Abstract
Despite its isolation and scarce occupation, Antarctica is not exempt from the input of contaminants related to present and past human activities. Several deleterious compounds, such as the persistent organic pollutants (POPs) may reach Antarctic ecosystems, mostly via atmospheric long-range transport and further deposition. In this context, snow and its seasonal melting water represent a sink to these pollutants and also the last compartment before they reach marine primary producers. In order to assess the concentration of a selection of organic contaminants, a PDMS headspace extraction method was chosen due to its improvement in fieldwork sampling. Samples were collected in King George Island, during the austral summers from 2007 to 2010. PBDEs and PAHs remained under the method detection limits in all of the cases, restricting data interpretation to organochlorine compounds: average Σ HCHs ranged from 1.46 to 4.17, HCBs from 1.36 to 3.77, Σ Drins from <0.35 to 4.29, Σ Chlordanes from 5.72 to 13.3, Σ DDTs from 4.32 to 24.4, and PCBs from 132 to 156 (always in pg kg−1). Results were, in general, in agreement with previous literature. Nevertheless, due to the fact that samples were collected progressively later into the austral summer, one trend can be noticed: the sum of the concentrations in both matrixes seems to decrease, with a proportional increase in snow. Some exceptions can be remarked, hypothetically linked to the passage of South American frontal systems. Finally, results for these two compartments are compatible with the exposure expected for lower trophic-level organisms from such ecosystem.
Similar content being viewed by others
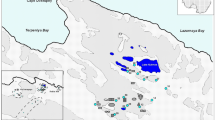
Explore related subjects
Discover the latest articles, news and stories from top researchers in related subjects.Avoid common mistakes on your manuscript.
1 Introduction
Although it remains geographically isolated and scarcely occupied, Antarctica and its ecosystems are not exempt from the input of contaminants related to present and past human activities. Organochlorine pesticides, polychlorinated biphenyls (PCBs), polybrominated diphenyl ethers (PBDEs), and polycyclic aromatic hydrocarbons (PAHs) have a large record of production (also as byproducts), utilization, and inadequate disposal throughout the planet. In the present work, these compounds were analyzed in snow and seasonal melting water samples from five sites located at King George Island (South Shetland Archipelago, Antarctica) collected in the 2007–2008, 2008–2009, and 2009–2010 austral summers. They were chosen not only due to their largely reported deletery effects but also due to the fact that these compounds represent the majority of the ones restricted or banned by the UNEP. Moreover, due to their physico-chemical properties, they can undergo long-range atmospheric transport, evaporating in warmer regions and condensing in colder ones as they are carried by winds. These cycles of evaporation and deposition, known as the “grasshopper effect” (Gouin et al. 2004), allow these contaminants to reach areas with little to none antropic influence, such as Antarctica. Comparable levels of PCB contamination in seawater from north and south of the Antarctic Convergence, which separates sharply defined and distinct water masses, indicated that the atmosphere, not the water, was the dominant pathway for the transport of the PCB compounds to the Antarctic (Montone et al. 2003). The same conclusion can be taken regarding the Arctic (Patton et al. 1989) or even regions that are colder due to altitude, not necessarily latitude (e.g., Wang et al. 2007).
The choice of the matrixes was due to the fact that they represent the interface between biotic and abiotic compartments in this environment. These compounds, once present in the atmosphere, can be removed by several processes that include partition between the air/water phases and wet or dry deposition through ice, snow, rain, particulate matter, soil, and vegetation (Wania et al. 1998; Wania and Mackay 1995). When seasonal melting occurs, these contaminants are released into the Antarctic environment, becoming available in the seawater and therefore to primary producers. The importance of this source has already been reported in both low trophic-level organisms such as krill (Cincinelli et al. 2009) and higher trophic-level organisms (e.g., Geisz et al. 2008).
The work had as aim, therefore, to provide baseline levels of organic pollutants in snow and seasonal melting water for this Antarctic region, after testing an adequate methodology regarding the comparatively lower levels in these matrixes.
2 Materials and Methods
2.1 Sample Collection
Samples of both snow and melting water were collected in five different sites (shown in Fig. 1) in King George Island, Maritime Antarctica (62° 05′ S, 58° 23′ W) during the austral summers of 2007–2008 (late November/early December), 2008–2009 (mid-December), and 2009–2010 (late February/early March), with previously n-hexane-rinsed steel cups, stored in clean glass containers (previously combusted at 450 °C for 4 h), frozen at −20 °C upon return to the Brazilian Research Station (Estação Antártica Comandante Ferraz) and kept frozen until analysis. No filtration was performed in any of the steps before or during analyses. Sampling sites were defined by previous field knowledge, with no formal geomorphological description, but they were basically sandy/rocky creeks of running water above sea level, to ensure the glacier source and to avoid seawater mix.
Since there is a non-linear redistribution of the solutes between the solid and liquid phases (e.g., Gross et al. 1977 and as shown further in this work) only the top 5 cm of snow were collected in order to avoid the interference of percolation and refreezing. For this same reason, the frozen samples were completely defrosted and only then the right mass of water was removed for further analyses.
2.2 Analyses
Given the analytical challenge in analyzing these matrixes, present since early publications (e.g. Peel, 1975), four different methods were tested: adsorption with polyurethane foam (PUF), XAD-2 resin, direct extraction by phase separation, and finally, headspace extraction with polydimethylsiloxane (PDMS) rods, since there is a previously available data for successful analyses of organochlorine pesticides (Silva et al. 2007), PBDEs (Montes et al. 2007), and PAHs/PCBs (Poerschmann et al. 2000) with extraction steps involving PDMS media. The only satisfactory results according to QA/QC criteria (further shown) were achieved with a methodology using headspace extraction with PDMS rods, mostly based upon Montes et al. (2007). Analyses were performed upon sample arrival at the Marine Organic Chemistry Laboratory, University of São Paulo, i.e., in April 2008, 2009, and 2010 for the 2007–2008, 2008–2009, and 2009–2010 austral summers, respectively.
Briefly, 80 ml of the already liquid sample, 24 g of sodium chloride and surrogates were placed in a 110-ml glass vessel. A 10-mm-long PDMS rod (2.0 mm diameter, Goodfellow Cambridge Ltd.), previously conditioned, was placed in the flask headspace with the aid of a metallic pin, and the vessels were hermetically closed. All materials were previously combusted at 450 °C for at least 4 h for decontamination, with the exception of the caps (Teflon® coated), which were soaked in trace analysis quality n-hexane. Extraction was carried out at 95 °C for 14 h, then flasks were left at room temperature for at least 2 h. Rods were removed with decontaminated tweezers, holding by the pin and gently dried using a soft tissue. The PDMS rods were then placed in a 1.5-ml GC autosampler vial and soaked with 1 ml of dichloromethane three times for 5 min each. The remaining 3 ml were evaporated to 150 μl under a gentle nitrogen flow, and 100 μl of internal standards were added. Contrary to the original method, extracts were not evaporated to dryness since previous laboratory tests showed unacceptable loss of lighter PAHs, which could be possibly extended to lighter POPs, such as hexachlorobenzene (HCBs) and hexachlorocyclohexanes (HCHs).
OC analyses were run in a gas chromatograph equipped with an electron capture detector (GC-ECD, Agilent Technologies, model 6890N). Hydrogen was used as carrier gas at a constant pressure (13.2 psi, i.e., 91.01 kPa). The injector was operated in splitless mode and kept at 300 °C. The capillary column used was a DB-5 (30 m length × 250 μm internal diameter × 0.25 μm film thickness). The detector was operated at 320 °C using N2 as makeup gas at a flow rate of 58 ml min−1. The oven was programmed as follows: 70 °C for 1 min, 5 °C min−1 to 140 °C (1 min), 1.5 °C min−1 to 250 °C (1 min), and 10 °C to 300 °C (5 min). The investigated compounds were PCBs (IUPAC no. 8, 18, 28, 31, 33, 44, 49, 52, 56, 60, 66, 70, 74, 77, 87, 95, 97, 99, 101, 105, 110, 114, 118, 123, 126, 128, 132, 138, 141, 149, 151, 153, 156, 157, 158, 167, 169, 170, 174, 177, 180, 183, 187, 189, 194, 195, 199, 201, 203, 206, and 209), DDTs (o,p′-DDE, p,p′-DDE, o,p′-DDD, p,p′-DDD, o,p′-DDT, and p,p′-DDT), HCBs, HCHs (α, β, γ, and δ isomers), chlordanes (α- and γ-chlordane, heptachlor, and heptachlor epoxide), mirex, and drins (aldrin, dieldrin, and endrin). Surrogate recovery ranged from 55 to 82%.
PBDE and PAH analyses were performed in an Agilent 6890 Plus attached to the MS 5973N Mass Selective Detector (GC-MS), with a HP-5MS column (30 m × 250 μm × 0.25 μm). PBDEs analyzed were the IUPAC no. 28, 47, 99, 100, 153, 154, and 183. Injector was operated at 270 °C. Oven was programmed as follows: 130 °C for 1 min, 12 °C min−1 to 154 °C (0 min), 2 °C min−1 to 210 °C (0 min), and 3 °C min−1 to 300 °C (5 min). Surrogate recoveries ranged from 56 to 79%.
PAHs analyzed were naphthalene, 1- and 2- methyl naphtalene, biphenyl, acenaphtylene, acenaphtene, fluorene, fluoranthene, phenantrene, anthracene, pyrene, benz[a]anthracene, and chrysene. Oven was programmed as follows: 40 °C (1 min), 20 °C min−1 to 60 °C (0 min), 5 °C min−1 to 290 °C (0 min), and 10 °C min−1 to 300 °C (10 min). Surrogate recoveries ranged from 55 to 82%.
In regard to QA/QC, method detection limits (MDLs) were set as three times the standard deviation (σ) of seven method blank replicates. Spiked matrices were recovered within the acceptance ranges, i.e., 40–130% for at least 80% of the spiked analytes (Wade and Cantillo 1994). Blanks were included in every analytical batch (usually 10–12 samples), and all data were blank subtracted. For practical and comparative reasons, kilograms and liters of water are used interchangeably. Individual MDLs (pg kg−1) ranged from 0.27 to 0.35 for drins, 0.03 to 0.36 for HCHs, 0.09 to 0.15 for chlordanes, 0.11 to 1.46 for DDTs, and 0.08 to 15.8 for PCBs.
3 Results and Discussion
Since there was no statistically significant spatial variation (Tukey HSD) within the results in any of the three seasons for all the pollutants groups, the whole data for each of the matrixes will be presented as the season average from this point on. None of the compounds within the PBDE and PAH groups could overcome the method detection limits, which were two to three orders of magnitude higher than the ones for the chlorinated compounds The highest MDL for the PBDEs was 650 pg kg−1, and for PAHs it was 2700 pg kg−1, so it is plausible to suppose environmental concentrations (for individual compounds) lower than these values in any of the present samples. Concentrations are shown in Table 1.
Quantitatively, values are, in a general way, in agreement with previous studies. For snow samples along the Antarctic Peninsula (Dickhut et al. 2005), concentrations ranged from 3.17 up to 8.91 pg l−1 whereas heptachlor and its epoxide were up to 7.82 pg l−1. An extensive work (Kang et al. 2012) on surface snow collected during a 1400-km inland traverse beginning in a coastal region in East Antarctica (69° S) presented levels of HCHs and HCBs one to two orders of magnitude higher than our findings. Moreover, this work confirmed a slight increase of α- and γ-HCH with increasing altitude (therefore decreasing temperature) along the route, which corroborates the long distance atmospheric transport of these compounds, even in a much smaller scale.
Literature for melting water (Chiuchiolo et al. 2004) presented values one order of magnitude higher than the present ones for Σ HCHs and Σ DDTs, respectively, ranging from nd up to 0.04 ng l−1 and from nd up to 0.05 ng l−1. However, the cited work separates particulate and dissolved phases, which could represent an overestimation bias if one of the compartments presents proportionally higher concentrations in regard to the other, as it happens indeed. Moreover, since the cited work analyzes melting water resulting from sea ice, the presence of ice algae is not negligible. In another work (Tanabe et al. 1983) containing samples collected much earlier (in 1981, samples dated between 1960 and 1980), results for Σ HCHs in snow went from 1500 up to 4900 pg l−1, i.e., three orders of magnitude higher than our results, whereas Σ DDTs varied from 9 up to 17 pg l−1, within the same order of magnitude of our findings.
Considering that these previous studies used for comparison occurred in latitudes over 62°, one must take into consideration the “cold trap” effect, i.e., the removal of compounds from the atmosphere due to a decrease in temperature and further deposition. It is quite clear that gas exchange is a major, and often dominant, contributor to atmospheric loading in the oceans (Bidleman 1999). This, combined with the fact that biological and degradative pumps present in the Antarctic environment are exposed to high diffusive air to water fluxes, turns this environment into an important net sink of HCHs and, to a minor extent, of HCBs (Galbán-Malagón et al. 2013). The same reference brings evidence that snow might behave seasonally as a significant secondary source of g-HCH to the regional atmosphere. A similar pattern is reported in another work (Kallenborn et al. 2013), reinforcing the plausibility of using these matrixes, especially in regard to lighter and more hydrophilic POPs (e.g., Muir and Lohmann 2013).
Moreover, the temporal dimension of the previous comparisons has to be taken into account, since during the collections of Tanabe et al. (1983) the practical effects of restrictions on POPs were fairly recent, if not inexistent. Therefore, it is reasonable to conclude that the area of study of the present work presents results within the same order of magnitude but consistently lower than other locations closer to the Pole. This is caused by milder temperatures due not only to a lower latitude but also to the smoothing effect of the sea in the Admiralty Bay (located within an island) temperatures compared with continental coasts in higher latitudes.
In the course of the three collection of seasons, which were taken each time later into the austral summer (2007–2008, late November/early December; 2008–2009, mid-December; and 2009–2010, late February/early March), it is possible to notice, in a general way, some trends: the sum of the concentrations in both matrixes seems to decrease,in the same way as a proportional rise in snow concentrations in regard to the melting water ones. Such facts could be explained by the non-linear redistribution of the compounds between these two phases, a previously known phenomenon (as seen in Gross et al. 1977). Besides that, there are some exceptions to be remarked: drin and DDT levels rise in the last season. In the second season, chlordane snow levels rise. HCHs and HCBs showed a far more regular behavior, since in all of the cases the sum of the concentrations in both matrixes decreases and the proportion in snow increases. Montone et al. (2003) had already reported the increase in atmospheric PCB concentrations due to the passage of frontal systems coming from South America, which could represent a source of fluctuation for the present data as well.
In a similar way to the previously presented pesticides, one can notice a slight decrease in PCB levels when both matrixes are summed up along the seasons and also a higher proportion in melting water samples when compared with snow ones. The hypothesis for such results is analogous to the previous one for pesticides: as compounds leave the solid phase in a non-linear manner, therefore the proportion within the snow phase decreases, which in turn leads to an increase in the liquid phase.
Comparison with literature brings total PCBs ranging from 160 up to 1000 pg l−1 (Tanabe et al. 1983), with the same restrictions concerning the sampling time as previously discussed. Grimalt et al. (2009) presented a study with a concentration of 730 pg l−1 in snow for a location situated in the opposite latitude to the one from the present work (62° N, Øvre Neadalsvatn, Norway). This higher value could be justified by the fact that sampling occurred in a location closer to pollutants sources (von Waldow et al. 2010).
Qualitative PCB data is shown in Fig. 2, with congeners distribution (normalized by total PCBs) in the upper part of the graph and then data for the snow/water partition (normalized by chlorination number) in the bottom. Fig. 2 Congeners distribution (top) and snow/water partition (bottom) for PCBs in the three sampling seasons according to chlorination number. White columns for snow; gray ones for water.
In regard to qualitative analyses, the results are once more in accordance to those previously presented for pesticides: in the later, the collection occurs within the melting season and the proportion is found to be higher in de-icing water in comparison with snow. However, with qualitative profiles, it is possible to infer how this transfer happens in function of the chlorination number and therefore, the molecular weight. Variation within the distribution data and also in snow/water partition decreases from the hexachlorinated congener onwards, which would indicate an exposure pattern for primary producers, since the dominant factor regulating the input of contaminants of both ocean water and snow is the same: phase exchange (Bidleman 1999; Galbán-Malagón et al. 2013). Moreover, water resulting from the melting of seasonal snow ends up reaching the ocean, corroborating the previous statement.
Within both distribution and snow/water partition data, it is possible to remark that the variation for hexa- and over-chlorinated congeners is rather small, which would indicate a pattern of exposure for primary producers similar to the one from atmospheric process inputs through long-range transport, confirming the conclusion taken on the previous paragraph. It differs from what occurs in regard to lighter congeners, up to five chlorines, especially the tetra- and penta-chlorinated ones, which presented a larger difference regarding the sampling time along the melting season, indicating an intermediate qualitative profile in addition to intermediate concentrations.
However, when the sampling seasons are compared, it is noticeable in both congener distribution and snow/water partition that there is a larger difference between the first and second sampling seasons than between the second and the third. It was not exactly expected since the intervals between the collections would suggest otherwise: there are about 15 days of difference between the first and second seasons (regarding the start of the austral summer) and roughly two and a half months between the second and third. This could suggest a transient regime at the beginning of the melting season that would gradually become closer to a permanent regime of phase equilibrium. Indeed, literature brings an analytical phase equilibrium model (Wania 1997) demonstrating that during melting, such compounds can be lost to the liquid phase and then enter a new equilibrium state, re-evaporate or even be adsorbed to organic matter, which would partly explain the differences hereby found. Empiric data supporting this claim is given in literature for snow samples presenting concentrations five times higher than old ones for α-HCH and roughly the double for γ-HCH (Patton et al. 1989).
This reinforces the need for future studies with several collections during the same melting season and, especially, during the previous winter in order to better understand how the accumulation and further release of contaminants occur in a temporal scale.
References
Bidleman, T. F. (1999). Atmospheric transport and air-surface exchange of pesticides. In Fate of pesticides in the atmosphere: implications for environmental risk assessment (pp. 115–166). Dordrecht: Springer Netherlands. doi:10.1007/978-94-017-1536-2_6.
Chiuchiolo, A. L., Dickhut, R. M., Cochran, M. A., & Ducklow, H. W. (2004). Persistent organic pollutants at the base of the Antarctic marine food web. Environmental Science & Technology, 38(13), 3551–3557 http://www.ncbi.nlm.nih.gov/pubmed/15296304.
Cincinelli, A., Martellini, T., Del Bubba, M., Lepri, L., Corsolini, S., Borghesi, N., et al. (2009). Organochlorine pesticide air-water exchange and bioconcentration in krill in the Ross Sea. Environmental Pollution (Barking, Essex: 1987), 157(7), 2153–2158. doi:10.1016/j.envpol.2009.02.010.
Dickhut, R. M., Cincinelli, A., Cochran, M., & Ducklow, H. W. (2005). Atmospheric concentrations and air-water flux of organochlorine pesticides along the Western Antarctic Peninsula. Environmental Science & Technology, 39(2), 465–470 http://www.ncbi.nlm.nih.gov/pubmed/15707045.
Galbán-Malagón, C., Cabrerizo, A., Caballero, G., & Dachs, J. (2013). Atmospheric occurrence and deposition of hexachlorobenzene and hexachlorocyclohexanes in the Southern Ocean and Antarctic Peninsula. Atmospheric Environment, 80, 41–49. doi:10.1016/j.atmosenv.2013.07.061.
Geisz, H. N., Dickhut, R. M., Cochran, M. A., Fraser, W. R., & Ducklow, H. W. (2008). Melting glaciers: a probable source of DDT to the Antarctic marine ecosystem. Environmental Science & Technology, 42(11), 3958–3962 http://www.ncbi.nlm.nih.gov/pubmed/18589951.
Gouin, T., Mackay, D., Jones, K. C., Harner, T., & Meijer, S. N. (2004). Evidence for the “grasshopper” effect and fractionation during long-range atmospheric transport of organic contaminants. Environmental Pollution, 128(1–2), 139–148. doi:10.1016/j.envpol.2003.08.025.
Grimalt, J. O., Fernández, P., & Quiroz, R. (2009). Input of organochlorine compounds by snow to European high mountain lakes. Freshwater Biology, 54(12), 2533–2542. doi:10.1111/j.1365-2427.2009.02302.x.
Gross, G. W., Wong, P. M., & Humes, K. (1977). Concentration dependent solute redistribution at the ice–water phase boundary. III. Spontaneous convection. Chloride solutions. The Journal of Chemical Physics, 67(11), 5264. doi:10.1063/1.434704.
Kallenborn, R., Breivik, K., Eckhardt, S., Lunder, C. R., Manø, S., Schlabach, M., & Stohl, A. (2013). Long-term monitoring of persistent organic pollutants (POPs) at the Norwegian Troll station in Dronning Maud Land, Antarctica. Atmospheric Chemistry and Physics, 13(14), 6983–6992. doi:10.5194/acp-13-6983-2013.
Kang, J. H., Son, M. H., Hur, S. D., Hong, S., Motoyama, H., Fukui, K., & Chang, Y. S. (2012). Deposition of organochlorine pesticides into the surface snow of East Antarctica. Science of the Total Environment, 433, 290–295. doi:10.1016/j.scitotenv.2012.06.037.
Montes, R., Rodríguez, I., Rubí, E., & Cela, R. (2007). Suitability of polydimethylsiloxane rods for the headspace sorptive extraction of polybrominated diphenyl ethers from water samples. Journal of Chromatography A, 1143(1–2), 41–47. doi:10.1016/j.chroma.2007.01.036.
Montone, R. C., Taniguchi, S., & Weber, R. R. (2003). PCBs in the atmosphere of king George Island, Antarctica. The Science of the Total Environment, 308(1–3), 167–173. doi:10.1016/S0048-9697(02)00649-6.
Muir, D., & Lohmann, R. (2013). Water as a new matrix for global assessment of hydrophilic POPs. TrAC-Trends in Analytical Chemistry, 46, 162–172. doi:10.1016/j.trac.2012.12.019.
Patton, G. W., Hinckley, D. a., Walla, M. D., Bidleman, T. F., & Hargrave, B. T. (1989). Airborne organochlorines in the Canadian high Arctic. Tellus B, 41B(3), 243–255. doi:10.1111/j.1600-0889.1989.tb00304.x.
Peel, D. A. (1975). The search for organochlorine residues in Antarctic snow. Adsorption Journal of The International Adsorption Society, 108–111.
Poerschmann, J., Górecki, T., & Kopinke, F.-D. (2000). Sorption of very hydrophobic organic compounds onto poly(dimethylsiloxane) and dissolved humic organic matter. 1. Adsorption or partitioning of VHOC on PDMS-coated solid-phase microextraction fibers—a never-ending story? Environmental Science & Technology, 34(17), 3824–3830. doi:10.1021/es000038b.
Silva, R. C., Zuin, V. G., Yariwake, J. H., Eberlin, M. N., & Augusto, F. (2007). Fiber introduction mass spectrometry : Determination of pesticides in herbal infusions using a novel sol–gel PDMS/PVA fiber for solid-phase microextraction. Journal of Mass Spectrometry, 42, 825–829. doi:10.1002/jms.
Tanabe, S., Hidaka, H., & Tatsukawa, R. (1983). PCBs and chlorinated hydrocarbon pesticides in Antarctic atmosphere and hydrosphere. Chemosphere, 12(2), 277–288. doi:10.1016/0045-6535(83)90171-6.
von Waldow, H., Macleod, M., Scheringer, M., & Hungerbühler, K. (2010). Quantifying remoteness from emission sources of persistent organic pollutants on a global scale. Environmental Science & Technology, 44(8), 2791–2796. doi:10.1021/es9030694.
Wade, T. L., & Cantillo, A. Y. (1994). Use of standards and reference materials in the measurement of chlorinated hydrocarbon residues. Chemistry Workbook. NOAA Technical Memorandum NOS ORCA, 77, 59 http://www.jodc.go.jp/info/ioc_doc/Technical/103571e.pdf.
Wang, F., Zhu, T., Xu, B., & Kang, S. (2007). Organochlorine pesticides in fresh-fallen snow on East Rongbuk Glacier of Mt. Qomolangma (Everest). Science in China Series D: Earth Sciences, 50(7), 1097–1102. doi:10.1007/s11430-007-0079-8.
Wania, F. (1997). Modelling the fate of non-polar organic chemicals in an ageing snowpack. Chemosphere, 35(10), 2345–2363.
Wania, F., Hoff, J. T., Jia, C. Q., & Mackay, D. (1998). The effects of snow and ice on the environmental behaviour of hydrophobic organic chemicals. Environmental Pollution, 102(1), 25–41. doi:10.1016/S0269-7491(98)00073-6.
Wania, F., & Mackay, D. (1995). A global distribution model for persistent organic chemicals. Science of the Total Environment, 160–161, 211–232. doi:10.1016/0048-9697(95)04358-8.
Acknowledgements
Collection of samples was part of the project “Environmental Management of Admiralty Bay, King George Island, Antarctica: Persistent organic pollutants and sewage” (contract no. 55.0348/2002-6), funded by the Brazilian Antarctic Program (PROANTAR), Ministry of the Environment (MMA), and Conselho Nacional de Desenvolvimento Científico e Tecnológico (Brazilian National Council for Scientific and Technological Development - CNPq). Antarctic logistics was provided by “Secretaria da Comissão Interministerial para os Recursos do Mar” (Secretary of the Interministerial Commission on Marine Resources - SECIRM). CVZ Cipro was funded by the São Paulo Research Foundation (FAPESP grant no. 2007/55956-0) and also by the Brazilian Coordination for the Improvement of Higher Education Personnel (CAPES, PDEE grant no. 2999/10-2) during the course of this work.
Author information
Authors and Affiliations
Corresponding author
Rights and permissions
About this article
Cite this article
Cipro, C.V.Z., Taniguchi, S. & Montone, R.C. Organic Pollutants in Snow and Seasonal Melting Water from King George Island, Antarctica. Water Air Soil Pollut 228, 149 (2017). https://doi.org/10.1007/s11270-017-3325-5
Received:
Accepted:
Published:
DOI: https://doi.org/10.1007/s11270-017-3325-5