [34]. Kim et al. [37] treated GO powder with microwave radiation and quickly reduced it to get rGOMW (graphite oxide with microwave treatment) powder. Furthermore, rGOMW powder was activated with KOH to obtain rGOMWKOH (GO with microwave treatment and KOH activation) powder for adsorption of VOCs. Adsorption experiments of benzene and acetaldehyde with low concentration (30 ppm) showed that the maximum volume capacity of toluene gas and acetaldehyde gas contained by rGOMWKOH powder was 3510 and 630 m3 g–1, respectively [37]. Shirkhanloo and group [38] used a simple and fast method based on bismuth oxide nanoparticles coupled to heterogeneous graphene/graphene oxide (BONPs-NG/NGO) for the rapid removal of xylene vapour from environmental air by UV photocatalytic degradation-adsorption procedure (UV-PCDA). By static system in the presence of UV irradiation, the capacity adsorption of xylene with 200 mg of BONPs-NG/NGO and NG/NGO was obtained as 223 and 134.6 mg g–1 in 85°C for 10 min, respectively [38]. Metal-organic framework (MOFs) is a kind of crystalline material composed of ionic clusters and organic connectors. MOFs-based materials are more stable, have more modifiable sites and have certain catalytic effect. Ding et al. [39] developed chemically functionalized 3D graphene oxide hydrogels (FGH) decorated with MOFs-derived Co3O4 nanostructures, in which the Co3O4 nanostructures are uniformly distributed in 3D FGH frameworks. It is found that the Co3O4/FGH composites exhibits a distinct cross-selectivity for acetone against other interfering gases (1 ppm) [39]. Besides, Szczęśniak et al. [40] recorded that graphene–MOF composite materials were prepared from mixed porous materials composed of metal-organic skeleton and graphene through in-situ obtaining materials with higher crystallinity or physical mixing, which had good adsorption performance for CO2, H2, VOC and methane [40].
Graphene’s excellent photocatalytic activity has also been applied to the treatment of air pollutants. Photocatalytic technology can make use of inexhaustible solar energy to make pollutants undergo redox reaction, which has a broad application prospect. Pei et al. [41] compounded graphene (Gr) sheets with TiO2/ZnO/Bi2O3 (TZB), and prepared TZB-Gr composite nanofibres by a new sol–gel-based electrospinning process. The nanofibre is fabricated with its surface packed with 10-nm size TZB nanocrystallites that increases the surface area, thereby improving light harvesting. The TZB-Gr photocatalyst, after optimized with as much as 26.5% by mass of graphene in the nanofibres, has superior photoactivity in degradation of NO under solar irradiation [41]. Kanjwal and Leung [42] introduced the graphene into circular rolls inserted in the 65–140 nm TiO2 (T), TiO2–CuO (TC) and TZB nanofibres to obtain several high-performance photocatalyst, such as T-Gr, TC-Gr and TZB-Gr. Under the irradiation of visible light, the holes and electrons generated by the intervening light can react with water molecules adsorbed on the catalyst surface to generate hydroxyl groups. The generated hydroxyl groups play an important role in the photocatalytic degradation of HCHO. The degradation effect of these composites on formaldehyde is obvious and much higher than that of the precursors. Detailed reaction mechanism is given below [42]. Trapalis et al. [43] prepared surfactant-stabilized graphene (ssG) materials using liquid phase exfoliation, and then synthesized TiO2–graphene (Gr) composite photocatalysts (TiO2/Gr, TiO2/rGO) by solvothermal process. Both of them have high photocatalytic degradation efficiency for nitrogen oxides (NO, NO2), and the photocatalytic efficiency of TiO2/rGO is better than that of TiO2/Gr even when the concentration of graphene is lower than 0.1% [43].
4 Adsorption and enrichment of miscellaneous pollutants by graphene and its derivative materials
Nitroaromatic compounds (NACs) are intermediates that are widely used in pesticides, explosives and synthetic compounds. How to reduce and eliminate nitrobenzene substances in the environment has always been the focus of the environment [44,45,46]. NACs have high adsorption properties and are often adsorbed on sediments in groundwater, which changes their mobility and bioavailability. However, the adsorption process only changes the form of NACs in the environmental system, and its potential environmental risks have not been eliminated. Recent studies have found that the effect of graphene on microorganisms is closely related to concentration. Low concentrations of graphene can promote microbial metabolism, while high concentrations have an inhibitory effect. Chemically synthesized graphene and GO can promote the reduction of iron-reducing bacteria Shewanella oneidensis MR-1 and activated sludge to nitrobenzene and dye compounds [47,48]. Adding biochar to the paddy soil contaminated by pentachlorophenol (PCP) can increase the conversion rate of microbes to pentachlorophenol so that the contaminated soil can be repaired. In this process, biochar can not only promote the extracellular electron transfer process of microorganisms, but also promote the growth and metabolism of microorganisms in the soil, which together promote the reductive dechlorination of PCP [49].
Researchers generally believe that nano-carbon materials are harmful to biological cells and may inhibit biodegradation activity. The reason for the potential toxicity is determined by its structure, because the edges of graphene-based nanomaterials are not very neat, but have many tooth-like structures. These sharp edges may reduce the activity of bacterial cells by piercing the cell membrane of organisms or unbalanced oxidation and antioxidant effects in organisms, resulting in tissue damage [50].
5 Parameters influencing the adsorption and enrichment efficiency of graphene and its derivatives
5.1 Solution pH
The pH of the solution can change the electromotive force on the graphene surface and affect the interaction between particles. The electromotive force is mainly an indicator of how the stability of colloidal particles changes as the pH of the water changes. Not only the pH value of the solution is a key indicator that affects the adsorption performance of graphene, but also the value of the graphene isoelectric point (IEP) that affects the dispersion of the suspension worthy of attention. The isoelectric point is the pH value under special circumstances. Currently, there is no net charge on the surface of the molecule. The pH value of the solution is closely related to the physical properties of the graphene material, such as density and osmotic pressure. According to reports, the IEP of graphene is around pH 3.8–4.7, while the IEP of GO is less than 3 [51]. The electromotive force is mainly used to compare the dispersion of the colloid in the solution. The greater the electromotive force, the more stable the system.
When the pH value of the solution system is close to the isoelectric point, the zeta potential of the graphene surface gradually tends to zero, and the electrostatic force between the layers is very weak, even to the point where it can be ignored, so it is easy to form irreversible agglomeration and precipitate down. In general, we do not want agglomeration to occur, as this will make the whole adsorbed particles unstable and poorly dispersed.
When the pH value of the solution system is far from the isoelectric point, the same charge will be attached to the surface of the graphene, which evident with the change in pH. The farther away from the isoelectric point, the greater the electrostatic repulsion between graphene particles, which hinders the agglomeration of graphene. Lanphere et al. [51] and Chowdhury et al. [52] have shown that pH is the most important factor in determining adsorption. In the normal pH range (4.0–10.0) of the environment, the pH value will not significantly affect the hydrodynamic diameter and electrophoretic mobility (EPM) of GO. But when the pH is within the acidic range, the average EPM of GO will decrease significantly and the electrostatic repulsion on the surface will immediately weaken if the pH drops from 4.0 to 2.0 [51,52].
Vu et al. [53] pointed out that the pH value of the solution will affect the surface properties of carbonaceous materials and the existence of target metal ions, thereby regulating the adsorption process. Li et al. [54] explored the influencing factors of the adsorption of ciprofloxacin (CIP) by nitrilotriacetic acid-functionalized magnetic graphene oxide (NDMGO), and found that the adsorption rate gradually increased with the alkalinity of the solution, and the changing trend was first to increase then decrease. As shown in figure 4, when the pH = 9, the maximum adsorption capacity of CIP is 230.57 mg g–1 [54].
(a) pH effect on CIP adsorption onto NDMGO with and without 0.01–1.0 mg l–1 Cu(II); (b) effect of Cu(II) concentration on CIP adsorption at pH 9.0. C0(CIP) = 50 mg l–1, m/V = 0.10 g l–1, T = 25°C, t = 14 h [54].
5.2 Adsorption time
Generally speaking, the adsorption rate of nanomaterials to pollutants in the water body will become faster and faster over time since the pollutant concentration and adsorbent activity are relatively maintained at a relatively high level during the initial stage of adsorption. Once the balance is reached, the efficiency will not change again. Chen et al [58] devoted themselves to exploring the efficiency of graphene oxide/Fe3O4 (GO/Fe3O4) composites for adsorbing Cu(II) and fulvic acid (FA) in water. They found that the sorption of Cu(II) and FA on GO/Fe3O4 increase rapidly during the first 5 and 16 h, then remains constant with increasing contact time [21]. The reason for the phenomenon that the rate first increases and then decreases is that the adsorption site of nanomaterials and the concentration of adsorbed ions are almost constant at the beginning of adsorption, and the local concentration is quite high, which is conducive to the rapid occurrence of the reaction. Once the adsorption sites are all occupied by adsorbed ions, the nanomaterials will not continue to interact with the remaining heavy metal ions. Raghubanshi et al. [55] investigated the adsorption of GO on Pb2+ and the adsorption rate reached 96% after only 5 min and 98% after 10 min, at which time the reaction basically reached equilibrium (figure 5). This observation is consistent with previous report such as, Huang et al [56] investigated the adsorption of Pb2+ from aqueous solution low-temperature exfoliated graphene nanosheets and about 85% of the Pb2+ was removed within the initial 5 min.
The effect of contact time on the amount of Pb2+ adsorption [55].
5.3 Ionic strength and salt solution type
According to the DLVO theory [57], when the strength of anions and cations in the solution is large, the electric double layer of colloids with different charges will be compressed, so that the electrostatic repulsion between the colloids is greatly reduced, with the potential barrier of the colloids decreasing, prompting the coagulation of the graphene colloidal particles further. The role and stability of GO during coagulation in the aqueous environment must comply with the theory of graphene colloid coagulation. Lanphere et al [51] revealed that the hydraulic diameter and ion electrophoresis mobility (EPM) of graphene in a water environment vary with the ionic strength (IS). The greater the IS in the solution (10−3 to 10−1 M KCl), the greater hydrodynamic diameter, while EPM had the opposite trend [51]. For example, the adsorption capacity of GO for tetracycline decreases with increasing IS in solution because the increase in ionic strength directly inhibits the electrostatic interaction that occurs between the positively charged graphene amino group and the dissociated carboxyl groups in solution. Chen et al [58] showed that the content of NaClO4 affects the adsorption capacity of GO on Ni2+. Because the increase of IS can reduce the electrostatic repulsion and increases the aggregation of GO in aqueous solutions, which thereby decreases the number of available binding sites for the uptake of Ni2+ [58]. Besides, Bi et al [59] pointed out that in the study of the adsorption mechanism of GO on Cu2+, when the NaCl concentration in the solution is zero, the maximum equilibrium adsorption capacity of GO on Cu2+ can reach to 91.6 mg g–1, but when the NaCl concentration in the solution increased slightly to 0.05 mol l–1, the maximum adsorption capacity decreased to 72.7 mg g–1, a decrease of about 25%. After that, the concentration of NaCl continued to increase, and the adsorption capacity did not change much and remains almost stable [59] (figure 6). This is because in the case of high ionic strength, GO will undergo irreversible agglomeration, which greatly reduces the number of surface active sites, and the common ion effect also affects the adsorption capacity, as Na+ and Cu2+ compete with each other for adsorption sites on GO, resulting in a decrease in adsorption capacity.
The effect of ionic strength on the adsorption of Cu2+ by GO [59].
6 Outlook
At present, the research on graphene in environmental pollutants focuses more on the preparation of sewage adsorption materials, while the research on the adsorption mechanism needs to be in-depth, and it may be one of the main directions of future research to further investigate the interaction between pollutant molecules and graphene at the molecular level. At the same time, since graphene is affected by many aspects when adsorbing pollutants in water, such as the nature of the graphene material itself, the molecular structure of the pollutants, and the environmental temperature, etc., it obviously increases the complexity of studying the adsorption mechanism. Moreover, such interactions are often not monolithic, so they cannot be explained by simple adsorption theory.
Graphene nanomaterials are an emerging field, and their advantages in practical applications cannot be ignored, but how to recycle them after use is also something we should think about. In the application of GO in natural water, surface modification can be considered to prepare adsorbent materials with good mechanical properties and easy recovery by introducing other functional groups or combining them with other carrier materials.
Graphene nanomaterials have many properties different from their macroscopic state because of their special particle size. Therefore, we should make further use of this advantage and strive to establish new analysis techniques such as miniaturization, automation and visibility to provide new ideas for solving the difficulties in the analysis and determination of pollutants. At the same time, since graphene materials are inherently toxic to some degree and can absorb some toxic chemical substances, they may trigger a series of migration after entering the environment, thereby changing the biological effects of these pollutants. The cumulative effects of graphene materials in plants or animals, as well as whether and how long it takes to degrade in the environment, and what effects it will have, should also receive more attention.
Graphene materials have great potential and advantages in the adsorption of environmental pollutants, although there are still many practical problems that have not been solved, we look forward to a breakthrough in developing graphene materials for the adsorption and purification of pollutants in the future.
References
Geim A K and Novoselov K S 2007 Nat. Mater. 6 183
Sreeprasad T S, Maliyekkal S M, Lisha K P and Pradeep T 2011 J. Hazard. Mater. 186 921
Li B, Cao H and Yin G 2011 J. Mater. Chem 21 13765
Li B, Cao H, Yin J, Wu Y A and Warner J H 2012 J. Mater. Chem 22 1876
Maliyekkal S M, Sreeprasad T, Krishnan D and Waghmare U V 2013 J. Small 9 273
Gupta S S, Sreeprasad T S, Maliyekkal S M, Das S K and Pradeep T 2012 ACS Appl. Mater. Interfaces 4 4156
Tian L, Li Y, Chen Y and Ji P 2018 J. Appl. Polym. Sci. 135 45834
Yazdi G R, Akhtar F, Ivanov I G and Schmidt S 2019 Appl. Surf. Sci. 486 239
Li F, Jiang X, Zhao J and Zhang S 2015 Nano Energy 16 488
Stern P C, Webler T and Smal M J 2014 Environ. Sci. Technol. 48 8287
Hummers W S and Offeman R E 1958 J. Am. Chem. Soc. 208 1334
Lin Y, Tian Y, Sun H and Hagio T 2020 Chemosphere 270 129
Hiew B, Lee L Y, Lee X J and Gan S 2018 Process Saf. Environ. Prot. 116 262
Zhou X and Liang F 2014 Curr. Med. Chem. 21 855
Baig N, Ihsanullah, Sajid M and Saleh T A 2019 J. Environ. Manage. 244 370
Yi M and Shen Z 2015 J. Mater. Chem. A 3 11700
Kumar N, Salehiyan R, Chauke V and Ray S S 2021 FlatChem 27 100224
Manawi Y M, Ihsanullah, Samara A and Atieh M A 2018 Materials 11 822
Wang Y 2019 IOP Conf. Ser.: Mater. Sci. Eng. 677 022121
Hung Y-F, Cheng C, Huang C-K and Yang C-R 2019 Nanomaterials 9 376
Li J, Zhang S, Chen C and Zhao G 2012 ACS Appl. Mater. Interfaces 4 4991
Liu M, Chen C, Hu J, Wu X and Wang X 2011 J. Phys. Chem. C 115 25234
Sun Y, Yang S, Chen Y and Wang X 2015 Environ. Sci. Technol. 49 4255
Dreyer D R, Park S, Bielawski C W and Ruoff R S 2010 Chem. Soc. Rev. 3 228
Lin Y, Tian Y, Sun H and Hagio T 2021 Chemosphere 270 129420
Wan S, He F, Wu J and Wan W 2016 J. Hazard. Mater. 314 32
Chen L, Wang W, Wang M Z, Yang J and Ge X W 2016 Chin. Chem. Lett. 27 511
Yang Y, Wang W, Li H, Jin X, Wang H, Zhang L et al 2017 Mater. Lett. 197 17
Prabhakarrao N, Chandra M R and Rao T S 2017 J. Alloys Compd. 694 596
Cizmas L, Sharma V K, Gray C M and McDonald T J 2015 Environ. Chem. Lett. 13 381
Dodgen L K and Zheng W 2016 Chemosphere 156 286
Moussavi G, Hossaini Z and Pourakbar M 2016 Chem. Eng. 287 665
Jauris I M, Matos C F, Saucier C and Zanella I 2016 Phys. Chem. Chem. Phys. 18 1526
Samaddar P, Son Y, Tsang D C, Kim K and Kumar S 2018 Coord. Chem. Rev. 368 93
Cao B, Cao S, Dong P and Jing Gao 2013 Mater. Lett. 93 349
Wang S, Tristan F, Minami D and Kaneko 2014 Carbon 76 220
Kim J M, Kim J H, Lee C Y, Jerng D W and Ahn H S 2018 J. Hazard. Mater. 344 458
Faghihi-Zarandi A, Rakhtshah J, Yarahmadi B B and Shirkhanloo H 2020 J. Environ. Chem. Eng. 8 104193
Ding D, Xue Q, Lu W and Xiong Y 2018 Sens. Actuators B Chem. 259 289
Szczęśniak B, Choma J and Jaroniec M 2018 J. Colloid Interface Sci. 514 801
Pei C C, Kinshinglo K and Leung W W 2017 Purif. Technol. 184 205
Kanjwal M A and Leung W W 2019 Ceram. Int. 45 5617
Trapalis A, Todorova N, Giannakopoulou T and Boukos N 2016 Appl. Catal. B 180 637
Ju K S and Parales R E 2010 Microbiol. Mol. Biol. Rev 74 250
Holliger C, Gaspard S, Glod G and Heijman C 1997 FEMS Microbiol. Rev. 20 517
Tiwari J, Tarale P, Sivanesan S and Bafana A 2019 Environ. Sci. Pollut. Res. 26 28650
Yan F-F, He Y-R, Cheng Y-Y and Li W-W 2014 Environ. Sci. Technol. Lett. 1 128
Wang J, Wang D, Liu G and Lu H 2014 J. Chem. Technol. Biotechnol. 89 750
Tong H, Hu M, Li F B and Chen M J 2014 Soil Biol. Biochem. 70 142
Pinto A M, Gonçalves I C and Magalhaes F D 2013 Colloids Surf. B 111 188
Lanphere J D, Luth C J and Walker S L 2013 Environ. Sci. Technol. 47 4255
Chowdhury I, Duch M C, Manuskhani N D and Bouchard D 2013 Environ. Sci. Technol. 47 6288
Vu H C, Dwivedi A D, Le T T and Chang Y-S 2017 Chem. Eng. J. 307 220
Li M F, Liu Y G, Liu S B and Shu D 2017 Chem. Eng. J. 319 219
Raghubanshi H, Ngobeni S M, Osikoya A O and Dikio C W 2017 J. Ind. Eng. Chem. 47 169
Huang Z-H, Zheng X, Lv W and Wang M 2011 Langmuir 27 7558
Overbeek, T. 1999 Adv. Colloid Interface Sci. 83 IX
Chen Y, Zhang W, Yang S and Wang X 2016 Sci. China Chem. 59 412
Bi Q, Hu Y and Tan P 2015 Industrial Water Wastewater 46 4
Acknowledgements
We are thankful for the financial support of Basic Research Development Program of Taicang of Jiangsu Province, China (TC2021JC17, TC2021JC11, 2021) and the Natural Science Fund for Colleges and Universities in Jiangsu Province (No. 19KJB310017).
Author information
Authors and Affiliations
Corresponding authors
Rights and permissions
About this article
Cite this article
Zhu, Q., Wang, J., Zhang, L. et al. Research progress of graphene for the adsorption, enrichment and analysis of environmental pollutants. Bull Mater Sci 46, 17 (2023). https://doi.org/10.1007/s12034-022-02856-5
Received:
Accepted:
Published:
DOI: https://doi.org/10.1007/s12034-022-02856-5