Abstract
Diabetes is a common metabolic disease, which might influence susceptibility of the kidney to arsenic toxicity. However, relative report is limited. In this study, we compared the influence of inorganic arsenic (iAs) on renal oxidative damage and urinary metabolic profiles of normal and diabetic mice. Results showed that iAs exposure increased renal lipid peroxidation in diabetic mice and oxidative DNA damage in normal mice, meaning different effects of iAs exposure on normal and diabetic individuals. Nuclear magnetic resonance (NMR)-based metabolome analyses found that diabetes significantly changed urinary metabolic profiles of mice. Oxidative stress-related metabolites, such as arginine, glutamine, methionine, and β-hydroxybutyrate, were found to be changed in diabetic mice. The iAs exposure altered amino acid metabolism, lipid metabolism, carbohydrate metabolism, and energy metabolism in normal and diabetic mice, but had higher influence on metabolic profiles of diabetic mice than normal mice, especially for oxidative stress-related metabolites and metabolisms. Above results indicate that diabetes increased susceptibility to iAs exposure. This study provides basic information on differential toxicity of iAs on renal toxicity and urinary metabolic profiles in normal and diabetic mice and suggests that diabetic individuals should be considered as susceptible population in toxicity assessment of arsenic.
Similar content being viewed by others
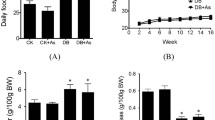
Explore related subjects
Discover the latest articles, news and stories from top researchers in related subjects.Avoid common mistakes on your manuscript.
Introduction
Arsenic (As) is recognized carcinogen and toxicant (Tchounwou et al. 2003), existing inorganic and organic forms in the environment. Inorganic As (iAs) is more toxicity than organic As. Besides carcinogenic effect on the skin, lung, bladder, liver, and kidney, epidemiological studies found that some non-carcinogenic effects such as nephrotoxicity, cardiovascular disease, and diabetes mellitus are associated with chronic exposure of high levels of As (Guha Mazumder 2008; Navas-Acien et al. 2006). The kidney is one of the most susceptible organs to As exposure, which plays important roles in As accumulation or excretion (Nandi et al. 2006). Thus, renal toxicity of As receives more and more attentions. The in vivo study found that As exposure could increase levels of reactive oxygen species (ROS) in the kidney (Wang et al. 2014). Since oxidative stress and damage have been considered as one of the mechanisms of As toxicity, recent studies have suggested that As exposure could induce renal toxicity by disturbing antioxidant defense system and causing oxidative damage (Fowler et al. 2004; Prabu and Muthumani 2012).
Type 2 diabetes mellitus (T2DM) is characterized by disruptions in whole-body glucose homeostasis due to insulin resistance and impaired β cell dysfunction, which could lead to various pathological changes in many tissues, such as the kidney and liver. For example, fatty liver is common in T2DM patients with severity of insulin resistance and dyslipidemia (Kelley et al. 2003), and T2DM can cause new or worsen renal diseases (Patel 2007). Since As exposure could also induce renal and hepatic damages, it could be important to determine whether the T2DM could increase susceptibility of As toxicity on these tissues. Our previous research has reported that As exposure induced higher hepatic oxidative damage in diabetic mice (Liu et al. 2014). For the kidney, Patel and Kalia (2010) found that As exposure induced nephrotoxicity and oxidative damage in the kidney of diabetic rats. However, no report on differential toxicity of As in the kidney of normal and diabetic individuals is available.
Urine is the excretion of the kidney. Thus, urinary metabolites are associated with renal conditions. For example, urinary metabolome analysis has been used in many kidney disease researches to identify potential biomarkers and pathogenic pathways (Ganti and Weiss 2011; Kim et al. 2011). Development of metabolomics provides new approaches, such as proton nuclear magnetic resonance spectroscopy (1H–NMR) and liquid chromatography-tandem mass spectrometry (LC-MS/MS) to comprehensively analyze the urinary metabolic profiles, which could identify the changes in dozens or even hundreds of metabolites (Emwas et al. 2015; Fu et al. 2016).
The objective of this study is to determine differential toxicity of iAs exposure on renal oxidative damage and urinary metabolic profiles in normal and diabetic mice. The C57BLKS/J (db/m) and C57BKS/Leprdb (db/db) mice were chosen as the normal and diabetic mice, respectively. The mice were exposed to iAs or deionized water for 16 weeks. Renal oxidative damage and urinary metabolic profiles in mice were determined. Differential toxicities of iAs on nephrotoxicity and urinary metabolic profiles in normal and diabetic mice were characterized upon the above information.
Materials and methods
Mouse administration
Two types of mice (db/db and db/m mice with 7-week-old) were purchased from Model Animal Research Centre of Nanjing University. All mice were housed in stainless-steel cages with a controlled temperature 25 ± 3 °C, 50 ± 5% humidity, and maintained under a 12-h light/dark cycle. The 16 db/m mice were randomly divided into two groups after 1-week acclimation (8 mice each group), which were fed with deionized water (CK group) and 3-mg/L sodium arsenite solution (CK + As group), respectively. The 16 db/db mice were also randomly divided into two groups without or with 3-mg/L sodium arsenite solution exposure (DB and DB + As groups, respectively). Sodium arsenite was obtained from National Standard Material Center (Beijing, China). After 16-week exposure, urine from each mouse was collected by tubes with 200-μL 0.3% sodium azide solution. All experimental processes were performed according to NIH Guide for the Care and Use of Laboratory Animals. And the protocol was approved by the Committee on the Ethics of Animal Experiments of the Nanjing Military General Hospital.
Determination of total arsenic in the kidney
After mice were anesthetized, the kidney of each mouse was removed, weighed, immediately frozen, and stored −80 °C until further processes. Total As in the kidney was detected using inductively coupled plasma mass spectrometry (ICP-MS) (Rahman et al. 2009). Briefly, 10% (w/v) renal tissue homogenates (500 μL) were digested with HNO3 to a final concentration of 2%. After being filtered with 0.45-μm membrane filter, digested homogenate was used to quantify As concentration in mouse kidney by ICP-MS.
Oxidative stress analysis
Activities of superoxide dismutase (SOD) and glutathione peroxidase (GSH-Px) and levels of lipid peroxidation product malondialdehyde (MDA) were evaluated by commercial kits (Jiancheng, China) (Oberley and Spitz 1984; Yagi 1998). Part of the kidney was firstly homogenized. Then, the protein contents of supernatants were detected using the modified Lowry method (Lowry et al. 1951). The SOD, GSH-Px activates, and MDA level were normalized by protein content. Besides, the 8-hydroxy-2-deoxyguanosine (8-OHdG) was also evaluated to indicate oxidative DNA damage. Genomic DNA of mouse kidney was isolated by Genomic DNA Mini Preparation Kit (Beyotime, China). Concentration and quality of the extracted DNA were determined with Nanodrop 2000 (Thermo Scientific, USA). Then, 8-OHdG level was measured by 8-OHdG enzyme-linked immunosorbent assay (ELISA) kit (Jiancheng, China) and was normalized by DNA content. Each assay was performed in triplicate.
ATPase activity test
Part of the kidney with 1-mL phosphate buffer solution was homogenized and centrifuged for 10 min at 3000×g. Activities of Na+K+-ATPase and Ca2+Mg2+-ATPase in the supernatant were measured by ELISA kit (Jiancheng, China), which was determined by measuring the formation rate of phosphoric acid from ATP. The absorbance was analyzed at 660 nm wavelength. Each assay was performed in triplicate.
Metabolomics analysis
Urinary metabolic profiles in normal and diabetic mice were analyzed by 1H-NMR. Phosphate sodium buffer of 300 μL (0.2 M Na2HPO4; 0.2 M NaH2PO4; 0.25 (w/v) NaN3; 20% (v/v) D2O; 10 mM sodium trimethylsilyl [2,2,3,3-2H4] proionate (TSP); pH 7.4) was added to each mouse urine sample (300 μL). The mixture was centrifuged for 5 min at 14,000×g. Then, 550 μL supernatant was transferred into 5 mm NMR glass tube and analyzed by a Bruker AV600 spectrometer (Bruker, Germany) (Lindon et al. 1999; Zhang et al. 2012). Fourier transformed 1H-NMR spectra were analyzed by MestReC software (MestreC Research, Spain). All spectra were referenced to TSP (δ = 0.00 ppm). Each spectrum was segmented into 0.005-ppm bins. Resonances of water (5.0–4.5 ppm) and urea (5.5–6.0 ppm) were removed prior to normalization. Partial least-square discriminant analysis (PLS-DA) was used to explore the main effect in the NMR data sets by MetaboAnalyst 3.0 (http://www.metaboanalyst.ca/ MetaboAnalyst). Then, metabolites were identified according to their chemical shift (Lindon et al. 1999; Yin et al. 2015) and the Human Metabolome Database (HMDB, http://www.hmdb.ca/). Significantly altered metabolites (SAMs) in treated groups were identified by fold change following the criteria that p < 0.05. The SAMs were selected for further metabolic pathway enrichment analysis using MetaboAnalyst 3.0.
Statistical analysis
Statistical analyses among groups were calculated using one-way ANOVA followed by Tukey’s post hoc test. Before the ANOVA analysis, homogeneity of variances for values was tested. All analyses were performed by Graphpad Prism (version 5.0). The p value <0.05 was considered as statistical significance.
Results and discussion
Relative kidney weight and concentration of As in the kidney
Basic information of body weight and As excretion in db/m and db/db mice with or without iAs exposure has been reported in our previous paper (Liu et al. 2014). In this study, the kidney was chosen as the target organ. Results of relative kidney weight and iAs concentrations are shown in Fig. 1. The db/db mice have higher kidney weight than db/m mice. Since the body weight of db/db mice was very high, relative kidney weight of db/db mice was lower than that of db/m mice. iAs exposure did not change (relative) kidney weight in both kinds of mice. However, iAs exposure significantly increased As concentrations in the kidney of db/m and db/db mice. No significant difference on As concentration in the kidney was found between CK + As and DB + As groups. Above results showed that the kidney might be one of the accumulation organs, which were also reported in the previous report (Chen et al. 1992). Thus, adverse effect of As on the kidney should receive attentions.
Oxidative stress and damage in the kidney
Oxidative stress and damage have been considered as one of the mechanisms of As toxicity in the kidney (Flora 2011; Flora et al. 2005; Forbes et al. 2008). Thus, changes in antioxidant defense system and oxidative damage in normal and diabetic mice were determined after iAs exposure. Results are shown in Fig. 2. No significant difference on SOD activities between db/m and db/db mice was found. iAs exposure did not change the SOD activities in both kinds of mice. For GSH-Px, db/db mice had higher GSH-Px levels than db/m mice. The GSH-Px could inactivate ROS induced by xenobiotics. Increase of GSH-Px level indicates the change in antioxidant defense system. When generation of ROS overloads antioxidant defense system, excessive ROS can affect macromolecules such as lipid, protein, and nucleic acid, altering their morphology and function (Albina et al. 2010; Coskun et al. 2005). Thus, in this study, two biomarkers of oxidative damage, MDA and 8-OHdG, were analyzed. Significant increase of MDA level was only found in DB + As group (p < 0.05), indicating generation of lipid peroxidation (Kurata et al. 1993). However, no statistical difference between two kinds of mice with and without iAs exposure. For 8-OHdG, an oxidation product of DNA (Toyokuni et al. 1997), iAs exposure significantly increased its level in db/m mice. It was interesting that mice in DB group had higher 8-OHdG level than db/m control mice, and iAs exposure did not further increase its level in db/db mice. Above results showed that oxidative stress in db/db control mouse kidney was higher than that in db/m control mice, which is consistent with previous report that oxidative stress is a major culprit in kidney disease in diabetes (Forbes et al. 2008). Moreover, iAs exposure increased MDA levels in diabetic mice but increased 8-OHdG levels in normal mice. The results indicated that iAs exposure could induce the differential effects on oxidative damage.
ATPase activities in the kidney
Activities of Na+K+-ATPase and Ca2+Mg2+-ATPase were analyzed. Results are shown in Fig. 3. There was no difference for Na+K+-ATPase activities among db/m and db/db mice with or without iAs exposure. The iAs exposure increased renal Ca2+Mg2+-ATPase activities in both kind of mice. Statistical difference was found between DB and DB + As groups (p < 0.05), but no difference between CK + As and DB + As groups. The results showed that iAs exposure might disturb energy consumption in normal and diabetic mice (Garcia-Sevillano et al. 2014).
Changes in urinary metabolic profiles
Urinary metabolic profiles were determined by 1H-NMR and analyzed by PLS-DA. Results showed that db/db mice in DB and DB + As groups were separated from db/m mice in CK and CK + As groups (Fig. 4), suggesting big differences in metabolic profiles between db/m and db/db mice. iAs exposure had higher influences on diabetic mice than normal mice, indicating that diabetes increased susceptibility of As toxicity on urinary metabolic profiles.
In order to explore biological meaning of altered metabolic profiles, the SAMs in treated groups were further analyzed and discussed. With the criteria that p < 0.05, a total of 28 and 28 SAMs were identified in DB group (compared to CK group) and DB + As group (compared to CK + As group), respectively (Table S1). For mice with or without iAs exposure, there were 8 and 12 SAMs in CK + As group (compared to CK group) and DB + As group (compared to DB group), respectively. Among these SAMs, ascorbate, ATP, creatine, hippurate, histidine, and itaconic acid were specially found in DB + As group. Choline and taurine were specially found in CK + As group.
The SAMs in each group were mapped into biological pathways by MetaboAnalyst 3.0. Compared to db/m control mice, amino acid metabolism, carbohydrate metabolism, energy metabolism, lipid metabolism, and nucleotide metabolism were significantly changed in db/db control mice (Table S2). iAs exposure mainly changed amino acid metabolism and carbohydrate metabolism in both kind of mice. A total of 11 and 18 altered metabolic pathways were filtered out in CK + As group (compared to CK group) and DB + As group (compared to DB group), respectively. Results of SAMs and relative pathways further showed that iAs exposure had higher influence on metabolic profiles of diabetic mice than normal mice.
Changes in oxidative stress-related metabolisms
Oxidative stress has been proven to be associated with diabetes (Matsuda and Shimomura 2013), which plays a key role in insulin resistance and β cell dysfunction. In this study, compared to CK group, mice in DB group had high alterations in oxidative stress-related metabolisms, including arginine and proline metabolism, cysteine and methionine metabolism, alanine, aspartate and glutamate metabolism, and purine metabolism. The SAMs including arginine, glutamine, glutamate, methionine, and β-hydroxybutyrate were involved in these oxidative stress-related metabolisms, which were down-regulated in DB group (compared to CK group). Our previous research found that above metabolites and metabolic pathways were also changed in serum of diabetic mice (Liu et al. 2014). Arginine can ameliorate oxidative stress in alloxan-induced experimental diabetes mellitus and mediate oxygen metabolites that might be due to its direct chemical interaction with oxygen radicals (El-Missiry et al. 2004; Lass et al. 2002). The β-hydroxybutyrate is an endogenous histone deacetylase inhibitor to suppress oxidative stress (Shimazu et al. 2013). Glutamine and methionine can be used to synthesize antioxidant GSH and maintain intracellular GSH level to inactivate ROS (Matés et al. 2002; Meister 1981; Patra et al. 2001). Since GSH-Px levels were increased in DB group (Fig. 2), it could be deduced that the increase of GSH-Px levels might be the reason of decrease in glutamine and methionine.
When iAs was exposed to normal and diabetic mice, oxidative stress-related metabolites and metabolisms were also changed in both type of mice. In DB + As group (vs DB group), ascorbate and histidine were specially changed, compared to the results of CK + As group vs CK group. Ascorbate was found to be involved in antioxidant defense system, which could take part in the ascorbate-GSH cycle (Apel and Hirt 2004). Histidine is a known scavenger of hydroxyl radicals and singlet oxygen (Pisarenko 1996). In this study, diabetic effects down-regulated histidine level based on the results of DB group vs CK group. However, iAs exposure increased its level, which might be due to that mouse kidney in DB + As group need more histidine to reduce As-induced oxidative stress. Above results showed that iAs exposure might induce higher influence on urinary metabolic profiles of diabetic mice than normal mice.
Changes in carbohydrate and energy metabolisms
Diabetic effect induced high changes in carbohydrate metabolism in mouse urine (Tables S1 and S2), which was consistent with previous literatures (Ajala et al. 2013; Birkenfeld and Shulman 2014). Compared to CK and DB groups, iAs exposure significantly changed 6 and 8 carbohydrate metabolism pathways in CK + As and BD + As groups, respectively. The result was consistent with our previous research that As exposure could alter the carbohydrate metabolism in ICR mouse (Liu et al. 2013). As shown in Fig. 5, carbohydrate metabolism is associated with energy metabolism. For example, citrate cycle (TCA cycle) is one of the carbohydrate metabolisms that occur in the inner mitochondrial membrane and play an important role in energy metabolism (Friedrich 2012). iAs exposure significantly increased intermediate metabolites of TCA cycle, including citrate and succinate (Tannahill et al. 2013). Changes in TCA cycle could also affect other metabolites, such as acetate and alanine (Fig. 5). Meanwhile, butanoate metabolism, glycolysis or gluconeogenesis, glyoxylate and dicarboxylate metabolism, propanoate metabolism, and pyruvate metabolism were also disrupted in CK + As and DB + As groups after iAs exposure, indicating that iAs exposure could disrupt energy metabolism in db/m and db/db mice. The results are consistent with result of Ca2+Mg2+-ATPase activities (Fig. 3), which showed that iAs exposure increased ATPase activities in both kind of mice. It was very interesting that, for energy metabolism, iAs exposure altered methane metabolism and nitrogen metabolism in diabetic mice, but not in normal mice. And more SAMs and altered metabolisms associated with carbohydrate and energy metabolisms were found in diabetic mice than normal mice, indicating that iAs exposure had higher influence on diabetic mice than normal mice.
Perturbed metabolites and pathways in CK + As and DB + As groups induced by iAs exposure compared to CK or DB control groups, respectively. Arrowhead indicates that metabolites were significantly up-regulated or down-regulated. Horizontal line indicates no change. The blue and red were applied to indicate the CK + As and DB + As groups, respectively
Changes in amino acid and lipid metabolisms
In diabetic mice of DB group, amino acid metabolism was significantly changed compared to CK group, indicating that the diabetic effect could disturb the metabolic pathway. The result has been widely verified and discussed in previous literatures (Dheer et al. 2015; Pavlik et al. 2010; Wang et al. 2015). iAs exposure further changed amino acid metabolism (Table S2). For example, aspartate and glutamate metabolism and glycine, serine and threonine metabolism that include the changes of succinate, choline, and alanine were altered in CK + As group compared with CK group. Further, as shown in Fig. 5, increase of succinate and histidine and decrease of creatine and alanine in DB + As group (compared to DB group) lead to the changes in alanine, aspartate and glutamate metabolism, arginine and proline metabolism, histidine metabolism, and tyrosine metabolism. Above results showed that iAs exposure could disturb amino acid metabolism in normal and diabetic mice (Table S1 and Fig. 5).
In addition, lipid metabolism in the diabetic mice of DB group was altered compared to CK group. Previous studies have reported the same result (Butte 2000; Scheuermann-Freestone et al. 2003). Like the amino acid metabolism, iAs exposure could significantly change lipid metabolism in both kinds of mice. Glycerophospholipid metabolism and primary bile acid biosynthesis which are associated with choline and acetate were significantly changed in CK + As group. Glycerolipid metabolism and primary bile acid biosynthesis were altered in DB + As group. The results showed that iAs exposure caused different effects on lipid metabolism in normal and diabetic mice.
In conclusion, iAs exposure caused nephrotoxicity, including oxidative damage, DNA damage, and changes in urinary metabolic profiles in normal and diabetic mice. Further, iAs exposure altered amino acid metabolism, lipid metabolism, carbohydrate metabolism, and energy metabolism in normal and diabetic mice. Compared to normal mice, diabetes increased susceptibility of iAs toxicity in urinary metabolic profiles. Our results suggested that diabetic effects should be considered in renal toxicity assessment of arsenic.
References
Ajala O, English P, Pinkney J (2013) Systematic review and meta-analysis of different dietary approaches to the management of type 2 diabetes. Am J Clin Nutr 97:505–516. doi:10.3945/ajcn.112.042457
Albina ML, Alonso V, Linares V, Bellés M, Sirvent JJ, Domingo JL, Sánchez DJ (2010) Effects of exposure to BDE-99 on oxidative status of liver and kidney in adult rats. Toxicology 271:51–56
Apel K, Hirt H (2004) Reactive oxygen species: metabolism, oxidative stress, and signal transduction. Annu rev Plant Biol 55:373–399
Birkenfeld AL, Shulman GI (2014) Nonalcoholic fatty liver disease, hepatic insulin resistance, and type 2 diabetes. Hepatology 59:713–723. doi:10.1002/hep.26672
Butte NF (2000) Carbohydrate and lipid metabolism in pregnancy: normal compared with gestational diabetes mellitus. Am J Clin Nutr 71:1256s–1261s
Chen CJ, Chen CW, Wu MM, Kuo TL (1992) Cancer potential in liver, lung, bladder and kidney due to ingested inorganic arsenic in drinking-water. J Cancer 66:888–892. doi:10.1038/bjc.1992.380
Coskun O, Kanter M, Korkmaz A, Oter S (2005) Quercetin, a flavonoid antioxidant, prevents and protects streptozotocin-induced oxidative stress and β-cell damage in rat pancreas. Pharmacol Res 51:117–123
Dheer R, Patterson J, Dudash M, Stachler EN, Bibby KJ, Stolz DB, Shiva S, Wang Z, Hazen SL, Barchowsky A, Stolza JF (2015) Arsenic induces structural and compositional colonic microbiome change and promotes host nitrogen and amino acid metabolism. Toxicol Appl Pharmacol 289:397–408. doi:10.1016/j.taap.2015.10.020
El-Missiry M, Othman A, Amer M (2004) L-arginine ameliorates oxidative stress in alloxan-induced experimental diabetes mellitus. J Appl Toxicol 24:93–97
Emwas AH, Luchinat C, Turano P, Tenori L, Roy R, Salek RM, Ryan D, Merzaban JS, Kaddurah-Daouk R, Zeri AC, Gowda GAN, Raftery D, Wang Y, Brennan L, Wishart DS (2015) Standardizing the experimental conditions for using urine in NMR-based metabolomic studies with a particular focus on diagnostic studies: a review. Metabolomics 11:872–894. doi:10.1007/s11306-014-0746-7
Flora SJ (2011) Arsenic-induced oxidative stress and its reversibility. Free Radical Bio Med 51:257–281
Flora SJ, Bhadauria S, Pant SC, Dhaked RK (2005) Arsenic induced blood and brain oxidative stress and its response to some thiol chelators in rats. Life Sci 77:2324–2337
Forbes JM, Coughlan MT, Cooper ME (2008) Oxidative stress as a major culprit in kidney disease in diabetes. Diabetes 57:1446–1454
Fowler BA, Whittaker MH, Lipsky M, Wang G, Chen X-Q (2004) Oxidative stress induced by lead, cadmium and arsenic mixtures: 30-day, 90-day, and 180-day drinking water studies in rats: an overview. Biometals 17:567–568
Friedrich N (2012) Metabolomics in diabetes research. J Endocrinol 215:29–42. doi:10.1530/joe-12-0120
Fu JZ, Schoeman JC, Harms AC, van Wietmarschen HA, Vreeken RJ, Berger R, Cuppen BVJ, Lafeber FPJG, van der Greef J, Hankemeier T (2016) Metabolomics profiling of the free and total oxidised lipids in urine by LC-MS/MS: application in patients with rheumatoid arthritis. Anal Bioanal Chem 408:6307–6319. doi:10.1007/s00216-016-9742-2
Ganti S, Weiss RH (2011) Urine metabolomics for kidney cancer detection and biomarker discovery. Urol Oncol -Semin Ori 29(5):551–557
Garcia-Sevillano MA, Contreras-Acuna M, Garcia-Barrera T, Navarro F, Gomez-Ariza JL (2014) Metabolomic study in plasma, liver and kidney of mice exposed to inorganic arsenic based on mass spectrometry. Anal Bioanal Chem 406:1455–1469. doi:10.1007/s00216-013-7564-z
Guha Mazumder DN (2008) Chronic arsenic toxicity & human health. Indian J Med res 128:436–447
Kelley DE, McKolanis TM, Hegazi RAF, Kuller LH, Kalhan SC (2003) Fatty liver in type 2 diabetes mellitus: relation to regional adiposity, fatty acids, and insulin resistance. Am J Physiol Endocrinol Metab 285:E906–E916. doi:10.1152/ajpendo.00117.2003
Kim K, Taylor SL, Ganti S, Guo L, Osier MV, Weiss RH (2011) Urine metabolomic analysis identifies potential biomarkers and pathogenic pathways in kidney cancer. Omics 15:293–303
Kurata M, Suzuki M, Agar NS (1993) Antioxidant systems and erythrocyte life-span in mammals comp. Biochem Physiol B Comp Biochem 106:477–487
Lass A, Suessenbacher A, Wölkart G, Mayer B, Brunner F (2002) Functional and analytical evidence for scavenging of oxygen radicals by L-arginine. Mol Pharmacol 61:1081–1088
Lindon JC, Nicholson JK, Everett JR (1999) NMR spectroscopy of biofluids. Annual Reports on NMR Spectroscopy 38:1–88
Liu S, Guo X, Zhang X, Cui Y, Zhang Y, Wu B (2013) Impact of iron precipitant on toxicity of arsenic in water: a combined in vivo and in vitro study. Environ Sci Technol 47:3432–3438. doi:10.1021/es400176m
Liu S, Guo X, Wu B, Yu H, Zhang X, Li M (2014) Arsenic induces diabetic effects through beta-cell dysfunction and increased gluconeogenesis in mice. Sci Rep 4:6894. doi:10.1038/srep06894
Lowry OH, Rosebrough NJ, Farr AL, Randall RJ (1951) Protein measurement with the Folin phenol reagent. J Biol Chem 193:265–275
Matés JM, Pérez-Gómez C, de Castro IN, Asenjo M, Márquez J (2002) Glutamine and its relationship with intracellular redox status, oxidative stress and cell proliferation/death. Int J Biochem Cell Biol 34:439–458
Matsuda M, Shimomura I (2013) Increased oxidative stress in obesity: implications for metabolic syndrome, diabetes, hypertension, dyslipidemia, atherosclerosis, and cancer. Obes Res Clin Pract 7:e330–e341. doi:10.1016/j.orcp.2013.05.004
Meister A (1981) On the cycles of glutathione metabolism and transport. Curr top Cell Regul 18:21–58
Nandi D, Patra RC, Swarup D (2006) Oxidative stress indices and plasma biochemical parameters during oral exposure to arsenic in rats. Food Chem Toxicol 44:1579–1584. doi:10.1016/j.fct.2006.04.013
Navas-Acien A, Silbergeld EK, Streeter RA, Clark JM, Burke TA, Guallar E (2006) Arsenic exposure and type 2 diabetes: a systematic review of the experimental and epidemiologic evidence. Environ Health Perspect 114:641–648. doi:10.1289/ehp.8551
Oberley LW, Spitz DR (1984) [61] assay of superoxide dismutase activity in tumor tissue. Methods Enzymol 105:457–464
Patel A (2007) Effects of a fixed combination of perindopril and indapamide on macrovascular and microvascular outcomes in patients with type 2 diabetes mellitus (the ADVANCE trial): a randomised controlled trial. Lancet 370:829–840. doi:10.1016/S0140-6736(07)61303-8
Patel HV, Kalia K (2010) Sub-chronic arsenic exposure aggravates nephrotoxicity in experimental diabetic rats. Indian J Exp Biol 48:762–768
Patra R, Swarup D, Dwivedi S (2001) Antioxidant effects of α tocopherol, ascorbic acid and L-methionine on lead induced oxidative stress to the liver, kidney and brain in rats. Toxicology 162:81–88
Pavlik M, Pavlikova D, Staszkova L, Neuberg M, Kaliszova R, Szakova J, Tlustos P (2010) The effect of arsenic contamination on amino acids metabolism in Spinacia oleracea. L Ecotox Environ Safe 73:1309–1313. doi:10.1016/j.ecoenv.2010.07.008
Pisarenko OI (1996) Mechanisms of myocardial protection by amino acids: facts and hypotheses. Clin Exp Pharmacol Physiol 23:627–633
Prabu SM, Muthumani M (2012) Silibinin ameliorates arsenic induced nephrotoxicity by abrogation of oxidative stress, inflammation and apoptosis in rats. Mol Biol Rep 39:11201–11216
Rahman MM, Rahman F, Sansom L, Naidu R, Schmidt O (2009) Arsenic interactions with lipid particles containing iron. Environ Geochem Health 31:201–206. doi:10.1007/s10653-008-9236-z
Scheuermann-Freestone M, Madsen PL, Manners D, Blamire AM, Buckingham RE, Styles P, Radda GK, Neubauer S, Clarke K (2003) Abnormal cardiac and skeletal muscle energy metabolism in patients with type 2 diabetes. Circulation 107:3040–3046. doi:10.1161/01.cir.0000072789.89096.10
Shimazu T, Hirschey MD, Newman J, He WJ, Shirakawa K, Le Moan N, Grueter CA, Lim H, Saunders LR, Stevens RD, Newgard CB, Farese RV, de Cabo R, Ulrich S, Akassoglou K, Verdin E (2013) Suppression of oxidative stress by β-hydroxybutyrate, an endogenous histone deacetylase inhibitor. Science 339:211–214
Tannahill GM, Curtis AM, Adamik J, Palsson-McDermott EM, McGettrick AF, Goel G, Frezza C, Bernard NJ, Kelly B, Foley NH, Zheng L, Gardet A, Tong Z, Jany SS, Corr SC, Haneklaus M, Caffrey BE, Pierce K, Walmsley S, Beasley FC, Cummins E, Nizet V, Whyte M, Taylor CT, Lin H, Masters SL, Gottlieb E, Kelly VP, Clish C, Auron PE, Xavier RJ, O'Neill LAJ (2013) Succinate is an inflammatory signal that induces IL-1 beta through HIF-1 alpha. Nature 496:238–242
Tchounwou PB, Patlolla AK, Centeno JA (2003) Carcinogenic and systemic health effects associated with arsenic exposure - a critical review. Toxicol Pathol 31:575–588. doi:10.1080/01926230390242007
Toyokuni S, Tanaka T, Hatton Y, Nishiyama Y, Yoshida A, Uchida K, Hiai H, Ochi H, Osawa (1997) Quantitative immunohistochemical determination of 8-hydroxy-2′-deoxyguanosine by a monoclonal antibody N45. 1: its application to ferric nitrilotriacetate-induced renal carcinogenesis model. Lab Investig 76:365–374
Wang XN, Zhao HY, Shao YL, Wang P, Wei YR, Zhang WQ, Jiang J, Chen Y, Zhang ZG (2014) Nephroprotective effect of astaxanthin against trivalent inorganic arsenic-induced renal injury in wistar rats. Nutr Res Pract 8:46–53. doi:10.4162/nrp.2014.8.1.46
Wang XX, Mu XL, Zhang J, Huang QY, Alamdar AV, Tian MP, Liua LP, Shen HQ (2015) Serum metabolomics reveals that arsenic exposure disrupted lipid and amino acid metabolism in rats: a step forward in understanding chronic arsenic toxicity. Metallomics 7:544–552. doi:10.1039/c5mt00002e
Yagi K (1998) Simple assay for the level of total lipid peroxides in serum or plasma. Free Radic Antioxid Protocol 108:101–106
Yin J, Zhang X-X, Wu B, Xian Q (2015) Metagenomic insights into tetracycline effects on microbial community and antibiotic resistance of mouse gut. Ecotoxicology 24:2125–2132. doi:10.1007/s10646-015-1540-7
Zhang Y, Zhang X, Wu B, Cheng S (2012) Evaluating the transcriptomic and metabolic profile of mice exposed to source drinking water. Environ Sci Technol 46:78–83. doi:10.1021/es201369x
Acknowledgements
This work was supported by Natural Science Foundation of Jiangsu Province (BK20131270), Foundation of State Key Laboratory of Pollution Control and Resource Reuse, and Fundamental Research Funds for the Central Universities of Nanjing University.
Author information
Authors and Affiliations
Corresponding author
Ethics declarations
Compliance with the NIH Guide for the Care and Use of Laboratory Animals. And the protocol was approved by the Committee on the Ethics of Animal Experiments of the Nanjing Military General Hospital.
Additional information
Responsible editor: Philippe Garrigues
Electronic supplementary material
ESM 1
(DOCX 20 kb)
Rights and permissions
About this article
Cite this article
Yin, J., Liu, S., Yu, J. et al. Differential toxicity of arsenic on renal oxidative damage and urinary metabolic profiles in normal and diabetic mice. Environ Sci Pollut Res 24, 17485–17492 (2017). https://doi.org/10.1007/s11356-017-9391-9
Received:
Accepted:
Published:
Issue Date:
DOI: https://doi.org/10.1007/s11356-017-9391-9