Abstract
Pantanal is a unique biome located in Brazil, with diverse fauna and flora, being home to native species such as the rice types Oryza latifolia and Oryza rufipogon. Rice is a staple food for two-thirds of the population, with increasing consumption, especially in Asia, regions of Sub-Saharan Africa, countries in the Caribbean, and Latin America. The per capita rice consumption had an average consumption of 160 g/day. However, rice consumption may lead to the intake of other harmful substances to health, such as toxic metals/metalloids. The determination of potential hazards in native species is crucial in maintaining the local population in good health. In this study, we determined the concentration of essential elements and potentially toxic elements in seven different types of Brazilian rice grains, including the two Pantanal native species O. latifolia and O. rufipogon, using ICP-OES to identify their nutritional richness or potential toxicity. The contaminant with the highest HQ levels was chromium, with an HQ above one only in the native species. All species (commercial and native) showed carcinogenic risk considering inorganic arsenic. Rice exhibits duality in its classification, providing nutritional content and leading people to potential risks of overexposure to toxic elements. While rice can be part of a healthy and nutritious diet, more studies should be conducted on avoiding or remedying contamination with toxic elements.
Similar content being viewed by others
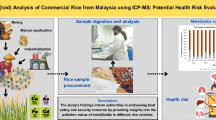
Explore related subjects
Discover the latest articles, news and stories from top researchers in related subjects.Avoid common mistakes on your manuscript.
Introduction
Pantanal is a unique biome located in Brazil’s central-western region, with a proximate area of 150,000 km2 in the Upper Paraguay River Basin, encompassing several environments with open water, ground, perennial, and floating vegetation [1]. Having this multitude of conditions, Pantanal comprises diverse flora, including an area for grassy-woody Savannah (flooded grassland) with coarse grasses and wild species, such as Oryza spp. [2].
Some areas in Pantanal where rice grows are called “arrozais” and are flooded for 7–10 months a year. The deluge depends on floodwaters from the north. The arrozais areas have monodominants stands of Oryza latifolia Desv. (Poaceae) and Oryza rufipogon Griff. with other species, such as Leersia hexandra Sw. and Hymenachne amplexicaulis (Rudge) Nees [3].
Both O. latifolia Desv. (Poaceae) and O. rufipogon Griff are popularly known as “Arroz-do-Pantanal” and “Arroz-do-Campo” and are used in traditional dishes, such as Galinhada (rice and jerk chicken) [4]. This paper will use the synonym “arroz pantaneiro” (in English: Pantaneiro Rice (PR)), which is “Arroz-do-Pantanal” to name these rice species. Some riverside communities in the Amolar region (Brazilian Pantanal) use these species in their diet [5]. Although the biology and phenology of O. latifolia and O. rufipogon in the Pantanal wetland in Mato Grosso do Sul, Brazil, are described [6, 7], there is little information on the composition of macro- and micro-elements in these rice species.
In recent years, studies have been conducted to determine the concentration of metals and non-metals in various rice using inductively coupled plasma-optical emission spectrometry (ICP-OES) [8,9,10,11]. Rice is known to absorb metals from the soil with more efficiency than other plants, and it is a staple food, so its consumption may offer risks, due to the accumulation and potential exposure to these species [12]. It is worth noting that these data are not supported by epidemiological investigation, but since humans cannot eliminate toxic metals efficiently, the intake should be regarded [13].
Although various types of rice are consumed in several countries, monitoring their composition is necessary as it is related to nutrition and toxicity. That is especially true for wild species, where few studies are available determining its safe consumption. The wild species O. latifolia and O. rufipogon were not widely consumed nationwide in Brazil and are objects of projects trying to recover their use by the population of the Indigenous Guató and other riverside communities [14], but they lack information regarding their safety.
The elements calcium (Ca), cobalt (Co), copper (Cu), chromium (Cr), iron (Fe), potassium (K), magnesium (Mg), manganese (Mn), phosphorus (P), selenium (Se), and zinc (Zn) are essentials to health human; however, aluminum (Al), arsenic (As), cadmium (Cd), molybdenum (Mo), and lead (Pb) can be toxic [15,16,17]. Even essential nutrients may be toxic, depending on the dose. It is possible to ensure adequacy for healthy people for most essential elements by following the dietary reference intakes (DRIs) with proposed intake amounts for most of the population, and when available, to avoid toxicity, there are upper consumption limits [21]. For non-essential nutrients, there are other ways to estimate their possible hazard, such as the hazard quotient (HQ) for a particular element and the hazard index (HI), both of which are risk calculations for long-term exposure to noncarcinogens [22], and metal(loid)s and risk associations were performed in Brazilian rice [18,19,20], but we did not find evidence of elemental composition in PR 1 and PR 2.
Therefore, determining the concentration of essential elements and contaminants in rice is crucial to understanding its nutritional values, especially when there is no description of these characteristics, as in the case of PR 1 and PR 2. This study aims to analyze samples of rice from the Pantanal and assess their nutritional significance and consumption risk.
Materials and Methods
Acquisition of Rice Samples
Native samples of O. latifolia and O. rufipogon rice were acquired in April 2019 through the donation of riverside dwellers who live near the city of Corumbá, MS, Brazil, who collected in various sites of the city of Corumbá and donated the grains in a single pool. Samples were identified by Geraldo Damasceno, and there were already deposits in the Herbarium from the Federal University of Mato Grosso do Sul (UFMS) with identification numbers CGMS 19799 and CGMS 36071 for O. latifolia and O. rufipogon, respectively. All other samples were commercially available rice of long-grain polished white rice (LWR), organic short-grain brown rice (OSBR), parboiled long-grain brown rice (LBR), short-grain Black rice (SBR), and short-grain Red rice (SRR) that were purchased from supermarkets in the City of Campo Grande, MS, Brazil, in January 2020. Figure 1 shows the collected (native) and purchased rice.
Rice species (left to right). Native species with popular names: (a) (Oryza latifolia Desv.) Arroz-do-campo, arroz-do-brejo, arroz selvagem, or arroz pantaneiro (pantaneiro rice); (b) (Oryza rufipogon) Arroz-do-campo; purchased species: (Oryza sativa) (c) short-grain black rice, (d) short-grain red rice, (e) long-grain polished white rice, (f) organic short-grain brown rice, and (g) parboiled long-grain brown rice
Microwave-Assisted Digestion and Analysis by ICP-OES
Microwave digestion was used by a modified method used by Kilic [23]. Briefly, proximate 0.25 g of powdered rice was weighted directly into Teflon DAP60™ vessels, and 2 mL of HNO3 (65%, Merck, Darmstadt, Germany) and 1.5 mL of H2O2 (30%, Merck) were added. The rice samples digestion occurred in a microwave system (Speedwave four™, Berghof, Germany) on conditions reported in Table 1. The blank solutions were prepared in the same way as the samples, without adding the rice samples.
After the digestion procedure, the concentrations of Al, As, Ca, Cd, Co, Cr, Cu, Fe, K, Mg, Mn, Mo, P, Pb, Se, and Zn in the rice samples were determined by inductively coupled plasma-optical emission spectrometer (ICP-OES) (iCAP 6300 Series, Thermo Scientific, Cambridge, UK). The operating conditions of ICP-OES for analysis were the following: radiofrequency power of 1150 W, pump flow of 50 rpm, plasma argon flow of 12 L/min, auxiliary argon flow of 0.50 L/min, nebulizer gas flow of 0.70 L/min, and axially viewed observation mode. The wavelength used in the measurements of emission signal for the chosen ICP-OES was as follows: Al 167.079 nm, As 189.042 nm, Ca 393.366 nm, Cd 228.802, Co 228.616 nm, Cr 283.563 nm, Cu 324.754 nm, Fe 259.940 nm, K 766.490 nm, Mg 279.553 nm, Mn 257.610 nm, Mo 202.030, P 177.495 nm, Pb 220.353 nm, Se 196.090 nm, and Zn 213.856 nm.
A multi-element standard solution containing 1000 mg/L of each element for the ICP-OES test was prepared by dilution (SpecSol, Quinlab, Jacarei, SP, Brazil). We used seven different concentrations to build calibration curves for the quantitative analysis of rice. The concentration range for the elements was 0.01–5.0 mg/L.
A recovery test was performed, where the solutions were spiked with 1 ppm of the used elements. The spiking solution was made from a single multi-element stock solution of 1000 ppm. Results from spike and recovery experiments were in the range of 82–115% (Table 2).
The detection limit of the method was determined as 3×B/SD, where SD is the value of the standard deviation as the concentration of the analyte approaches 0, and B is the blank signal. The limit of quantification (LOQ) was defined as 10 ×B/SD. Table 3 contains the detection limits (LOD), quantification (LOQ), and correlation coefficient (R2) for each element quantified in the present study.
We used one-way ANOVA to compare rice varieties and considered a significant difference when the p-value was below 0.05.
Intake Limits
The intake of any food can pose risks when its elemental content exceeds the values determined by DRI, considering the upper tolerable intake level (UL), which is the highest amount of nutrient intake considered safe for the majority of the population. Exceeding this intake limit can be detrimental to health [24].
However, some elements do not have a defined UL. In these cases, certain limits can be used as a simple comparison parameter, such as provisional maximum tolerable daily intake (PTDMI) and provisional tolerable weekly intake (PTWI) [25].
The PTWI for aluminum is 2 mg/kg per week [26], and the PTDMI for copper has been determined as 0.5 mg/kg/day [27]. The PTWI for iron is 700 µg/kg/day, for chromium is 3 µg/kg/day [25], for manganese is 140 µg/kg/day, for nickel is 15 µg/kg/day [26], and for zinc is 0.3 mg/kg/day [25].
Some elements had proposed intake limits but were withdrawn as there was no evidence that the initially proposed levels were truly safe. These elements include 15 µg/kg/week for arsenic, 7 µg/kg/week for cadmium, and 25 µg/kg/week for lead [26].
Risk Calculations
Inorganic Arsenic Calculation
We calculated inorganic arsenic (i-As) by estimating a value of 70% of total arsenic (t-As), in a method described by European Food Safe Authority (EFSA) [28, 29].
Hazard Quotient
The HQ refers to the amount of a substance received at the point of exposure to the estimated daily intake (EDI) concerning a specific toxicological benchmark, known as the reference dose (RfD), which is a low end-point extrapolated either from a non-observed adverse effect level (NOAEL) or lowest observed adverse effect level (LOAEL). HQ values less than one indicate that adverse effects are unlikely to occur and can be considered negligible. HQs greater than one do not show statistical probabilities of harm happening, while a simple statement of whether (and by how much) an exposure concentration exceeds the reference dose [30].
It is possible to estimate the risk through oral ingestion using the HQ, which uses the EDI, inversely proportional to the RfD for oral exposure (Eq. 1):
The EDI in Eq. 1 derives from the calculation presented in Eq. 2, where the EDI is directly proportional to the multiplication of the concentration of the target element Cele (µg/kg or mg/kg, depending on the reference for each element) by the Cali intake (kg/day) of the food, and inversely proportional to the body weight (kg). EDI and HQ calculations are regularly used to identify exposure risk to metals and metalloids [31].
We used the RfD to establish the safe intake in the HQ calculation, employing the following values for each element: As 0.3 µg/kg/day [32], Mo 5 µg/kg/day, Fe 700 µg/kg/day [33], Cr 3 µg/kg/day [34], Mn 140 µg/kg/day [35], Se 5 µg/kg/day [36], and Zn 300 µg/kg/day [37]. In the absence of an RfD, the TWI was used for determining a possible lead HQ: 25 µg/kg/week [38], Cd 2.5 µg/kg/week [39], and Al 1 mg/kg/week [40]. The provisional reference dose (p-RfD) for Co was set at 3 µg/kg/day [41], and the PTDMI used for copper was 0.5 mg/kg/day [27].
The HQ was not calculated for elements without an RfD or other suitable measures for calculating chronic oral toxicity, such as essential elements with low oral toxicity probability (Ca, K, Mg, P). A daily consumption of 160 g of rice was considered, taking into account the average rice consumption [42], and a body weight of 70 kg was considered for adults. For children, we considered the p50 value from WHO child growth standards [43] for children 1 to 5 years for boys (9.6 kg, 12.2 kg, 14.3 kg, 16.3 kg, and 18.3 kg) and girls (8.9 kg, 11.5 kg, 13.9 kg, 16.1 kg, and 18.2 kg) and the intake reported by Toledo et al. [44] for children 1–5 years old (54.9 g, 54 g, 60.6 g, 66.7 g, and 58.2 g, respectively).
Hazard Index
The HI determines the cumulative risk from exposure to different noncarcinogens. In the same way as the HQ, the acceptable cumulative exposure should be below one [45], according to Eq. 3:
Carcinogenic Risk
The carcinogenic risk (CR) was calculated using the incremental lifetime cancer risk (ILCR) for arsenic. The CR is defined by Eq. 4:
where the EDI is determined by the amount of food containing the element ingested and the amount of ingestion per day, such as in the HQ formulae. The SF is the cancer slope factor; briefly, it is an acceptable upper bound estimation of the likelihood of a response per unit intake of a chemical in a lifetime spam [46]. We considered the current slope factor available for heavy metals through oral ingestion, of inorganic arsenic (i-As). For other heavy metals, there were no available slopes considering oral intake [32, 47]. The US EPA classified the contamination levels as very low to CR below 10−6, low if the CR is between 10−6 and 10−5, medium if the CR is between 10−5 and 10−4, high if they are in the range 10−4–10−3, and very high if they are above 10−3 [48].
Nutritional Claims
As a basis for comparison, we used parameters determined by the Food and Drug Administration (FDA) regarding the content and classification of nutritional claims [49] for essential elements with defined recommended dietary allowances (RDA) or adequate intake (AI) [50], where:
-
Foods containing between 10–19% of a specific nutrient can be considered a “good source” or use the terms “contains” or “provides.”
-
Foods containing above 20% of a specific nutrient can be considered “rich in” or use the term “high in.”
-
Foods with more than 100% of a specific nutrient can be considered “high potency.”
Results and Discussion
Determination of Macro- and Micro-elements
Table 4 shows the elemental concentration of macro- and micro-element data obtained from Pantanal and purchased rice varieties.
The results of metal and metalloid detection are presented in Table 4, while Figure 2 illustrates the HQ for the consumption of these rice varieties, based on the analyzed elements. When available, the elemental content found in the studied rice was compared to the limits set by FAO/WHO and Anvisa (Brazilian national regulation) for rice (or cereal) contamination.
The rice with the highest concentration of aluminum was the LBR, while the one with the lowest concentration was the white rice. Silva et al. found average levels of aluminum ranging from 4 to 10 mg/kg in samples of white, parboiled, and brown rice [19], consistent with the findings of this study, except for the parboiled long-grain brown rice, which exhibited a much higher aluminum concentration (28.8 ± 3.7 mg/kg).
All rice samples demonstrated detectable levels of t-As with SRR having the highest concentration (Table 4), but arsenic content did not surpass the FAO/WHO limit for husked or polished i-As or t-As [51]. The average arsenic detection in rice in a study conducted by Shraim et al. was 0.046 mg/kg [53], which was lower than the levels found in all rice samples in this study.
All rice varieties showed higher quantities of calcium (Table 4) compared to those found by Falahi et al., who reported amounts ranging from 5 to 6.4 mg/kg in varieties of brown rice [54]. They also exceeded the levels found by Silva et al., with concentrations between 0 (undetectable) and 69 mg/kg in white, parboiled, and brown rice varieties in Brazil [19].
All rice samples were contaminated with cadmium, with concentrations ranging from 0.016 to 0.032 mg/160g. Shi et al. evaluated cadmium contamination in rice samples in several countries including four continents: Africa, America, Europe, and Asia. Although all samples showed cadmium contamination, the lowest concentration was 4.9 µg/kg and the highest concentration was 3712 µg/kg. The results found in this study would be comparable to the 4th quartile for cadmium concentration for samples in all continents [55]. Neither sample surpassed the FAO/WHO and Anvisa limits set for cadmium in rice [51].
In all samples, cobalt detection levels are below the detection limits. Naseri et al. found concentrations ranging from 0.17 to 0.44 µg/g in rice available in the Shiraz market [56], above our findings.
Only the PR 1 and 2 and parboiled long-grain brown rice showed detectable amounts of chromium in their grains. Shraim et al. detected chromium in 19 samples of rice acquired in South Arabia, with a mean concentration of 0.057 mg/kg ± 0.045 mg/kg [53], less than PR 1 and PR 2 samples.
Rice that had a higher copper content was LBR and SBR, and the lowest concentration was found in PR 2. The copper concentrations in the rice samples were lower than the mean concentration found by Joy et al. with 5.52 mg/kg in brown rice samples and 3.09 mg/kg in white rice samples [57].
The rice with the highest iron content was LBR (Table 4), which is consistent with the findings of Silva et al., who reported higher levels of this mineral in parboiled rice samples compared to white rice [20].
Except for white rice, all other rice varieties (with lower processing levels) found values compatible or higher than those found by Kumar et al. in brown rice samples for iron content, which reported detections ranging from 13.6 to 19.5 mg/kg [58]. Factors such as processing time and grain morphology can affect the final iron concentration in rice grains, where white rice tends to lose a larger amount of iron [59].
The rice samples have potassium in the following descending order: SRR, OSBR, SBR, PR 1, LBR, PR 2, and LWR. Antoine et al. found potassium ranging from 465 to 3097 mg/kg in samples of white, brown, and Jamaican rice, with higher concentrations of potassium in brown rice and lower concentrations in white rice [9]. Although this study found higher potassium concentrations in brown rice samples, there was an even higher concentration in red rice samples.
Magnesium concentration in rice samples ranged from 82 to 336 mg/kg. Silva et al. found magnesium values ranging from 102 to 1243 mg/kg in samples of Brazilian white, brown, and parboiled rice [19], consistent with the findings of this study.
LWR has enough manganese to be considered rich in this nutrient for men and high potency for women. All other rice varieties can be considered high-potency sources of manganese, as they exceed the RDA by 100% in a single portion [49]. Oliveira et al. found manganese variations ranging from 13.6 to 37.7 mg/kg in rice samples cultivated in Southern Brazil [60], which is consistent with the findings in this study. Rice processing can remove more than 50% of the manganese content [61], which may explain the lower quantity of this element in LWR compared to other samples.
The rice with the highest molybdenum concentration were LBR and SBR (Table 4). The values found by Choi et al. for molybdenum in rice samples were much higher than those detected in this study, with 2690 µg/100 g [62]. Zhang et al. reported that over 50% of the elemental variation in molybdenum in rice grains from the Faridpur and Qyiang areas can be explained by the difference in cultivars. The difference in cultivars accounted for 40% of the variation in the Arkansas area and 39% in Texas [63].
LWR and PR 2 had the lowest phosphorus concentration. Reddy et al. obtained phosphorus content in pigmented rice ranging from 449.2 to 966.4 mg/kg in polished rice and 1546.8 to 1843.6 mg/kg in raw rice [64], compatible with the findings of this study.
The amount of lead in LWR is below the detection limit. For the other rice varieties, it decreases in the following order: PR 2 > OSBR and SRR > LBR and SBR > PR 1. The concentration of lead found in the samples is within the range reported by Xie et al. in rice from different varieties in China, ranging from 0.0125 mg/kg to 0.1511 mg/kg [65]. No sample was above the lead maximum concentration allowed for rice (Anvisa) [52] or for cereals (FAO/WHO) limits [51].
The rice with the lowest selenium concentration was LWR. However, even this rice can be considered rich in selenium. All other rice can be considered potent in selenium, as one serving exceeds 100% of the daily selenium recommendations [49]. Except for LWR, all other selenium detections in the rice were higher than the averages found by Joy et al., which were 0.025 mg/kg for brown rice and 0.028 mg/kg for white rice [57].
The zinc values found in this study are slightly higher than those found by Oliveira et al., with 11.7 to 27.5 mg/kg, and concentrations 20% higher in samples of brown rice than in white rice [60].
Intake Risks from Rice Samples
During the life cycle of plants, they act as miners of various elements present in the soil [66], capable of transporting and accumulating potential toxic elements in their edible parts, serving as the main entry point and incorporation into the food chain [67]. Soil contamination resulting from irrigation with different organic and inorganic pollutants can lead to the absorption and accumulation of these pollutants in rice [68], which may lead to health hazards if consumption is above safe limits. Although heavy metals in our study complied with FAO/WHO and Anvisa regulations, Wei et al. argue that rice can be harmful even within the limits set by standards [69]; therefore, other assessments could be carried out to better understand the risks food can pose.
Despite all rice samples showing aluminum contamination, none exceeded HQ of one (Figure 2), and they did not reach the tolerable weekly intake (TWI) value set of 1 mg/kg for a 70 kg adult, indicating the safety of consumption for this element [40]. For children aged 1–5 years, aluminum also demonstrated an HQ below 1, presented in Supplementary information (SI), (Tables S1 to S10) for all rice. Figure 2 displays the HQ values for the analyzed rice consumption regarding each element that has an RfD.
Figure 2 illustrates the HQ associated with the consumption of all studied rice varieties concerning i-As intake for adults, where SRR only surpassed the HQ of one. For children of 5 years old of both sexes, no rice had an HQ above one, although kids have a smaller body weight to food intake ratio, which could harm them more [70], also the intake is less, amending this fact in this case. On the other hand, SRR presented an HQ above one for children 1–4; PR 2 and LBR showed HQ superior to one only for 1-year-old both sexes (SI, Table S1 to S10). Although the samples showed a higher concentration of t-As, i-As is more toxic to humans than t-As [28], so we used it for our calculations regarding risk. The total concentration of arsenic in rice correlates linearly with the arsenic concentration in soil and water. However, the accumulation of arsenic in different parts of the rice plant varies significantly in the following increasing order: grain < straw < husk < root [71]. This relationship may explain why LWR shows the lowest arsenic content, as the processing of polished white rice removes other parts [72].
All rice samples are contaminated with cadmium, which is a non-essential metal; the cadmium content found in one serving is below the tolerable weekly intake levels proposed by the European Food Safety Authority (EFSA) of 2.5 µg/kg/week [39]. They also do not exceed the HQ of one for adults (Figure 2) or children aged 1–5 (SI, Tables S1 to S10). The cadmium contamination values also fall below the PTWI values of 7 µg/kg/week for this element [26], making the consumption of the rice samples unlikely to cause cadmium toxicity. The concentrations of cadmium found are much higher than those described by Shi et al., with an average of 12.2 µg/kg [55].
Both native Pantanal rice varieties exhibited chromium levels with an HQ above one, considering the intake of a rice serving (Figure 2) for adults; in the same way, HQ calculations considering children 1–5 years from both sexes ranged from 1.53 (5-year-old boys) to 7.27 (1-year-old girls) (SI, Tables S10 and S1, respectively), making their chronic use potentially risky [34]. The high concentration of chromium found in PR 1 and PR 2 is comparable to that found in medium-grain brown rice from Bangladesh, with 0.909 mg/kg in a study conducted by Shraim, but higher than other varieties studied by the authors, with an average of 0.057 mg/kg [53].
None of the rice varieties exceeds the copper UL of 10 mg/day (for adults of both sexes) taking into account the ingestion of one serving [73]. They also did not surpass the PTDMI of 0.5 mg/kg/day [27]. In the same way, all rice samples have a hazard quotient below one (Figure 2) for adults and children from ages 1 to 5 (SI, Tables S1 to S10), indicating a low risk in their chronic consumption considering this element only.
None of the rice samples exceeded an HQ of one (Figure 2) for iron content for adults or children from ages 1–5 years (SI, Tables S1 to S10), or the UL of 45 mg/day [74], or the PTDMI of 700 µg/kg/day considering consumption by a 70 kg adult. Based on this data, it can be inferred that the risk of iron toxicity from the daily consumption of any of the rice samples is unlikely.
As of now, there is no established UL for potassium, as there is not sufficient evidence for potassium toxicity through dietary means in healthy individuals [75]. Similarly, there is no reference dose for this element.
So far, there is no RfD established for magnesium toxicity calculations, and the UL of 350 mg applies for supplementation only, not accounting for dietary sources, as the body tends to eliminate excess magnesium through renal excretion [24]. Therefore, regardless of the high concentrations of magnesium in the studied rice, the chances of intoxication by this element are low.
Despite the high concentration of manganese in rice samples, the HQ for any sample does not exceed one for adults (Figure 2) (SI, Tables S1 to S10), indicating a low likelihood that long-term daily ingestion could cause toxicity. Additionally, no rice exceeds the manganese UL (tolerable upper intake level) of 11 mg/day [24] for the consumption of a single serving, supporting the indications from the HQ.
Regarding the HQ for molybdenum, no sample showed potential for long-term toxicity from consuming one serving of rice per day, as all HQ values were below one (Figure 2) for molybdenum content in adults and children 1 to 5 years (SI, Tables S1 to S10). No rice exceeded the equivalent concentration to the molybdenum UL (tolerable upper intake level) of 2 mg/day [24], supporting the HQ values.
The daily consumption of any of the rice varieties does not exceed the proposed PTWI values of 25 µg/kg/week for lead. These values have been withdrawn as they are no longer considered safe [26]. Similarly, none of the rice exceeded an HQ of one (Figure 2) for adults and children aged 1–5 years (SI, Tables S1 to S10). Even with this information, it is not possible to assert that the lead content in the samples is safe for long-term consumption, as safe limits have not yet been established.
Even with high selenium concentrations, none of the samples exceeded the UL of 400 µg/day. Similarly, no sample surpassed the HQ (Figure 2) for adults and kids 1–5 years old (SI, Tables S1 to S10). Considering that selenium is an essential nutrient for human health [50] and that the doses do not exceed proposed intake limits, the studied rice can be considered a good source of this nutrient with a low risk of toxicity.
The UL determined for zinc is 40 mg/day [24], much higher than the amount found in a serving of any of the samples. None of the samples exceeded the HQ of one (Figure 2) considering adults and children with ages ranging from 1 to 5 years (SI, Tables S1 to S10), reaffirming that the likelihood of causing health damage due to excess zinc from the studied rice is unlikely.
While HQ values are useful when there is one main contaminant, the HI takes into account several sources of contamination and can be helpful to determine risk when different elements build up to a probable toxic level. Figure 3 shows the HI for rice samples.
The HI calculates the potential hazard through all metals and metalloid ingestion, being the HQ sum of all metal(loid)s examined. Again, for PR 1 and PR 2, the element contributing the most to noncarcinogenic risk was chromium, while for other rice, arsenic was the major contaminant (Figure 3). PR 1, PR 2, and SRR had HI values superior to one, showing that cumulative hazard can be computed for all those species for the adult population and for children aged 1–4 years (SI, Tables S1 to S8), while SRR did not pose an HI above one for children aged 5 years from both sexes (SI, Tables S9 and S10). Zeng et al. found HI up to 14.6 for samples of brown rice in China, evaluating the metals Cd, Cr, As, Ni, Pb, Mn, and Hg, far superior to any rice variety in our study [76]. The milling and dehusking process may end up reducing mineral content in rice (both toxic and essential elements) once it removes the bran [77], which may explain why both native species had higher HI, once both samples were not dehulled or participated in any processing, and why other rice with less processing accumulated more metal and metalloids than LWR. Considering that several rice varieties have an HI superior to one, strategies to reduce contamination should be advised and intake amounts limited to pursue safe chronic intakes.
Elemental Content Mitigation in Rice
Elements that are not essential and elements that are essential but are present at concentrations beyond tolerable could pose health hazards [16, 17]. This section will focus on topics for elements with elevated content in this study or non-essential elements present in the samples.
Shariatifar et al. evaluated different cooking methods in impacting the essential and toxic elements content in rice marketed in Iran, where they used two cooking methods to prepare 200 g of rice: the first one “Rinse Cooking Method” (RCM), the rice was brought to ebullition in 1 L of distilled water for 15 min, the extra excess water was eliminated, and then the rice was placed into a new container until done cooking. The other method, the “Traditional Cooking Method” (TCM), consisted of bringing the rice added with 400 mL to a boiling point, reducing the heat, and cooking the rice for another hour at 105 °C. Both methods were compared to determine the final elemental concentration, where aluminum, arsenic, and cadmium presented smaller amounts using the RCM, while chromium and lead showed minor amounts in the TCM [78]. In the same way, Sharafi et al. tested the effects of washing, soaking (1 h, 5 h, and 12 h), and two methods for rice cooking, namely “Kateh”(2:1 water:rice ratio) and “Rinsed” (4:1 water:rice ratio, and disposing of the extra water after cooking). They determined that the combination of washing, soaking for 12 h, and cooking with the Rinsed method was more efficient in removing toxic metals (As, Cd, and Pb), rather than essential metals (Fe, Cu, Co, and Zn) [79]. While considering rice to water ratio and washing times, Shahriar et al. investigated cooking rice with different proportions of distilled water and rice (2:1, 3:1, 4:1, and 6:1 water:rice ratio) and two methods of washing it (3 times and 5 times) and how they influence the final concentration of toxic metals (As, Cd, and Pb) in rice marketed in Bangladesh, determining that the best method to mitigate toxic elements was washing the rice 5 times and keeping a 6:1 rice:water ratio [80]. Therefore, these methods could improve toxicity levels for the studied rice regarding As, Cd, and Pb content.
On the other hand, some elements are not efficiently removed in washing, soaking, and cooking of rice, as in the case of chromium, where rinsing cooked rice led to an increase in chromium concentration [78]. In this case, the contamination by chromium might be prevented at the sowing stage through soil remediation. The application of ferrous oxide covered with modified hairs level 2—according to the methodology of Ullah et al.—reduced the amount of chromium by 79% compared to the control in rice grain samples [81]. However, this option is not viable for native species. Therefore, the safest way to consume native rice varieties with high chromium concentrations is to control portions and intake frequency, which is a valid approach for any element.
Carcinogenic Risk
The carcinogenic risk was estimated considering the consumption of i-As from rice (Table 5).
Considering the CR for i-As exposure, no rice type had a risk below 10−6, which is considered a very low risk for a single-element assessment [48]. Besides, the rice LWR, PR 2, LBR, and SRR presented medium CR for all ages. In the same way, Freire et al. [18] related CR risk for rice cultivated in Brazil. The CR was higher for children than adults, which may be expected, once children and toddlers are more susceptible to contaminants’ presence, due to their immature development and the ratio between intake and body weight is higher [70].
Conclusions
The elemental concentration was determined for different types of rice: LWR, PR 1, PR 2, OSBR, LBR, short-grain black, and SRR.
Considering nutritional intake recommendations for a single serving, no rice was able to significantly contribute to meeting the nutritional recommendations for calcium and cobalt. However, all rice samples can be considered at least a source of the minerals magnesium and molybdenum. In the same way, all kinds of rice are rich in the elements manganese, phosphorus, and selenium. This demonstrates that rice, as a staple food, can contribute to achieving an adequate intake of essential nutrients. All rice showed high potency for at least one nutrient, where manganese and selenium exceeded 100% of the nutritional recommendations for all samples, but white rice.
Taking individual elements into account, chromium was the main contaminant, adding up an HQ above one for two samples—PR 1 and PR 2. The principal way to avoid chromium through rice ingestion should be limiting its intake, once the element cannot be effectively removed from rice in the cooking stage. In SRR, the HQ surpassed one for arsenic, which can be reduced in samples through washing and rinsing techniques before and after preparation.
The HI reflects the potential hazard for all elements; PR 1, PR 2, and SRR surpassed the HI of one, showing possible risks for the long-term intake of these rice, where chromium was the major contaminant for PR 1 and PR 2, and arsenic the principal contaminant for the other samples; also regarding i-As contamination, all rice showed low or medium CR.
Thus, rice, being a staple food, has a duality: providing nutritional content and exposing humans to potential risks of overexposure to toxic elements. While it can be part of a healthy and nutritious diet, more studies should be conducted on the possibility of preventing or remediating contamination with toxic elements.
Data Availability
Data material is available within the text; raw data is available upon request.
References
Silva VO, de Mello CR, Chou SC, et al (2024) Characteristics of extreme meteorological droughts over the Brazilian Pantanal throughout the 21st century. Front Water 6:. https://doi.org/10.3389/frwa.2024.1385077
Pott A, Oliveira AKM, Damasceno-Junior GA, Silva JSV (2011) Plant diversity of the Pantanal wetland. Braz J Biol 71:265–273. https://doi.org/10.1590/S1519-69842011000200005
Bolzan FP, Pereira GMF, Tomas WM et al (2021) Monetary value of the ecosystem services of the Pantanal and its surroundings: first approximations and perspectives. In: Damasceno-Junior GA, Pott A (eds) Flora and vegetation of the Pantanal wetland. Springer International Publishing, Cham, pp 767–783
Bortolotto IM, Hiane PA, Ishii IH et al (2017) A knowledge network to promote the use and valorization of wild food plants in the Pantanal and Cerrado, Brazil. Reg Environ Change 17:1329–1341. https://doi.org/10.1007/s10113-016-1088-y
Bortolotto I, Guarim Neto G (1998) Etnobotânica e Conservação da Natureza em uma Escola Rural do Distrito de Albuquerque-Corumbá-MS: Uma abordagem para a Educação. Cuiabá. Revista de Educação Pública 7:
Bertazzoni EC, Damasceno-Júnior GA (2011) Aspectos da biologia e fenologia de Oryza latifolia Desv. (Poaceae) no Pantanal sul-mato-grossense. Acta Bot Bras 25:476–786. https://doi.org/10.1590/S0102-33062011000200023
Leandro TD, Manvailer V, de Oliveira AR, do C, Scremin-Dias E, (2022) Pantanal flood pulse reveals constitutive and plastic features of two wild rice species (Poaceae, Oryzoideae): implications for taxonomy, systematics, and phylogenetics. Braz J Bot 45:1261–1278. https://doi.org/10.1007/s40415-022-00835-y
Sneddon EJ, Hardaway CJ, Sneddon J et al (2017) Determination of selected metals in rice and cereal by inductively coupled plasma-optical emission spectrometry (ICP-OES). Microchem J 134:9–12. https://doi.org/10.1016/j.microc.2017.04.009
Antoine JMR, Hoo Fung LA, Grant CN et al (2012) Dietary intake of minerals and trace elements in rice on the Jamaican market. J Food Compos Anal 26:111–121. https://doi.org/10.1016/j.jfca.2012.01.003
Oliveira A, Baccan N, Cadore S (2012) Evaluation of metal ions in rice samples: extraction and direct determination by ICP OES. J Braz Chem Soc 23:838–845. https://doi.org/10.1590/S0103-50532012000500008
Ebrahimi-Najafabadi H, Pasdaran A, Rezaei Bezenjani R, Bozorgzadeh E (2019) Determination of toxic heavy metals in rice samples using ultrasound assisted emulsification microextraction combined with inductively coupled plasma optical emission spectroscopy. Food Chem 289:26–32. https://doi.org/10.1016/j.foodchem.2019.03.046
Zakaria Z, Zulkafflee NS, Mohd Redzuan NA et al (2021) Understanding potential heavy metal contamination, absorption, translocation and accumulation in rice and human health risks. Plants 10:1070. https://doi.org/10.3390/plants10061070
Jallad KN (2015) Heavy metal exposure from ingesting rice and its related potential hazardous health risks to humans. Environ Sci Pollut Res 22:15449–15458. https://doi.org/10.1007/s11356-015-4753-7
Bortolotto I (2017) Conservação da biodiversidade, alimentos e cultura em Mato Grosso do Sul. Campo Grande: Editora UFMS
Drugs M, Agents M-BD (2005) The use of metals in medicine. Eds M Gielen, ERT Tiekink NY: John Wiley and Sons
Skalnaya MG, Skalny AV (2018) Essential trace elements in human health: a physician’s view. Tomsk: Publishing House of Tomsk State University 224:1–222
Saha SK, Pathak NN (2021) Mineral Nutrition. In: Saha SK, Pathak NN (eds) Fundamentals of animal nutrition. Springer, Singapore, pp 113–131
Freire BM, Paniz FP, Lange CN et al (2023) Effect of water management on human exposure to inorganic arsenic and other trace elements through rice consumption. J Food Compos Anal 122:105462. https://doi.org/10.1016/j.jfca.2023.105462
da Silva IJS, Paim APS, da Silva MJ (2018) Composition and estimate of daily mineral intake from samples of Brazilian rice. Microchem J 137:131–138. https://doi.org/10.1016/j.microc.2017.10.006
da Silva DG, Scarminio IS, Anunciação DS et al (2013) Determination of the mineral composition of Brazilian rice and evaluation using chemometric techniques. Anal Methods 5:998–1003. https://doi.org/10.1039/C2AY26158H
NIH Office of Dietary Supplements -. https://ods.od.nih.gov/HealthInformation/nutrientrecommendations.aspx. Accessed 9 Apr 2024
Bleam WF (2012) Hazard quotient - an overview | ScienceDirect Topics. https://www.sciencedirect.com/topics/food-science/hazard-quotient. Accessed 21 Mar 2024
Kilic S (2024) Determination of potentially toxic elements and health risk assessment of dried fruits. J Food Sci Technol 61:397–402. https://doi.org/10.1007/s13197-023-05849-2
National Academies of Sciences E, Oria M, Harrison M, Stallings VA (2019) Dietary reference intakes (DRIs): tolerable upper intake levels, elements, Food and Nutrition Board, National Academies. https://www.ncbi.nlm.nih.gov/books/NBK545442/table/appJ_tab9/. Accessed 5 Oct 2020
WHO; JECFA (1983) Evaluations of the Joint FAO/WHO Expert Committee on Food Additives (JECFA). https://apps.who.int/food-additives-contaminants-jecfa-database/chemical.aspx?chemID=2859. Accessed 6 Oct 2020
WHO; JECFA (2011) Evaluations of the Joint FAO/WHO Expert Committee on Food Additives (JECFA). https://apps.who.int/food-additives-contaminants-jecfa-database/chemical.aspx?chemID=298. Accessed 6 Oct 2020
WHO; JECFA (2019) Evaluations of the Joint FAO/WHO Expert Committee on Food Additives (JECFA). https://apps.who.int/food-additives-contaminants-jecfa-database/chemical.aspx?chemID=2824. Accessed 6 Oct 2020
Authority EFS (2014) Dietary exposure to inorganic arsenic in the European population. EFSA J 12:3597. https://doi.org/10.2903/j.efsa.2014.3597
Sigrist M, Hilbe N, Brusa L et al (2016) Total arsenic in selected food samples from Argentina: estimation of their contribution to inorganic arsenic dietary intake. Food Chem 210:96–101. https://doi.org/10.1016/j.foodchem.2016.04.072
Pham LL, Borghoff SJ, Thompson CM (2020) Comparison of threshold of toxicological concern (TTC) values to oral reference dose (RfD) values. Regul Toxicol Pharmacol 113:104651. https://doi.org/10.1016/j.yrtph.2020.104651
Werdemberg dos Santos LC, Granja Arakaki D, de Pádua S, Melo E, Nascimento VA (2022) Health hazard assessment due to slimming medicinal plant intake. Biol Trace Elem Res 200:1442–1454. https://doi.org/10.1007/s12011-021-02732-z
US EPA National Center for Environmental (1991) Arsenic, inorganic CASRN 7440–38–2 | IRIS | US EPA, ORD. https://iris.epa.gov/ChemicalLanding/&substance_nmbr=278. Accessed 8 Feb 2024
US EPA (2006) Iron and compounds. https://cfpub.epa.gov/ncea/pprtv/chemicalLanding.cfm?pprtv_sub_id=1773. Accessed 8 Feb 2024
US EPA O (1998) Chromium(VI) CASRN 18540–29–9 | DTXSID7023982 | IRIS | US EPA, ORD. https://cfpub.epa.gov/ncea/iris2/chemicallanding.cfm?substance_nmbr=144. Accessed 8 Feb 2024
US EPA O (1995) Manganese CASRN 7439–96–5 | DTXSID2024169 | IRIS | US EPA, ORD. https://cfpub.epa.gov/ncea/iris2/chemicallanding.cfm?substance_nmbr=373. Accessed 8 Feb 2024
US EPA O (1991) Selenium and compounds CASRN 7782–49–2 | DTXSID9021261 | IRIS | US EPA, ORD. https://cfpub.epa.gov/ncea/iris2/chemicalLanding.cfm?substance_nmbr=472. Accessed 8 Feb 2024
US EPA O (2005) Zinc and compounds CASRN 7440–66–6 | DTXSID7035012 | IRIS | US EPA, ORD. https://cfpub.epa.gov/ncea/iris2/chemicallanding.cfm?substance_nmbr=426. Accessed 8 Feb 2024
Chain (CONTAM) EP on C in the F (2010) Scientific opinion on lead in food. EFSA J 8:1570 https://doi.org/10.2903/j.efsa.2010.1570
Chain (CONTAM) EP on C in the F (2011) Statement on tolerable weekly intake for cadmium. EFSA J 9:1975 https://doi.org/10.2903/j.efsa.2011.1975
EFSA (2008) Safety of aluminium from dietary intake - scientific opinion of the panel on food additives, flavourings, processing aids and food contact materials (AFC). EFSA J 6:754. https://doi.org/10.2903/j.efsa.2008.754
US EPA (2008) Cobalt. https://cfpub.epa.gov/ncea/pprtv/chemicalLanding.cfm?pprtv_sub_id=1655. Accessed 8 Feb 2024
OECD (2015) Rice projections: consumption, per capita. Organisation for Economic Co-operation and Development, Paris
WHO (2009) WHO Child growth standards. https://www.who.int/tools/child-growth-standards/standards/weight-for-age. Accessed 7 Jun 2024
Toledo MC, Lee JS, Batista BL et al (2022) Exposure to inorganic arsenic in rice in Brazil: a human health risk assessment. Int J Environ Res Public Health 19:16460. https://doi.org/10.3390/ijerph192416460
Bleam WF (2012) Chapter 10 - Risk assessment. In: Bleam WF (ed) Soil and environmental chemistry. Academic Press, Boston, pp 409–447
Baynes RE (2012) Chapter Nine - Quantitative risk assessment methods for cancer and noncancer effects. In: Hodgson E (ed) Progress in molecular biology and translational science. Academic Press, pp 259–283
US EPA (2007) Slope Factors (SF) from US EPA. http://www.popstoolkit.com/tools/HHRA/SF_USEPA.aspx. Accessed 7 Jun 2024
US-EPA. (1999) A risk assessment–multi way exposure spread sheet calculation tool
FDA (2018) Nutrient content claims. In: FDA Reader. https://www.fdareader.com/blog/2018/12/13/product-claims. Accessed 9 Feb 2024
National Academies of Sciences E, Oria M, Harrison M, Stallings VA (2019) Dietary reference intakes (DRIs): recommended dietary allowances and adequate intakes, elements, Food and Nutrition Board, National Academies. https://www.ncbi.nlm.nih.gov/books/NBK545442/table/appJ_tab3/. Accessed 17 Oct 2020
FAO/WHO (2023) Contaminants | CODEX ALIMENTARIUS. https://www.fao.org/fao-who-codexalimentarius/thematic-areas/contaminants/en/. Accessed 5 Jun 2024
BRASIL, Anvisa (2022) Instrução Normativa, n 160. https://antigo.anvisa.gov.br/documents/10181/2718376/IN_160_2022_.pdf. Accessed 7 Jun 2024
Shraim AM (2017) Rice is a potential dietary source of not only arsenic but also other toxic elements like lead and chromium. Arab J Chem 10:S3434–S3443. https://doi.org/10.1016/j.arabjc.2014.02.004
Falahi E, Hedaiati R, Ghiasvand AR (2010) Survey of iron, zinc, calcium, copper, lead, and cadmium in rice samples grown in Iran. Food Addit Contam : Part B 3:80–83. https://doi.org/10.1080/19440041003671288
Shi Z, Carey M, Meharg C et al (2020) Rice grain cadmium concentrations in the global supply-chain. Expo Health 12:869–876. https://doi.org/10.1007/s12403-020-00349-6
Naseri M, Vazirzadeh A, Kazemi R, Zaheri F (2015) Concentration of some heavy metals in rice types available in Shiraz market and human health risk assessment. Food Chem 175:243–248. https://doi.org/10.1016/j.foodchem.2014.11.109
Joy EJM, Louise Ander E, Broadley MR et al (2017) Elemental composition of Malawian rice. Environ Geochem Health 39:835–845. https://doi.org/10.1007/s10653-016-9854-9
Kumar A, Lal MK, Kar SS et al (2017) Bioavailability of iron and zinc as affected by phytic acid content in rice grain. J Food Biochem 41:e12413. https://doi.org/10.1111/jfbc.12413
Prom-u-thai C, Sanchai C, Rerkasem B et al (2007) Effect of grain morphology on degree of milling and iron loss in rice. Cereal Chem 84:384–388. https://doi.org/10.1094/CCHEM-84-4-0384
de Oliveira VF, Busanello C, Viana VE et al (2021) Assessing mineral and toxic elements content in rice grains grown in southern Brazil. J Food Compos Anal 100:103914. https://doi.org/10.1016/j.jfca.2021.103914
Yao B-M, Chen P, Sun G-X (2020) Distribution of elements and their correlation in bran, polished rice, and whole grain. Food Sci Nutr 8:982–992. https://doi.org/10.1002/fsn3.1379
Choi M-K, Kang M-H, Kim M-H (2009) The analysis of copper, selenium, and molybdenum contents in frequently consumed foods and an estimation of their daily intake in Korean adults. Biol Trace Elem Res 128:104–117. https://doi.org/10.1007/s12011-008-8260-2
Zhang H, Jang S-G, Lar SM et al (2021) Genome-wide identification and genetic variations of the starch synthase gene family in rice. Plants 10:1154. https://doi.org/10.3390/plants10061154
Reddy CK, Kimi L, Haripriya S, Kang N (2017) Effects of polishing on proximate composition, physico- chemical characteristics, mineral composition and antioxidant properties of pigmented rice. Rice Sci 24:241–252. https://doi.org/10.1016/j.rsci.2017.05.002
Xie LH, Tang SQ, Wei XJ et al (2017) The cadmium and lead content of the grain produced by leading Chinese rice cultivars. Food Chem 217:217–224. https://doi.org/10.1016/j.foodchem.2016.08.099
Peralta-Videa JR, Lopez ML, Narayan M et al (2009) The biochemistry of environmental heavy metal uptake by plants: Implications for the food chain. Int J Biochem Cell Biol 41:1665–1677. https://doi.org/10.1016/j.biocel.2009.03.005
Fu J, Zhou Q, Liu J et al (2008) High levels of heavy metals in rice (Oryzasativa L.) from a typical E-waste recycling area in southeast China and its potential risk to human health. Chemosphere 71:1269–1275. https://doi.org/10.1016/j.chemosphere.2007.11.065
Satpathy D, Reddy MV, Dhal SP (2014) Risk assessment of heavy metals contamination in paddy soil, plants, and grains (Oryza sativa L) at the East Coast of India. BioMed Research International 2014:e545473. https://doi.org/10.1155/2014/545473
Wei R, Chen C, Kou M et al (2023) Heavy metal concentrations in rice that meet safety standards can still pose a risk to human health. Commun Earth Environ 4:1–9. https://doi.org/10.1038/s43247-023-00723-7
de Paiva EL, Morgano MA, Arisseto-Bragotto AP (2019) Occurrence and determination of inorganic contaminants in baby food and infant formula. Curr Opin Food Sci 30:60–66. https://doi.org/10.1016/j.cofs.2019.05.006
Abedin MdJ, Cresser MS, Meharg AA et al (2002) Arsenic accumulation and metabolism in rice (Oryza sativa L.). Environ Sci Technol 36:962–968. https://doi.org/10.1021/es0101678
Runge J, Heringer OA, Ribeiro JS, Biazati LB (2019) Multi-element rice grains analysis by ICP OES and classification by processing types. Food Chem 271:419–424. https://doi.org/10.1016/j.foodchem.2018.07.162
NIH (2022) Office of dietary supplements - copper. https://ods.od.nih.gov/factsheets/Copper-HealthProfessional/. Accessed 9 Feb 2024
Micronutrients I of M (US) P on (2001) Iron. In: Dietary reference intakes for vitamin A, vitamin K, arsenic, boron, chromium, copper, iodine, iron, manganese, molybdenum, nickel, silicon, vanadium, and zinc. National Academies Press (US)
National Academies of Sciences (2019) Dietary reference intakes for sodium and potassium. National Academies Press (US), Washington (DC)
Zeng F, Wei W, Li M et al (2015) Heavy metal contamination in rice-producing soils of Hunan Province, China and potential health risks. Int J Environ Res Public Health 12:15584–15593. https://doi.org/10.3390/ijerph121215005
Lamberts L, De Bie E, Vandeputte GE et al (2007) Effect of milling on colour and nutritional properties of rice. Food Chem 100:1496–1503. https://doi.org/10.1016/j.foodchem.2005.11.042
Shariatifar N, Rezaei M, Alizadeh Sani M et al (2020) Assessment of rice marketed in iran with emphasis on toxic and essential elements; effect of different cooking methods. Biol Trace Elem Res 198:721–731. https://doi.org/10.1007/s12011-020-02110-1
Sharafi K, Yunesian M, Mahvi AH et al (2019) Advantages and disadvantages of different pre-cooking and cooking methods in removal of essential and toxic metals from various rice types- human health risk assessment in Tehran households. Iran Ecotoxicol Environ Safety 175:128–137. https://doi.org/10.1016/j.ecoenv.2019.03.056
Shahriar S, Paul AK, Rahman MM (2022) Removal of toxic and essential nutrient elements from commercial rice brands using different washing and cooking practices: human health risk assessment. Int J Environ Res Public Health 19:2582. https://doi.org/10.3390/ijerph19052582
Ullah A, Ma Y, Li J et al (2020) Effective amendments on cadmium, arsenic, chromium and lead contaminated paddy soil for rice safety. Agronomy 10:359. https://doi.org/10.3390/agronomy10030359
Funding
This research was supported by the National Council for Scientific and Technological Development (CNPq), Brazil. This study was financed in part by the Coordenação de Aperfeiçoamento de Pessoal de Nível Superior—Brasil—(CAPES)—Finance Code 001.
Author information
Authors and Affiliations
Contributions
Conceptualization: V.A.N. and R.R.; methodology: E.M. L.L, H.P, K.R.N.S.; formal analysis: D.G.A.; investigation: R.R., D.G.A., and V.A.N.; resources: R.C.A.G., K.C.F., P.A.H., D.B., and G.D.; images curation: A.S.A.J.; data curation: A.S.A.J., R.R., V.A.N.; writing—original draft preparation: R.R.; writing—review and editing: D.G.A. and V.A.N.; supervision: V.A.N.; all authors have read and agreed to the published version of the manuscript.
Corresponding author
Ethics declarations
Conflict of Interest
The authors declare no competing interests.
Additional information
Publisher's Note
Springer Nature remains neutral with regard to jurisdictional claims in published maps and institutional affiliations.
Supplementary Information
Below is the link to the electronic supplementary material.
Rights and permissions
Springer Nature or its licensor (e.g. a society or other partner) holds exclusive rights to this article under a publishing agreement with the author(s) or other rightsholder(s); author self-archiving of the accepted manuscript version of this article is solely governed by the terms of such publishing agreement and applicable law.
About this article
Cite this article
Rosa, R., Granja Arakaki , D., Melo, E. et al. Determination of Selected Metals and Metalloids in Different Types of Rice by Inductively Coupled Plasma-Optical Emission Spectrometry (ICP-OES). Biol Trace Elem Res (2024). https://doi.org/10.1007/s12011-024-04284-4
Received:
Accepted:
Published:
DOI: https://doi.org/10.1007/s12011-024-04284-4