Abstract
Traditional processing practices used in the manufacture of ready-to-eat edible crab products include a double-heat treatment involving an initial cooking step followed by washing and packaging and finally, a second heat pasteurisation. The latter, pasteurisation step, results in the most severe impact on product quality. The main objective of this research was to optimise this pasteurisation step using quality index degradation kinetic approach. Preliminary work involved the characterisation of temperature rise in the crab cold-spot during pasteurisation. Equivalent treatments (F90°C10°C = 10 min) were defined in order to assess the impact of pasteurisation temperature on different crab quality indexes in both crab meat types, white and brown. Colour degradation of crab white meat was defined as the critical quality parameter to be monitored during thermal pasteurisation. The effect of time and temperature on the kinetics of white meat colour change (ΔE*) were characterised and fitted to an exponential equation. Following this, an industry focus group was used to define white meat colour change vs product quality and defined ‘good’ (ΔE* ≤ 7), ‘acceptable’ (7 < ΔE* < 9) and ‘unacceptable’ (ΔE* ≥ 9) quality. Finally, using the developed equations, optimal pasteurisation conditions were defined and validated. To produce ‘good’ quality crab, optimal temperatures ranged between 96 and 100 °C while temperatures between 104 and 108 °C produced ‘acceptable’ quality in crabs of 400 and 800 g, respectively. Overall, the results show that the equations obtained could be used in a decision support system (DSS) to define heat pasteurisation conditions to optimise the quality of ready-to-eat edible crab.
Similar content being viewed by others
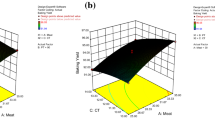
Avoid common mistakes on your manuscript.
Introduction
Thermal processing is the most commonly used food industry technology to produce microbiologically safe and shelf-stable foodstuffs. However, when it comes to solid foods, heat treatments generally require long times, which in turn may promote undesirable quality changes such as the development of off-odours, off-flavours, nutrient degradation and colour changes (Ling et al. 2015; Liaotrakoon et al. 2013; Zabbia et al., 2012). The selection of effective heat-processing conditions that produce adequate microbial inactivation while also minimising undesirable heat-related changes is important for the food industry. A kinetic model development approach is a possible solution towards solving this challenge. Such an approach can provide valuable information to predict and control product quality during thermal processing (Haefner 2005). The effect of heat treatments on the inactivation kinetics of microorganisms and enzymes have been extensively reported in the literature and different modelling equations have been proposed (FDA 2000; IFT, 2013). These models are widely accepted and used in the food industry to define the minimum required processing conditions to produce safe products. Some other studies have reported the effect of heat processing on the degradation kinetics of different quality attributes such as colour, nutrients and texture (Fante and Noreña 2012; Hadjal et al. 2013; Jaiswal et al. 2012; Yu et al. 2011). However, these models have to be developed for each individual food product due to the complexity of the reactions involved. Additionally, most of these studies have been performed in plant-based products. Relatively, a few of those have been performed on fish or seafood products (Kong et al. 2007; Ovissipour et al. 2013).
Crustaceans are highly appreciated worldwide with many crustacean products considered as a healthy choice for their high-quality protein, amino acid composition (Gökoðlu and Yerlikaya 2003; Maulvault et al. 2012) and low content of saturated fatty acids (Barrento et al. 2009; Barrento et al. 2008). Edible crab (Cancer pagurus) is highly valued and consumed in southern Europe and its market is expanding to other continents where consumption of processed ready-to-eat crab products is also increasing (Eurostat 2015). Although the market for this crustacean is still dominated by live crabs, this commercialization procedure often leads to significant losses, in some cases up to 50% of the products. By contrast, exporting crabs in a prepared, ready-to-eat format avoids the losses associated with transport and adds value to the final product (Barrento et al. 2008; Barrento et al. 2010; Uglow et al. 1986). The production of ready-to-eat whole edible crabs involves an initial cooking step followed by a washing/cooling step, then packaging and a second in-pack thermal treatment step (pasteurisation) to ensure microbiological safety and shelf-life (Edwards and Early 2001). This second in-pack pasteurisation is designed to inactivate the bacterial spores that may be present in these products. This step’s target is a 6 Log10 cycle reduction of Clostridium botulinum non-proteolytic type E (FDA 2011; Huss 1997; Linton et al. 2003). However, the intense heat treatment required to obtain this objective can result in undesirable changes in the final product if the heating process is not adequately designed and controlled.
The impact of this second pasteurisation step on the quality characteristics of edible crab has not been previously studied. Therefore, the main objectives of this research were: (1) to characterise the thermal profiles of edible crab in a pilot plant scale retort; (2) to evaluate the main quality attributes of edible crab affected by the thermal treatment; (3) to define the heat degradation kinetics of the most affected quality attribute under controlled conditions; and (4) to develop suitable equations to allow the definition of the optimal thermal pasteurisation conditions for ready-to-eat whole edible crab.
Material and Methods
Raw Materials and Crab Preparation
All experiments were carried out using female North Atlantic crabs (400–900 g), obtained from a local supplier in Zaragoza (Spain). Crabs were sacrificed by the supplier on the collection and immediately stored in frozen conditions at − 20 °C until required for analysis (maximum 30 days). Prior to use, crabs were defrosted in a cold room at 4 °C for 24 h and then weighed. Once defrosted, crabs were cooked in a thermostatic bath (CC-E, Huber, Germany) containing water with 2% w/v salt, at 95–98 °C for 20 min as recommended by the Irish crab industry (Edwards and Early 2001). After cooking, crabs were cooled in ice-water until a temperature below 20 °C was achieved in the cold-spot which was located in the crab’s abdomen as we demonstrated in a previous study (Condon-Abanto et al. 2018). Temperature was measured using a K-type thermocouple (Ahlborn, Holzkirchen, Germany) connected to a data logger (Ahlborn, mod. Almemo® 710). After cooling, crabs were weighed and vacuum-packed (Model EV-13, Tecnotrip, Tarrasa, Barcelona, Spain) prior to pasteurisation.
Heat Treatment Characterisation
After cooking, crabs were vacuum-packed and their cold-spot defined by studying the thermal profile in different locations of the crab anatomy. Once the crab’s cold-spot was identified, the thermal profile of crabs of different weights was characterised at different heat-processing temperatures ranging from 85 to 120 °C in a 90-l-capacity pilot plant scale superheated water shower retort MicroMar-mini (Marrodan, Lodosa, Navarra, Spain). Temperatures were monitored using a K type thermocouple (Ahlborn, Holzikirchen, Germany) placed in the crab cold-spot at time intervals of 1 min using a data logger (Ahlborn, mod. Almemo® 710) connected to a laptop with the data control software version 5.18.2.34 (64-bit). The temperature evolution with time were subsequently fitted to the Ball and Olson equation (Mafart 1994) (Eq. 1A and 1B) and the lethality of the heat treatments, in terms of total F value applied, was also calculated using Eqs. 2 and 3 as explained in Condon-Abanto et al. (2018) for whole brown crab.
Based on these equations, equivalent pasteurisation treatments at different temperatures were calculated based on the crabs weight to reach 6 Log10 reductions of C. botulinum non-proteolytic type E (i.e. F90 of 10 min, considering z value of 10 °C for treatment temperatures over 90 or 7 °C for treatment temperatures below 90 °C). This is based on the recommendations by the US Food and Drug Administration (FDA 2011). After pasteurisation, brown and white crab meats were removed and separated for quality measurements.
Quality Measurements
The quality characteristics considered in the present study, total moisture content, water holding capacity and colour were selected in consultation with different crab producers due to the practical approach considered in this work. Total moisture content and water holding capacity were selected for its importance on the juiciness and the exudates observed in the pack during the storage, and the colour because, as it has been already reported (Pathare et al. 2013), is one of the key parameters involved in the consumer’s choice. However, further chemical analyses will be considered in future research.
Moisture Content and Water Holding Capacity
Moisture content was determined by oven drying (P-model Digitronic Selecta, Barcelona, Spain) following the AOAC (2000) method and results were expressed on a wet weight percentage basis. For the water holding capacity (WHC) determinations, samples (3 g) were wrapped in a plastic mesh and placed in a 50-mL centrifuge tube half-filled with 6-mm diameter glass beads. Samples were centrifuged (Megafuge 1.0 R, Kendro, Germany) for 10 min at 3000×g at room temperature (Martínez et al. 2017). WHC was expressed as the percentage of post cook wet, weight moisture using Eq. 4.
Colour Measurement
A tristimulus colorimeter (Spectrophotometer CM-2002, Konica Minolta, Japan) was used to determine colour parameters of brown and white crab meat after pasteurisation. Results were expressed using the L*, a*, b* CIE colour space. Other colour parameters such as chroma (C*), hue angle (H*), total colour change (ΔE*), whiteness index (WI*) and browning index (BI*) were estimated from L*, a* and b* using the following equations (Pathare et al. 2013):
where L0, a0 and b0 represent the initial values of colour for white and brown meats prior to pasteurisation, and L*, a* and b* are those values following retort pasteurisation.
Where, \( X=\frac{a^{\ast }+1.75\left({L}^{\ast}\right)}{5.645\left({L}^{\ast}\right)+{a}^{\ast }-3.012\left({b}^{\ast}\right)} \)
From these colour parameters, chroma (C*) indicates the colour saturation, hue angle (H*) indicates the colour tone, ΔE* quantifies the total colour change taking as reference the colour after cooking (step 1) and before thermal pasteurisation (cook step 2), whiteness index (WI*) indicates the degree of whiteness and browning index (BI*) indicates the purity of the brown colour.
Colour Change Kinetics
The study of the effect of temperature on colour change kinetics was performed in white meat only. This study was performed under controlled conditions as follows: After cooking, step 1 (as described in the “Raw Materials and Crab Preparation” section), white meat from crab claws and legs was removed. A glass tube with a screw cap lid was filled with approximately 5 g of white meat. The tube was then sealed using a septum and screw cap. A hole in the tube cap allowed the insertion of a syringe to relieve the internal pressure in the tube by removing air/vapour during the beginning of the heat treatment (a schematic description of the tubes is shown in Fig. 1). The colour changes in the white meat during the heat treatment were measured through the tubes using a tristimulus colorimeter (Chroma Meter CR-400). Preliminary trials showed that the glass tube did not interfere with the colour measurements (data not shown). After the initial colour measurements, tubes were submerged in an oil bath (CC-E, Huber, Germany) at 95 °C, 105 °C and 115 °C. Preliminary experiments showed that the tube needed less than 1 min to reach the target temperature (data not shown). A single tube was removed at preselected times and immediately cooled down by submerging it in ice-water. After cooling it down to room temperature (T ≤ 20 °C), the white meat colour was measured again and all colour parameters were calculated.
All experiments were performed in triplicate and ten colour measurements were averaged for each replicate (i.e. tube).
The results obtained were fitted to zero order, first order and pseudo-first order association (Eq. 10A, B and C) kinetic equations, as recent reviews suggest for describing the kinetics of colour change in different food matrices (Ling et al. 2015; Pathare et al. 2013). Due to the complexity of food products, different kinetics have been used to describe for modelling of quality attributes in foods. Depending of the process, food or attribute, kinetics varies from zero-order formation to complex kinetics involving parallel and consecutive reactions, for instance, the Maillard reaction or degradation of some enzymes during heating (van Boekel and Tijskens, 2001). The order of kinetic was selected based on R2 as suggested by Mondal and Dash (2017). The highest correlation (R2) was obtained for the pseudo-first order association kinetic equation for the total colour change index (ΔE*), so for subsequent calculations, this parameter was the only one considered.
where Y0 represents the initial colour, Ymax is the value in which the colour becomes stable for a time (t) value equal to ∞ and k is the rate constant (min−1). Then the effect of the treatment temperature on k and Ymax was studied by plotting the decimal logarithm of these parameters versus the treatment temperature.
Finally, to validate the developed equations, 16 crabs were heat-treated at temperatures ranging from 90 to 120 °C, and the temperature of the crab’s cold-spot was monitored. After treatments, quality parameters were analysed.
Data Processing and Statistical Analyses
Data were processed using GraphPad PRISM 5.0 software (GraphPad Software, Inc., San Diego, CA, USA). Different equations were used to fit the data using the same software. The fitting showing the highest correlation coefficient (R2) and the lowest root-mean-square-error (RMSE) were considered for further calculations (Mondal and Dash 2017). Microsoft Excel 10 was used to develop the final equations. For the statistical analyses, t tests (p = 0.05) and ANOVA tests (p = 0.05) followed by Tukey’s test were performed using GraphPad PRISM 5.0 software to define statistical differences among samples, and differences were considered significant where p ≤ 0.05. Error bars in the figures correspond to the standard error of the mean for at least three independent replicates.
Results and Discussion
In addition to microbial inactivation, heat treatments also induce a series of chemical reactions which modify attributes of foodstuffs. These reactions can be positive including desirable flavour, colour or texture development, but they can also include reactions which are regarded as negative such as undesirable colour, odour or nutrient degradations. Ultimately, these reactions will influence consumer acceptance and, for this reason, an understanding of their development kinetics is essential in order to design thermal processes that better align with consumer satisfaction (Ling et al. 2015). In the case of edible crab, severe heat treatments are generally required for bacterial spore inactivation. Therefore, the main objective of this research was to optimise the pasteurisation treatment conditions (i.e. second thermal treatment) for edible crab after packaging based on the kinetic changes of some crab quality parameters and define different treatment conditions based on the thermal profile of crabs in order to ensure one of the recommended heat treatments for this type of ready-to-eat products (F90°C for 10 min) (FDA 2011).
Effect of Pasteurisation Temperature on Quality Characteristics of Edible Crab
The first step was the development of an equation (derived from Eqs. 1A and 1B) with the objective of characterising the temperature rise in the crab cold-spot, i.e. the abdomen during pasteurisation. Crab weight was also factored into this equation due to the important effect of this parameter on the temperature increase rate. Figure 2a and b show the effect of the crab’s weight on the equation parameters fh and j. It is observed that crab weight had a negligible effect on the j values. Therefore, to compose the final equation, this parameter was fixed at a constant value of 1.56 (± 0.35) units. By contrast, a linear relationship was found between fh value and crab weight. Essentially, this means that the temperature rise in the crab cold-spot is weight-dependent and both parameters are correlated in Eq. 11. These observations are in agreement with those reported in a previous study where the heat penetration of the crab cold-spot was monitored during the initial cooking process (Condon-Abanto et al. 2018)
Effect of crab weight on the heat penetration parameters a fh and bj, estimated from the fitting of Ball and Olson equation to the thermal profiles obtained in the crab cold-spot. Figure 2a also shows the regression line (block line) as well as the 95% confidence intervals (dotted lines)
The combination of Eqs. 1A, 1B and 11, produced Eq. 12 that predicts the evolution of the temperature in the crab cold-spot during retort pasteurisation, as influenced by the initial temperature of the crab, pasteurisation temperature and crab weight.
where Tt is the temperature (°C) at a certain time t (min), Tc is the pasteurisation temperature, T0 is the initial temperature in the crab ‘cold-spot’ and W is the crab weight (grams).
Based on Eq. 12 and using Eqs. 2 and 3, a series of equivalent pasteurisation treatments at temperatures ranging from 90 to 120 °C were defined in order to apply the heat treatment recommended by the FDA for crustaceans (i.e. F90°C10°C = 10 min), in order to produce a 6 Log10-cycle reduction in the population of Clostridium botulinum non-proteolytic type E.
Table 1 summarises the range of pasteurisation conditions in terms of time and temperature as a function of the crab weight ranges. Treatment temperatures did not influence the moisture content which ranged between 58.93 and 65.33% for brown meat and between 77.3 and 79.23% for white meat. By contrast, small differences in the WHC values were detected in both brown and white meat. This parameter varied from 59.75 to 69.77% and from 61.65 to 87.35% for white and brown meat, respectively. Observed moisture values are in the range of previously published data which focused on the effect of crab cooking methods. These results also showing that moisture content of white meat is higher than brown (Anacleto et al. 2011; Cóndon-Abanto et al. 2018; Martínez et al. 2017; Maulvault et al. 2012). In the case of WHC, the values obtained in the present study were higher than those reported by Martínez et al. (2017) for Callinectes sapidus. From a quality perspective, the objective of cooking crab is to coagulate meat proteins in order to produce specific organoleptic attributes. The cooking process also induces shrinkage of meat fibres and aggregation/denaturation of myofibrillar proteins which eventually lead to water loss (Hong et al. 1993; Kong et al. 2008). Therefore, changes in moisture and WHC of the crab meat occur during the first cooking step, rather than during the second heat treatment, the objective of the latter being pasteurisation.
In the case of meat colour, two parameters ΔE* and BI* were calculated (Eqs. 7 and 9, respectively) to assess the effect of pasteurisation temperature on crab meat colour (Table 1). BI* parameter ranged from 70.31 and 110.7 units and ΔE* parameter ranged from 5.05 and 8.85 units for the brown meat. For comparison purposes, the BI* value of brown meat before pasteurisation was 96.49 and parameters L*, a* and b* ranged between 43.3–45.4, 15.9–17.1 and 20.6–24.2, respectively. These values are in the range of those reported by Anacleto et al. (2011) for single cooked edible crab. However, no values which compare the results obtained for double-heat processing have been found in the literature. The slight differences observed in the colour of brown meat following the different pasteurisation treatments might be attributed to the intrinsic variability between crabs instead of the pasteurisation temperature itself. It is well known that brown meat content is very variable, even within crabs of the same batch. It is also highly affected by seasonality (Woll 2006). These factors probably impacted on the finding from the present study which did not show any trend between pasteurisation temperature and colour parameters of brown meat.
Contrary to what was observed in brown meat, pasteurisation significantly affected the colour parameters of white meat. BI* ranged between 14.74 and 39.39 units, and the total colour change (ΔE*) ranged between 3.88 and 18.52. For the non-pasteurised crab, the BI* calculated was 8.05 and values L*, a* and b* ranged from 66.7 to 73.3, 0.37 to − 3.3 and 4.1 to 10, respectively. The L* values observed in this investigation are in agreement with those reported by Anacleto et al. (2011), while the observed a* and b* values were lower. Our results are also in accordance with those reported by Requena et al. (1999) who observed that more intense thermal treatments led to a darker colour in canned blue crab. From the results presented in Table 1, it can be concluded that white meat colour is the quality parameter most affected, among the parameters which were studied in this research, by the pasteurisation conditions. Therefore, this is one of the essential parameters to be controlled during the heat treatment optimization of ready-to-eat whole edible crab once its microbial safety has been assured with the recommended heat treatments.
Colour Change Kinetics of Edible Crab White Meat
Once it was identified that the colour of white meat is a critical parameter which determines the quality of edible crab after pasteurisation, the effect of temperature on meat colour vs time was studied under the controlled conditions described in the ‘Colour Change Kinetics’ section. Table 2 shows the colour parameters L*, a* and b* measured in white meat during processing at different temperatures. The table also shows calculated colour parameters (i.e. C*, H*, BI*, ΔE* and WI*). Of the calculated colour parameters, ΔE*was selected to describe the colour change kinetics of white meat since it was the only colour parameter that varied with both time and temperature.
Equation 10C was used to describe the effect of heating time on ΔE* at different temperatures. Figure 3 illustrates the fitting of Eq. 10C to the data describing the evolution of ΔE* with time at 95, 105 and 115 °C. R2 values were 0.88, 0.92 and 0.93 at 95, 105 and 115 °C, respectively indicating the good correlations. As shown in the figure, ΔE* increased rapidly in the early stages of the heat treatments, but then began to plateau. Figure 4a and b show the relationships between the logarithm of the parameters k and Ymax, respectively vs. treatment temperature. The following linear equation describes the effect of the temperature on the logarithm of these parameters:
where T represents the treatment temperature in °C.
Effect of the processing time on the evolution of ΔE* of crab white meat at 95 °C (black circle), 105 °C (black square) and 115 °C (black triangle). Figure also shows the data fitting lines to Eq. 10C. Error bars represent the standard deviation of three replicates
The variation of ΔE* with temperature (k values) increased 1.8 and 3.0-fold (0.0207, 0.0377 and 0.0615 min−1 for 95, 105 and 115 °C, respectively) when increasing the temperature from 95 to 115 °C. From this relationship, a z value of 40.37 °C was calculated for the colour change rate of crab’s white meat (Fig. 4a). The maximum ΔE* values reached (represented by Ymax parameter) were 8.08, 10.55 and 12.79 ΔE* units at 95, 105 and 115 °C, respectively (Fig. 4b).
Considering the Log-linear relationships between k, Ymax and temperature (T) and substituting these relationships (as represented by Eqs. 13 and 14) into Eq. 10C, Eq. 15 would predict the total colour change in the crab’s white meat during the thermal processing:
where T and t are the temperature and time of the thermal treatments, respectively (Fig. 5).
Colour of processed crab meat has been classified in five general categories: blue, relates to crab blood and/or thermal processing; brown is associated with the Maillard reaction; black relates to metal reactions during canning; yellow is due to lipid oxidation and red is associated with pigments present in white meat (Requena et al. 1999). Figure 6 shows that the ΔE* is highly correlated with Browning index (BI*). This correlation seems to indicate that the main factor involved in the crab meat colour change during thermal pasteurisation is related with the Maillard reaction since it is the main responsible for the development of heat-induced brown colours in the crab meat (Requena et al. 1999). However, while the Maillard reaction has been associated mainly to zero or first-order kinetics, colour changes on crab white meat followed a pseudo-first order kinetics. Therefore, other process such as those related with the degradation of other natural pigments present in crab’s meat, such as carotenoids (mainly astaxanthin) (Maoka 2011) which are responsible for the red colour of most crustaceans developed during cooking. Though, the main reservoir of these pigments is the shell, some of those are also present in the meat or could be transferred from the shell to the meat due to the processing. Therefore, these pigments could be also involved in the colour changes of white meat during thermal processing (Ling et al. 2015; Requena et al. 1999).
Heat Process Optimization and Validation
In the final stage of this study, the optimization of the pasteurisation of ready-to-eat whole edible crab was performed based on microbial recommendations of the US Food and Drug Administration (FDA 2011) (i.e. six decimal logarithmic reductions of Clostridium botulinum non-proteolytic type E). For this optimization, Eqs. 2 and 3 that describe the lethality of heat treatments, Eq. 12 that describes the heat penetration and Eq. 15 that describes the colour change kinetics were used in conjunction with the data obtained from a survey conducted among edible crab producers in Ireland, to define the quality associated with colour developed during thermal pasteurisation. Figure 5 shows the results obtained from the survey performed to the Irish crab producers. As the figure shows, higher values of ΔE* parameter supposed the lower associated quality of the crab meat. The samples cooked/pasteurised during this investigation (in the ‘Effect of Pasteurisation Temperature on Quality Characteristics of Edible Crab’ and ‘Colour Change Kinetics of Edible Crab White Meat’ sections) were classified, by the producers, as ‘good quality’ (ΔE* ≤ 7), ‘acceptable quality’ (7 < ΔE* < 9) and ‘non-acceptable quality’ (ΔE* ≥ 9) based on the colour appearance. Previous work on other products has been suggested that ΔE* values between 1.5 and 3 units represent appreciable differences between food samples (Adekunte et al. 2010; Pathare et al. 2013).
Boundary lines (grey lines, dashed and solid) which define the time/temperature combinations based on the crab’s quality are shown in Fig. 7. The area below the grey dashed line represents the time/temperature combinations which would maintain the ΔE* below or equal to 7 (i.e. ‘good quality’). The area between grey dashed line and solid lines corresponds to the time/temperature combinations which would produce crab with ‘acceptable quality’ (ΔE* > 7 and < 9); and the area above the grey solid line would be related to the time/temperature combinations which would produce crab of ‘non-acceptable quality’ (ΔE* ≥ 9). Figure 7 also shows a series of black lines which define the required time/temperature combinations, based on the heat penetration equation developed in the ‘Effect of Pasteurization Temperature on Quality Characteristics of Edible Crab’ section, to reach 6 Log10-reduction of C. botulinum type E. Different lines correspond to the different crab weights. Based on Fig. 7, the shorter pasteurisation treatments recommended to maintain ‘good quality’ are 100 °C for 55 min and 96 °C for 83 min for crabs of 400 and 800 g, respectively (green area), and ‘acceptable quality’ at 108 °C for 45 min and 104 °C for 70 min for crabs of 400 and 800 g, respectively (blue area). The red area represents the pasteurisation conditions which ensure microbiological safety, based on the recommended F value, but produce undesirable colour changes. The areas which are orange and yellow show the treatments conditions in which a product which is not microbiologically safe is produced.
Theoretical optimisation graph for the pasteurisation process of ready-to-eat whole edible crab based on Eqs. 12 and 13. Grey lines represent the quality boundaries between ‘good’ and ‘acceptable’ (dashed line) and between ‘acceptable’ and ‘non-acceptable’ (block line). Black lines represents the minimum processing conditions (time/temperature) required for crabs of different weights (from bottom to top 400, 500, 600, 700 and 800 g) to achieve an adequate F value based on the inactivation of C. botulinum type E (F90°C10°C = 10 min)
Finally, to evaluate the robustness of the predictions obtained from the equations developed, a series of treatments, at different temperatures ranging from 90 to 120 °C, were defined based on Fig. 7. To validate the predictions of the heat penetration equation (Eq. 12), the crab’s cold-spot temperatures were monitored during the treatment. These measured temperatures from crabs of different weights were plotted against the predicted temperatures from Eq. 12. As Fig. 8a shows, good correlations were observed between real and predicted values with R2 of 0.89 and RMSE of 0.002. Since the treatments were designed to apply an equivalent F value of F9010 = 10 min, the total F values from measured temperatures were also calculated using Eqs. 2 and 3. In all cases, the total F value achieved was higher than 10 min, confirming the robustness of the equation developed. After the pasteurisation treatments, all quality parameters were also measured. As expected, no differences were observed in moisture and WHC among the crabs pasteurised at different temperatures of the white and brown meat and no differences in meat colour values were observed in the brown meat. However, significant differences were observed in white meat colour depending on the pasteurisation conditions as previously demonstrated. To evaluate the robustness of the predictions provided by Eq. 15, the observed ΔE* parameters were plotted against the ΔE* parameters calculated based on the theoretical heat penetration of crab claws (Fig. 8b). As observed in Fig. 8b, a good correlation between the observed and predicted ΔE* values exist, with R2 of 0.85 and RMSE of 0.09, confirming the applicability of the results obtained.
Observed versus predicted values for the a thermal profiles and b white meat colour degradations of crabs of different weights that were pasteurised under the conditions defined in Fig. 7
Conclusions
The main objective of the present work was to evaluate the effect of the pasteurisation treatments on different quality attributes of edible crab and to identify optimal autoclave pasteurisation conditions, based on quality attributes, using mathematical predictions. From the obtained results, the crab cold-spot was located in its abdomen during retort pasteurisation. The time necessary to achieve a target F value was dependent on the crab weight. The pasteurisation temperature did not affect moisture, water holding capacity or the colour of crab brown meat. Colour change of white meat was the most affected quality attribute during the thermal pasteurisation and was consequently used as the indicator for heat process optimization from the organoleptic perspective. The colour change kinetics revealed that the degradation of the colour of white meat followed a pseudo-first order kinetics during thermal treatment. Both the maximum colour change achieved and the colour change rate increased exponentially with treatment temperature. The equations developed in this investigation proved to be useful in designing the thermal pasteurisation treatments for ready-to-eat whole edible crab. These equations indicated that the maximum temperatures to pasteurise whole edible crab were: between 96 and 100 °C and between 104 and 108 °C (depending on the crab weights) in order to obtain ‘good’ or ‘acceptable’ quality, respectively. Results obtained in the present work may be transferred to the crab industry in defining the pasteurisation conditions with less impact on product quality.
References
Adekunte, A. O., Tiwari, B. K., Cullen, P. J., Scannell, A. G. M., & O’Donnell, C. P. (2010). Effect of sonication on colour, ascorbic acid and yeast inactivation in tomato juice. Food Chemistry, 122(3), 500–507.
Anacleto, P., Teixeira, B., Marques, P., Pedro, S., Nunes, M. L., & Marques, A. (2011). Shelf-life of cooked edible crab (Cancer pagurus) stored under refrigerated conditions. LWT - Food Science and Technology, 44(6), 1376–1382.
AOAC (2000). Official methods of analysis of the AOAC journal of the association of Official Analytical Chemists (17th edition), Arlington, Virginia.
Barrento, S., Marques, A., Pedro, S., Vaz-Pires, P., & Nunes, M. L. (2008). The trade of live crustaceans in Portugal: space for technological improvements. ICES Journal of Marine Science: Journal du Conseil, 65(4), 551–559.
Barrento, S., Marques, A., Teixeira, B., Anacleto, P., Carvalho, M. L., Vaz-Pires, P., & Nunes, M. L. (2009). Macro and trace elements in two populations of brown crab Cancer pagurus: ecological and human health implications. Journal of Food Composition and Analysis, 22(1), 65–71.
Barrento, S., Marques, A., Vaz-Pires, P., & Nunes, M. L. (2010). Live shipment of immersed crabs Cancer pagurus from England to Portugal and recovery in stocking tanks: stress parameter characterization. ICES Journal of Marine Science: Journal du Conseil, 67(3), 435–443.
Condón-Abanto, S., Arroyo, C., Álvarez, I., Brunton, N., Whyte, P., & Lyng, J. G. (2018). An assessment of the application of ultrasound in the processing of ready-to-eat whole brown crab (Cancer pagurus). Ultrasonics Sonochemistry, 40(Part A), 497–504.
Edwards, E. & Early, J.C. (2001). Catching, handling and processing crabs. Torry advisory note No. 26, Ministry of technology, Torry research station, Her majesty’s stationery office.
EUROSTAT (2015) http://ec.europa.eu/eurostat/data/database ( last accessed 4.05.2016).
Fante, L., & Noreña, C. P. Z. (2012). Enzyme inactivation kinetics and colour changes in garlic (Allium sativum L.) blanched under different conditions. Journal of Food Engineering, 108(3), 436–443.
FDA, (2000). Kinetics of microbial inactivation for alternative food processing technologies. https://www.fda.gov/downloads/Food/FoodborneIllnessContaminants/UCM545175.pdf. Last accessed March 2018.
FDA (2011). Fish and fishery products hazards and controls guidance, fourth edn. Technical report. US Department of Health and Human Services.
Gökoðlu, N., & Yerlikaya, P. (2003). Determinaton of proximate composition and mineral contents of blue crab (Callinectes sapidus) and swim crab (Portunus pelagicus) caught off the Gulf of Antalya. Food Chemistry, 80(4), 495–498.
Hadjal, T., Dhuique-Mayer, C., Madani, K., Dornier, M., & Achir, N. (2013). Thermal degradation kinetics of xanthophylls from blood orange in model and real food systems. Food Chemistry, 138(4), 2442–2450.
Haefner, J. W. (2005). Modelling biological systems: principles and applications. New York: Springer 463pp.
Hong, G. P., Flick, G. J., & Knobl, G. M. (1993). Development of a prediction computer model for blue crab meat yield based on processing and biological variables. Journal of Aquatic Food Product Technology, 1(3-4), 109–132.
Huss, H. H. (1997). Control of indigenous pathogenic bacteria in seafood. Food Control, 8(2), 91–98.
IFT (2013). Kinetic models for microbial survival during processing. http://www.ift.org/knowledge-center/read-ift-publications/science-reports/research-summits/kinetic-models.aspx. Last accesed January 2018.
Jaiswal, A. K., Gupta, S., & Abu-Ghannam, N. (2012). Kinetic evaluation of colour, texture, polyphenols and antioxidant capacity of Irish York cabbage after blanching treatment. Food Chemistry, 131(1), 63–72.
Kong, F., Tang, J., Lin, M., & Rasco, B. (2008). Thermal effects on chicken and salmon muscles: tenderness, cook loss, area shrinkage, collagen solubility and microstructure. LWT - Food Science and Technology, 41(7), 1210–1222.
Kong, F., Tang, J., Rasco, B., & Crapo, C. (2007). Kinetics of salmon quality changes during thermal processing. Journal of Food Engineering, 83(4), 510–520.
Liaotrakoon, W., De Clercq, N., Van Hoed, V., Van de Walle, D., Lewille, B., & Dewettinck, K. (2013). Impact of thermal treatment on physicochemical, antioxidative and rheological properties of white-flesh and red-flesh dragon fruit (Hylocereus spp.) purees. Food and Bioprocess Technology, 6(2), 416–430.
Ling, B., Tang, J., Kong, F., Mitcham, E., & Wang, S. (2015). Kinetics of food quality changes during thermal processing: a review. Food and Bioprocess Technology, 8(2), 343–358.
Linton, M., Mc Clements, J. M. J., & Patterson, M. F. (2003). Changes in the microbiological quality of shellfish, brought about by treatment with high hydrostatic pressure. International Journal of Food Science & Technology, 38(6), 713–727.
Mafart, P. (1994). Ingenieria industrial alimentaria: Procesos fisicis de conservacion. (1st edn.). Technique et Documentation-Lavoisier. Editorial Acribia, S.A.
Maoka, T. (2011). Carotenoids in marine animals. Marine Drugs, 9(2), 278–293.
Martínez, M. A., Velazquez, G., Cando, D., Núñez-Flores, R., Borderías, A. J., & Moreno, H. M. (2017). Effects of high pressure processing on protein fractions of blue crab (Callinectes sapidus) meat. Innovative Food Science & Emerging Technologies, 41, 323–329.
Maulvault, A. L., Anacleto, P., Lourenço, H. M., Carvalho, M. L., Nunes, M. L., & Marques, A. (2012). Nutritional quality and safety of cooked edible crab (Cancer pagurus). Food Chemistry, 133(2), 277–283.
Mondal, I. H., & Dash, K. K. (2017). Textural, color kinetics, and heat and mass transfer modeling during deep fat frying of Chhena Jhili. Journal of Food Processing and Preservation, 41(2), e12828.
Ovissipour, M., Rasco, B., Tang, J., & Sablani, S. S. (2013). Kinetics of quality changes in whole blue mussel (Mytilus edulis) during pasteurization. Food Research International, 53(1), 141–148.
Pathare, P. B., Opara, U. L., & Al-Said, F. A. J. (2013). Colour measurement and analysis in fresh and processed foods: a review. Food and Bioprocess Technology, 6(1), 36–60.
Requena, D. D., Hale, S. A., Green, D. P., McClure, W. F., & Farkas, B. E. (1999). Detection of discoloration in thermally processed blue crab meat. Journal of the Science of Food and Agriculture, 79(5), 786–791.
Uglow, R. F., Hosie, D. A., Johnson, I. T., & Macmullen, P. H. (1986). Live handling and transport of crustacean shellfish: an investigation of mortalities. Seafish technology SR280 (p. 24). London: MAFF R & D Commission.
van Boekel, M. A. J. S., & Tijskens, L. M. M. (2001). Kinetic modelling. In L. M. M. Tijskens, M. L. A. T. M. Hertog, & B. M. Nicolai (Eds.), Food process modelling (pp. 35–59). Boca Raton: Woodhead PublishingLimited and CRC Press.
Woll, A. K. (2006). Handbook: the edible crab-biology, grading and handling live crabs. Volda: Møreforsking Marine.
Yu, K., Wu, Y., Hu, Z., Cui, S., & Yu, X. (2011). Modeling thermal degradation of litchi texture: comparison of WeLL model and conventional methods. Food Research International, 44(7), 1970–1976.
Zabbia, A., Buys, E. M., & De Kock, H. L. (2012). Undesirable sulphur and carbonyl flavor compounds in UHT Milk: a review. Critical Reviews in Food Science and Nutrition, 52(1), 21–30.
Funding
The authors wish to acknowledge the financial support from the Food Institutional Research Measure (FIRM) funded by the Irish Department of Agriculture, Food and the Marine (project no. R13885) and to the European Regional Development Fund, MINECO-CICYT (AGL2015-69565-P) and the Department of Innovation Research and University of the Aragon Government and European Social Fund (ESF).
Author information
Authors and Affiliations
Corresponding author
Additional information
Publisher’s Note
Springer Nature remains neutral with regard to jurisdictional claims in published maps and institutional affiliations.
The original version of this article was revised: The Figures and captions/legends at the original version of this article are displaced.
Rights and permissions
About this article
Cite this article
Condón-Abanto, S., Raso, J., Arroyo, C. et al. Quality-Based Thermokinetic Optimization of Ready-to-Eat Whole Edible Crab (Cancer pagurus) Pasteurisation Treatments. Food Bioprocess Technol 12, 436–446 (2019). https://doi.org/10.1007/s11947-018-2222-2
Received:
Accepted:
Published:
Issue Date:
DOI: https://doi.org/10.1007/s11947-018-2222-2