Abstract
The removal of organic pollutants from contaminated water was the interest of numerous research works, which demonstrated the efficiency of adsorption and organo-modified bentonite-based adsorbents in discharging organic pollution from wastewater. However, the availability and the high cost of those adsorbents have limited further application; this study aims to solve these issues. The intercalation of an organic surfactant, tetraheptylammonium bromide (THPAB) onto the surface of Algerian bentonite from the deposit M’zila we named Ben, yields a new material. The synthesized tetraheptylammonium bentonite (THPA-Ben) underwent characterization before testing its effectiveness as an adsorbent for removing phenol from aqueous solutions. The FTIR, TGA, XRD, and SEM–EDS analysis confirmed successful intercalation of the THPAB onto the Ben surface. The results of adsorption experiment demonstrate the high efficiency of the new adsorbent in removing phenol from aqueous solution. The greatest percentage of the removed phenol was 98.2% when the initial concentration was 5 mg L−1. Adsorbing onto 40 mg of THPA-Ben, the optimum adsorption conditions were: 55 °C; pH values of 4.26 and ~ 9; equilibrium was reached after a contact time of 90 min. The modeling of phenol removal results by kinetic equations shows that the pseudo-second-order model is the best to describe the adsorption process (R2 > 0.9999). The modeled isotherms of phenol adsorption onto the THPA-Ben were well fitted with both the Langmuir and Freundlich isotherm models; the highest phenol adsorption capacity was obtained for the organo-modified bentonite; this indicates that the organo-bentonite THPA-Ben is a promising low-cost adsorbent that enhanced the phenol removal from aqueous media.
Similar content being viewed by others
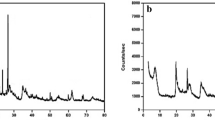
Explore related subjects
Discover the latest articles, news and stories from top researchers in related subjects.Avoid common mistakes on your manuscript.
Introduction
Environmental pollution is caused by enormous quantities of rejects generated from industrial activities [1,2,3]. The large development of chemical and petrochemical industries generates important amounts of organic pollution in water during manufacturing and processing, and this usually exceeds the level for safe discharge. The presence of organic hydrocarbons on the surface of fresh and salty water causes serious environmental pollution [4,5,6]. Phenol is an organic compound, used in pesticides, explosives, dyes and textiles; therefore, it is classified as harmful for organisms even with low concentrations and potentially dangerous pollutant for human health and is considered to be a prioritized pollutant; consequently, permitted limits are low (0.5–1.0 mg L−1) so they must be removed from wastewater [7,8,9].
Because of its significant advantages such as easy handling, high selectivity, lower operating cost, easy regeneration of adsorbent, the minimized production of chemical or biological sludge, and the important efficiency in removing organic water pollution, adsorption is classified as the most powerful purification method. The adsorption process is strongly affected by the chemistry and surface morphology of the adsorbent [10,11,12,13]. Recently, adsorption has emerged economically and technically important method used for water purification. Developing low-cost adsorbents based on clays and bentonite for removing soluble organic contaminants from water is a hot research topic as evident from the increasing number of publications every year [8, 14, 15]. Consequently, new adsorbents with a strong affinity and high loading capacity have been required; a large amount of them have been used for phenolic compound removal [9, 16,17,18,19,20].
Bentonite is a natural resource available for researchers [21, 22]. It is largely explored in the elimination of some impurities such as inorganic and organic substances [23, 24]. The adsorption performances of row and modified bentonite, such as adsorption capacity, equilibrium time, mechanism and regeneration, are closely related to specific differences among cationic surfactants. The results showed that the adsorption of phenols by modified bentonite mainly occurs through hydrophobic interaction, in which the chain length and the surfactant stacking density have a synergistic effect on the adsorption capacity. Furthermore, additional interactions derived from the functional groups of organic pollutants and Gemini surfactants, such as π–π and XH–π interactions and hydrogen bonds, have all been proven to have positive effects on adsorption [25]. The bentonite is a negatively charged surface, isomorphous substituted with Al3+ for Si4+ in a tetrahedral layer and Mg2+ for Al3+ in the octahedral layer in the presence of inorganic cations on the bentonite surface clay such as Na+ and Ca2+; the hydrophilic nature of natural bentonite makes it strongly hydrated in the presence of water but unlike the compounds are weakly adsorbed on its structure [18, 26, 27]. The adsorption properties of bentonite can be improved by modifying its surface by replacing inorganic exchangeable cations with a cationic surfactant so that the hydrophilic silicate surface of the clay becomes hydrophobic; in this case, the modified clay is called organo-clay [18, 28]. This organic clay has been generally proven as a potential adsorbent [9, 10, 16,17,18,19, 29,30,31,32], especially the organic bentonites [6, 33]. As a result, the organic clay complex is an excellent adsorbent for the removal of phenolic compounds and other organic contaminants from aqueous solutions [34, 35]. In addition, several studies proved that bentonite quaternary ammonium surfactants exhibited good efficacy in removing organic pollutants from wastewater [36,37,38,39].
Recently, surfactants are also used for hydrophobic ion pairing, and this is an effective strategy for converting hydrophilic molecules into hydrophobic complexes by pairing them with oppositely charged counter ions [40,41,42]. Many published reports have been dedicated to studying the effect of the structure of quaternary ammonium surfactants, including alkyl chain, spacer, head group and functional group, on the interlayer of modified bentonite [39, 43, 44]. Bentonite has been modified by different quaternary ammonium cationic surfactants: octyltrimethyl ammonium bromide (OTAB), dodecyltrimethylammonium bromide (DTAB), cetyltrimethylammonium bromide (CTAB) and stearyltrimethylammonium bromide (STAB) under similar conditions, and the adsorption capacity of bentonite was investigated [9]. To the best of our knowledge, there has been no study on tetraheptylammonium bromide THPAB surfactant used for the modification of bentonite.
Accordingly, removing phenols from water and wastewater by various techniques as adsorption is an important issue to protect human health and the environment. Extensive research has been carried out to develop innovative materials and technics to deal with phenol organic water pollution [19, 33]. S. Richards et al. [45] have concluded a significant increase in phenol adsorption capacity onto mineral clay after a modest and simple organic modification using organic surfactants HDTMA TMPA. However, Díaz-Nava et al. [46] found that the adsorption of phenol depends on the kind of surfactant as organo-modifier for clay-based adsorbents, and the adsorption was higher for the clay modified with 2 M NaOH than HDTMA. The experimental results obtained by Y. Xu et al. [35] demonstrate that the organo-modified montmorillonite-based adsorbents used for phenol removal are a low-cost material with high adsorption efficiency of phenol and organic pollutants from wastewater; that was confirmed in 2023 by the research work of R. Wei et al. [25] which confirmed that the organo-modified montmorillonite can serve as a green and potential adsorbent used efficiently in eliminating phenol from water.
This paper falls within the scope of scientific research on an intermediate material and its efficiency in environmental applications. The main goal of this study is to explore a new low-cost effective adsorbent which is a surfactant-modified clay minerals for removing organic pollutants (phenol) from aqueous solutions. In this paper, bentonite (Ben) was selected as a case of clay minerals due to its extensive application in the removal of pollutants. A quaternary ammonium organic cationic tetraheptylammonium bromide (THPAB: [CH3(CH2)6]4N(Br)) surfactants with four alkyl groups ions (C7H15−) and traditional halogen ion (Br−) were prepared and used to modify sodium bentonite (Na-Ben). Then, the modified Ben was applied to adsorb phenol in an aqueous solution. The performance characterization of tetraheptylammonium bentonite (THPA-Ben) was studied in detail. The effects of some important experimental factors on the adsorption process such as temperature, pH, adsorbent dose, initial concentrations of phenol, and contact time were investigated. Moreover, the adsorption kinetics was also discussed.
Materials and experimental methods
Materials
The bentonite used in this study was brought from the deposit of M’zila, a region in Mostaganem town (northwestern Algeria). This raw bentonite was used in this study as an adsorbent after purification and chemical modifications. Phenol, a commercial organic compound (analytical reagent grade, C6H5OH, M = 94.11 g/mol, with a purity of 98%), was supplied by China Chemistry Co. Ltd. (Shanghai, China), the chemical structure of which is illustrated in Fig. 1a. Tetraheptylammonium bromide (THPAB), an organic cationic surfactant (C28H60N+Br−, M = 490.69 g/mol, purity ≥ 99%), was obtained from Fluka, the chemical structure of which is presented in Fig. 1b. All the reagents were of analytical grade used as received without further purification. Distilled water also was used in all experiments.
Preparation of adsorbents
Raw bentonite is a mixture of minerals and fragments of crystalline impurities [47]. Practically, clays are purified to remove these impurities. To perform the purification, 10 g of crude bentonite were dispersed in a beaker containing 1L of distilled water, the mixture was stirred for 20 min at room temperature; the suspension was left to stand for 24 h. The supernatant phase was recovered, filtered and dried at 80 °C; the solid, after cooling in a desiccator, was ground and sieved. A quantity of 10 g of the sieved bentonite was immersed in 500 mL of 0.5 N HCl solution under magnetic stirring for 4 h at room temperature to remove carbonates (CO32−).
The bentonite suspension was then filtered, and the solid was washed several times with distilled water until the chloride ions (Cl−) disappeared, as proved by a negative test with silver nitrate (AgNO3). The purified clay was then dried, crushed and sieved, making it ready to be modified.
The purified Algerian bentonite (Ben) underwent a cation exchange by sodium chloride (NaCl) to obtain sodic bentonite (Na-Ben) [20]. This operation involves sodium homo-ionization by replacing various kinds of exchangeable cations in bentonite (calcium (Ca2+), magnesium (Mg2+), etc.), with sodium ions (Na+). The purified bentonite (Ben) was homo-ionized in a sodium chloride solution (1 M, NaCl) by stirring at room temperature for 24 h until saturation with (Na+). The residual salts and the rest of the impurities were then removed, and the clay paste was washed with distilled water and then centrifuged to remove from the clay all the NaCl excess salt and the exchanged cations. This operation was repeated several times until the negative test of Cl− ions using the AgNO3 (0.1 N). The resulting Na-Ben was dried at 70°, then ground and stored to be used later [31, 48].
The Na-Ben was intercalated by the organic surfactant: the tetraheptylammonium bromide (THPAB) according to Eq. 1:
where CEC is the cation exchange capacity of bentonite (eq g−1) determined by the standard test method for methylene blue index clay; f is the fraction of the CEC satisfied by THPAB; m cation is the mass of THPAB required to achieve the desired fraction of CEC (g); m clay is the mass of the clay (bentonite) (g); MM cation is the molecular mass of THPAB (g mol−1); and X is the charge by mole per equivalent (mol eq−1). The CEC represents the cations proportion of the bentonite exchangeable for other cations on a scale ranging from 0 to 1.0, and the extremes correspond to 0 and 100% exchangeability. In this study, we used to exchange 100% of the bentonite cations with the THPAB molecules (100% of CEC), yielding a value of f = 1.0 as illustrated in the literature [36]. In the intercalation, 10 grams of < 2 µm Na-Ben particles, were treated with the required amount of the cationic surfactant (THPAB) solution for 48 h under stirring at natural pH. The resulting mixture was filtered. Then, the solid was washed several times with pure water, centrifuged and then dried at 60 °C. The new modified clay (THPA-Ben) was sieved and stored in glass vials for further use and characterization.
Characterization methods
The Fourier transform infrared (FTIR) spectroscopic technique was used to demonstrate the chemical properties and the structural groups of the material, operated using a spectrometer Thermo Scientific. The X-ray diffraction (XRD) analysis was used to determine the crystal and mineralogical properties, and the X-ray diffraction specimens were obtained from a gentle press of the modified bentonites powder and then analyzed using a PANalytical EMPYREAN diffractometer. The thermogravimetric analysis (TGA) studies the thermal comportment of materials using an SDTQ600 analyzer. The scanning electron microscopy (SEM) using PHILIPS XL 30S microscope equipped with energy-dispersive X-ray spectroscopy (EDS) was used to investigate the surface morphology and elemental analysis, respectively.
Adsorption experiments
Adsorption experiments were applied to the new obtained organo-bentonite, and they were performed to determine the time needed to reach equilibrium and the pattern of the kinetics. For this purpose, a stock solution of 1000 mg L−1 was prepared by dissolving phenol in double-distilled water. A sample of 0.04 g of organo-clay was transferred into 100 mL of phenol solutions at a known concentration; the concentrations of phenol were determined in the aqueous solutions by UV–Vis spectroscopy DR6000 at 275 nm. The collected samples at predetermined time intervals from the shaken solutions were centrifuged. The solids were separated from the samples, and the solution supernatant was analyzed by a spectrophotometer at a wavelength of 275 nm for the residual phenol concentration. In the experiments that were studied, the initial concentration of phenol (5, 10, 15, 20, 25 mg L−1) and contact time were investigated to determine the adsorption equilibrium time.
The phenol removal percentage R (%) can be calculated as C0 follows:
where C0 is the initial phenol concentrations, mg L−1, and Ce is the residual concentrations of phenol in the solution, mg L−1.
where qt is the adsorbent capacity, mg g−1; Ct is the adsorbate concentration, mg L−1; C0 is the initial concentration of adsorbate, mg L−1; W is the adsorbent mass, g; and V is the solution volume, L. If the adsorption arrives at equilibrium, Ct equals Ce (the adsorbate equilibrium concentration), while qt equals qe (the equilibrium adsorption capacity) [49].
Results and discussion
Characterization results
The data of the characterization tests of the modified bentonites prepared with different methods indicate the modification of modified clays.
The FTIR spectra of the Na-Ben and THPA-Ben are presented in Fig. 2 shows the peak at 3622 cm−1 bands is attributed to the structural hydroxyl group vibrations in the mineral bentonites (Mg–OH–Al, Al–OH–Al, and Fe–OH–Al units) [28]. The hydroxyl groups stretching and bending vibration bands of the adsorbed water are seen, respectively, around 3425 and 1630 cm−1 for Na-Ben and also at 3423 and 1631 cm−1 for THPA-Ben, in which they occupy the sites of the modified bentonites layers and their octahedral and tetrahedral surface, respectively [50]. The gradual transformation of the silicate layer of the amorphous silica can be observed in the region of the stretching vibrations of Si–O groups at the observed spectral bands at 528, 989, and 1101 cm−1, which are due to the Si–O vibrations of Si–O–Al and Si–O–Si groups, located on the surface of clay minerals [20]. The characteristic absorption bands of the THPA+ cation intercalated into the Na-Ben surface correspond to the observed bands 1455 cm−1, which indicates the presence of C–H bending vibrations of the amine’s group [29].
The TGA results are summarized in the thermograms presented in Fig. 3, where the curves interpret the thermal behavior of the modified bentonites. TGA is a useful tool to evaluate the thermal stability of the synthesized materials by giving a valuable modification [2]. The modified bentonites are Na-Ben and THPA-Ben, under the T changes. TGA is a universal tool to study the thermal stability of surfactant-modified bentonite. Several studies have interpreted that the decomposition process of organo-bentonite often can be divided into four steps: water desorption, dehydration, surfactant desorption and dehydroxylation; it is shown in the thermogram that below 100 °C, the THPA-Ben has lower mass loss than Na-Ben, signifying less free water within the organo-bentonite, which can be explained by the reasons that the surfactant reduces the surface energy of the montmorillonite and converts the hydrophilic surface to the hydrophobic; the dehydrated Na-Ben loosed water until the stability of the rest of the materials after 600 °C [35, 51]. In this work, the mass loss of THPA-Ben was 16% under temperature changes until 600 °C. This is due to surfactant decomposition; above 800 °C, the organo-bentonite lost mass slightly due to its dehydroxylation until the stability of the rest of his weight. According to the TGA results, THPA-Ben can be thermally stable above 800 °C; on the other side, Na-Ben can be thermally stable above 600 °C, and this depends on the organic and inorganic phases grafted inside the bentonite.
The mineralogical compositions of the sodic and organo-bentonites were determined from the XRD analysis results performed on their samples using a PANalytical EMPYREAN automated diffractometer (Cu-Kα) with λ = 1.54 Å in 2Ɵ range of 3–90° (45 kV, 40 mA). The X-ray patterns of the modified bentonites are illustrated in Fig. 4 (a, b). When X-ray irradiates, the bentonite particles scattering occurs and can be described by the Bragg law. According to this law, the layer spacing of Na–Ben and THPA-Ben can be calculated [52]. The diffractogram shows the difference between the two analyzed bentonites. It is clear that with the addition of the intercalant agent, THPAB into the bentonite has a remarkable effect compared with the sodium bentonite case. The main peaks of the Na-Ben and THPA-Ben phases are presented in the diffractograms, as proposed in the literature. The principal clay minerals are montmorillonite and muscovite; the rest of the phases are considered as non-clay minerals: illite and quartz [47, 53]. Otherwise, these phases were classified by material researchers as clay phases and are more commonly used in depollution and water treatment [54,55,56]. With the surfactant chemical modification, the structure of the clay’s layers is changed, and the layer spacing d001 increased from d = 12.44 Å (2Ɵ = 7.22°) to d = 22.72 Å (2Ɵ = 3.89°). The increase in the basal spacing of Na-bentonite by adding the quaternary ammonium surfactant THPAB demonstrates the intercalation of the THPAB molecules into the interlayer space of the sodic bentonite, and this is with the replacement of the inorganic interlayer cations and their hydration water with THPA+ cations, as proposed in the literature. Regarding the orientation and the number of molecular layers in the interlamellar spacing of clay minerals and considering the molecular size of THPAB, the increases in the basal spacing point to a bilayer arrangement for THPAB [28, 57]. The results confirm the modification of bentonite minerals by the organic surfactant that introduces some changes into the crystal structure of bentonite minerals.
In SEM and EDS study, the SEM images of Na-Ben and THPA-Ben were observed and are presented in Fig. 5(a,b). When comparing the two images, we remark a laminated structure for the two materials. They also show a micro-morphology of clays surfaces which changed significantly by intercalation with THPAB. The sodic bentonite has a flake morphology; however, the resulting THPA-Ben has an enormous heterogeneous pore that varied in size [58]. The granular particle shapes are clearly seen that contribute to trapping and adsorbing phenol [59].
Also, the chemical composition of modified bentonite samples was investigated by EDS. The quantitative analysis results are listed in Table 1. The values of the weight percentage of the compositional elements describe the changes in the chemical composition after intercalation of the Na-Ben by THPAB. The appearance of new elements: C, N, and Br in the THPA-Ben form confirms the presence of the THPAB in the structure of the organo-modified clay; the decrease in the weight percentage of some elements: Na, Mg, K, and the disappearing Cl indicate that the cation exchange of the THAP+ was successfully done.
The major element in the structures of the clay is Si with 41.9 and 30.2 wt% for the Na-Ben and THPA-Ben, respectively, along with the Al, Si and O elements of SiO2, and Al2O3 forming the aluminosilicates structure, as proposed in the literature [60].
The adsorption experiments of phenol by the modified bentonites
Effect of contact time and initial phenol concentration
A series of experiments for phenol onto the unmodified, the sodic and the organo-bentonites were carried out at initial phenol concentrations of 5, 10, 15, 20, and 25 mg L−1 at natural pH and ambient temperature. Figure 6 (a, b, c) presents the effect of initial phenol concentration and the contact time of the adsorption process. It shows that phenol adsorption rate onto the unmodified and the modified bentonites (Na-Ben and THPA-Ben) increased rapidly in the first 30 min and then gradually until it reached equilibrium, which is because there were abundant adsorption surface sites at the beginning and less and less vacant sites were available for adsorption with increasing the contact time; in addition, the remaining vacant sites were hard and occupied due to the increasing electrostatic repulsion between the phenol molecule adsorbed on the surface of the tested bentonites and that in the solution [25]. It can also be seen that phenol was better removed onto THPA-Ben with ~ 98% removal percentage compared with ~ 67% onto Na-Ben, and ~ 36% for the unmodified bentonite Ben, which means that the interlacing surfactant (THPAB) has improved the adsorbent character of the intercalated bentonite (THPA-Ben) while freeing more surface and creating new sites. The adsorption process of phenol reaches equilibrium at about 180, 120, and 90 min for Ben, Na-Ben, and THPA-Ben, respectively. Hence, the fixed equilibrium times reflected the most important adsorption capacities of the unmodified and modified bentonites to be used after in all subsequent experiments.
Effect of adsorbent dose
The adsorbent dose is one of the important parameters in the adsorption process, which is due to its role in determining the capacity of retention on its surface [61]. To determine the effects of Ben, Na-Ben, and THPA-Ben doses on phenol adsorption, a series of experiments were performed at ambient conditions, in which phenol initial concentration was 5 mg L−1 (constant), whereas the quantity of the tested adsorbents varied (10, 20, 30, 40, 50 and 60 mg). The dependency of the percentage of phenol removal and the adsorbents amounts are shown in Fig. 7. From the adsorption process results, we concluded that: with increasing the dose of the adsorbent, the phenol removal rate onto the unmodified bentonite increased gradually from 10.16 to 47.27% by changing the Ben dose from 10 to 60 mg; however, with the phenol retention onto the modified bentonites for the amount of adsorbents from 10 to 40 mg, the removal of phenol increased from 41.83 to 64.27% for Na-Ben and from 89.5 to 97.83% for the THPA-Ben, but by increasing the adsorbent dose above 40 mg the removal process increases slightly. We can conclude that the amount of the adsorbent used for removing phenol increased, which is due to an increase in the number of adsorption sites available for the use of the phenol initial concentration; however, when the amount of the adsorbents is higher than 40 mg, that do not have an important influence on the phenol retention, which is due to the presence of so much adsorption sites that occupied the phenol molecules [61, 62].
Effect of pH media
The surface charge of the adsorbent and the ionization degree of the adsorbate are strongly affected by the pH of the aqueous solutions; hence, the uptake of phenol by the adsorbent depends on the solution pH [18]. It is well known that solution pH has an impact on the surface charge of the adsorbent and ionization degree of adsorbate [25].
To evaluate the effect of pH media on the adsorption of phenol onto organo-bentonite, a comparative study of the effect of pH solution on the phenol adsorption process was conducted; the experiments were carried out with an initial phenol concentration of 5 mg L−1 at ambient temperature onto a defined amount (40 mg) of Ben, Na-Ben and THPA-Ben by varying the pH of the initial phenol solutions (adsorbate) in the range of 2–9. Figure 8 presents the experimental results of this study using three bentonites as adsorbents. The uptake of phenol by the Ben, Na-Ben and THPA-Ben is almost constant in the pH range of 2 to 3. However, when the pH value exceeds 3, the adsorption of phenol decreases slightly with THPA-Ben and Na-Ben; on the other side, it increases with Na-Ben and Ben also until its maximum at around 5 pH point and then it decreases. At higher pH values, the ionization degree of phenol and the quantity of OH− ions decrease, thereby the diffusion of phenolic ions is hindered, and the electrostatic repulsion is between the negatively charged surface sites of the adsorbent. Bentonite contains metal oxides mainly Al and Si, which are hydrolyzed, in an aqueous solution, and so, a charge (positive or negative) is created on its surface. This charge is proportional to the pH of the solution which surrounds the oxide particles; so, the removal of phenol at lower pH is greater compared to higher pH [63]. As a result of our experiments, the removal of phenol onto THPA-Ben is greater at lower pH values 2–3 [20, 64, 65] and the pH points 4.25 and ~ 9 were selected as optimum pH values for the adsorption of phenol onto THPA-Ben and the pH point 5 for both Ben and Na-Ben.
Effect of temperature
For studying the temperature effects on the adsorption of phenol onto the organo-bentonite, the capacity of phenol removal was evaluated for the same amount of Ben, Na-Ben and THPA-Ben (40 mg) comparatively for the temperatures of 25, 35, 45 and 55 °C at the natural pH of the phenol solution. The adsorption of phenol onto the three bentonites was affected by temperature.
The evolution of the phenol capacity of removal as a function of temperature, is presented in Fig. 9. The most important capacity of phenol removal onto the unmodified and modified bentonite was at 55 °C; however, it increases proportionally with temperature, which indicates that the sorption of phenol onto Ben, Na-Ben, and THPA-Ben is endothermic as proper with the literature [9].
Kinetic of adsorption
To identify the dynamic mechanism of the phenol sorption process by the organo-bentonites, the following kinetic models were used [29], Eq. 5 for the Lagergren pseudo-first-order model and Eq. 6 for the pseudo-second-order model [18, 49]:
where k1 and k2 are the kinetic equilibrium rate constants for pseudo-first-order and pseudo-second-order in min−1 and g mg−1 min−1, respectively, qe is the amount of phenol adsorbed per unit mass of adsorbent at equilibrium in mg/g and qt is the mass of adsorbed solute per unit mass of adsorbent at time t in mg/g [66, 67].
The model parameters obtained for phenol adsorption onto the three bentonites after fitting the pseudo-first- and pseudo-second-order kinetic models (Figs. 10, 11) are given in Table 2. For the organo-bentonite, the high correlation coefficients indicated that the pseudo-second-order (R2 > 0.9999) models described the phenol adsorption kinetic well as proposed in the literature [18].
Adsorption isotherms
The adsorption isotherms are useful for understanding the mechanism of the adsorption process. They expose the particular relation between the concentration of adsorbate and its degree of accumulation onto the adsorbent surface at a determined surface at a constant temperature [68].
In our study, the Langmuir and Freundlich isotherm models were chosen and applied to describe the adsorption phenol experiment onto the raw and modified bentonites (Ben, Na-Ben, and THPA-Ben), which are shown in Fig. 12, ((A.1), (A.2), (A.3), (B.1), (B.2), (B.3)) according to the classification system for adsorption proposed by Giles.
The Langmuir model assumes that adsorption takes place at specific homogeneous sites on the surface of the adsorbent and also when a site is occupied by an adsorbate molecule, no further adsorption can take place at this site. The linear form of the Langmuir isotherm model can be presented as [18]:
where qe (mg g−1) is the amount of the phenol adsorbed per unit mass of adsorbent, Ce (mg L−1) is the equilibrium phenol concentration in the solution, qmax (mg g−1) is the Langmuir constant related to the maximum monolayer adsorption capacity and b (L mg−1) is the constant related to the free energy or net enthalpy of adsorption. The linear plot of Ce/qe versus Ce indicates that adsorption obeys the Langmuir model, and the constants qmax and b are obtained from the slope and intercept of the linear plot, respectively.
The essential features of the Langmuir isotherm model can be expressed in terms of “KL” a dimensionless constant, separation factor or equilibrium parameter, which is defined by the following equation [18]:
where Co (mg L−1) is the initial amount of adsorbate and b (L mg−1) is the Langmuir constant described above. The KL parameter is considered the most more reliable indicator of the adsorption. There are four probabilities for the KL value:
-
Favorable adsorption 0 < KL < 1;
-
Unfavorable adsorption KL > 1;
-
Linear adsorption KL = 1;
-
Irreversible adsorption KL = 0.
The Freundlich isotherm model is valid for multilayer adsorption on a heterogeneous adsorbent surface with sites that have different energies of adsorption, which is described by the empirical equation given in Eq. 9 [69] and its linear form presented in Eq. 10 [60]:
where the constant KF is the Freundlich adsorbent capacity (mg g−1 (L mg−1)1/n) and n is the reciprocal of reaction order. The values of n depend on the heterogeneity of the adsorbent, and the favorable adsorption process, the value of n should be less than 10 and higher than unity. The values of KF and 1/n are determined from the intercept and slope of the linear plot of log qe versus log Ce, respectively.
The fitted thermodynamic isotherm models of both Langmuir and Freundlich applied on phenol adsorption onto the three adsorbents are shown in Fig. 12 ((A.1), (A.2), (A.3), (B.1), (B.2), (B.3)). As seen from the data organized in Table 3, the correlation coefficients of the Freundlich model (RF2) are comparatively higher than the Langmuir model’s coefficients (RL2) for phenol adsorption onto Ben and Na-Ben. We infer that the phenol adsorption onto both Ben and Na-Ben is better modeled by Freundlich isotherm, while the adsorption pattern of phenol onto THPA-Ben is good fitted with both the Langmuir and Freundlich.
The results are in accordance with those by Rawajfih et al. [70] and Y. Xu et al. [35]. It is seen that KL values are between 0 and 1, which indicates that the phenol adsorption is favorable onto the three adsorbents (Ben, Na-Ben, and THPA-Ben). In the Langmuir isotherm model, the maximum adsorption capacity of phenol adsorption onto the three selected adsorbents increases with the increase in temperature as proposed in the literature. A remarkable high phenol adsorption capacity for the organo-modified bentonite indicates that THPA-Ben is a promising adsorption material for environmental remediation.
Comparison of different sorbents for phenol adsorption
Like zeolite, clays and bentonite have the properties that they can expand their layer structure and they may allow big molecules such as surfactants and phenols [46]. Besides, phenol can be adsorbed on bentonite surface through the hydrogen bonding between oxygen atoms of the silicate layer and hydroxyl groups of phenol and/or charge transfer complexes [71]. In this study, the adsorption efficiency removal of phenol from aqueous solutions onto the synthesized adsorbent THPA-Ben under different conditions was investigated in this work. THPA-Ben retained successfully 98.2% of phenol from the aqueous solution for the lowest initial concentration (C0 = 5 mg L−1) without having a significant effect on the time to reach the equilibrium after 90 min. M. Sprynskyy et al. [72] examine the capacity of phenol adsorption onto the raw, 2 M NaOH and HDTMA-modified zeolite, and their results show that the HDTMA-modified zeolite is characterized by its ability to adsorb phenol from aqueous solution. The best efficiency of phenol removal (> 90%) has been observed at sorption from solutions of low phenol concentrations (< 5 mg L−1) onto the HDTMA-modified zeolite. Phenol removal increased with time, and three stages may be divided into the sorption process: an initial rapid sorption extending over the first 60 minutes, between 60 and 90 min, the stage of slow approach to equilibrium; and the equilibrium stage of 8 h. The comparative scientific results of phenol adsorption onto surfactant-modified adsorbents [9, 19, 57] are given in Table 4. On the other side, we studied the temperature and pH effects, and we found that they have a significant effect on the phenol adsorption process.
Phenol adsorption increases proportionally with the increase in temperature and variation of pH, the 4.25 pH value and the temperature of 55 °C (highest temperature) were selected as the optimum adsorption conditions of phenol onto THPA-Ben. Z. Ceylan et al. [9] studied the phenol adsorption capacity of cetyltrimethylammonium bromide-modified clays (MMT-CTAB) and reported that the highest phenol removal was found at 4 pH media; their results also show that the increase in temperature at all tested phenol concentrations leads to a rise in the phenol removal for MMT-CTAB; this indicates that the sorption of phenol onto MMT-CTAB is endothermic. The initial sorption phenomenon is considered as physical and chemical phenomenon. This effect may be based on the expansion of pore size or the creation of some new active sites on the adsorbent surface.
Conclusions
In this paper, we present a comparative study of phenol adsorption onto sodic natural and sodic bentonite (Ben and Na-Ben, respectively) with an organo-bentonite THPA-Ben. Using a natural Algerian bentonite (Ben), we synthesized Na-Ben by cation exchange, and the THPA-Ben was prepared via intercalation by cationic surfactant THPAB. The synthesized Na-Ben and THPAB-Ben were characterized using FTIR, TGA, XRD, and SEM–EDS. The characterization confirms the intercalation of the THPAB cations into the Na-Ben and demonstrates that the intercalated material has a good property that comes with an important Phenol removal. The intercalation causes a penetration of the phenol into the interlayer space of bentonite as an organic cation when exchanging inorganic cations. Surfactant cations can interact with bentonite through electrostatic interactions, π interactions and van der Waals forces that create enormous heterogeneous pore that varied in size with granular particle shapes having a thermal stability beyond 800 °C.
At the end of this study, we were able to extract the following information:
-
The THPA-Ben presented the most important phenol adsorption capacity of 98.2% from C0 = 5 mg L−1 initial phenol concentration which takes a bit rapid time to reach equilibrium at about 90 min compared with Na-Ben and Ben that reached their adsorption equilibriums after 120 and 180 min, respectively.
-
Temperature and pH have a significant effect on the phenol adsorption process onto the studied adsorbents; the highest adsorption capacity was obtained at 55 °C for the THPA-Ben and acidic pH points 4 and ~ 9 which are the optimum conditions for the greatest phenol removal onto THPA-Ben; however, the best phenol removal for both Ben and Na-Ben was at ~ 5 pH point.
-
The modeling of the results by kinetic equations shows that the pseudo-second-order model is the one that best describes the adsorption process of phenol onto the three studied adsorbents (Ben, Na-Ben, and THPA-Ben) with the kinetic model parameters. The THPA-Ben is best fitted with: R22 = 0.9999 and K2 = 0.108 g g−1 min−1.
-
The phenol adsorption onto both Ben and Na-Ben is better modeled by the Freundlich isotherm, while the adsorption pattern of phenol onto THPA-Ben is good fitted with both the Langmuir and Freundlich. The highest phenol adsorption capacity was obtained for the organo-modified bentonite, which indicates that THPA-Ben is a promising adsorption material for environmental remediation.
-
The amounts of phenol adsorbed per unit mass of adsorbents at equilibrium are 0.7, 0.81, and 1.19 in mg g−1 for Ben, Na-Ben, and THPA-Ben, respectively, demonstrating that the chemical modification enhances the adsorption capacity of the new organo-bentonite THPA-Ben and confirming the efficiency of the surfactant THPAB as organo-modifier for the M’Zila Algerian bentonite.
In conclusion, the THPA-Ben has very good properties and an important efficiency of phenol removal that can classify the THPAB surfactant as a good modifier for the synthesis of the modified organo-bentonites by intercalation to be used as an adsorbent for environmental applications as desalination and water treatments.
Data availability
No data in this study are available in supporting information of this article.
References
I.M.M. Kenawy, M.A.H. Hafez, A.A. Mousa, Z.A. Elbary, K.S. Abou-El-Sherbini, Sustain Environ. Res. 31, 1–14 (2021)
M. Shafaati, M. Miralinaghi, R.H.S.M. Shirazi, E. Moniri, Res. Chem. Intermed. 46, 5231 (2020)
B. Abdollahi, A. Shakeri, S. Aber, M. Sharifi Bonab, Res. Chem. Intermed. 44, 1505 (2018)
M.F. Zawrah, R.M. Khattab, R.A. Gado, SILICON 10, 2055 (2018)
S.K. Chapagain, G. Mohan, A.B. Rimba, C. Payus, I.M. Sudarma, K. Fukushi, Sustain Environ. Res. 32, 1–14 (2022)
O.O. Okwonna, I.J. Otaraku, Sustain Environ. Res. 32, 1–14 (2022)
J. Michałowicz, W. Duda, Stud 16, 347 (2007)
S.P. Sasikala, T.A. Nibila, K.B. Babitha, A.A. Peer Mohamed, A. Solaiappan, Sustain Environ. Res. 1, 1 (2019)
Z. Ceylan, D. Mustafaoglu, E. Malkoc, Part. Sci. Technol. 36, 716 (2018)
Y. Shu, L. Li, Q. Zhang, H. Wu, J. Hazard. Mater. 173, 47 (2010)
M. I. GHAZALA, 212, 127 (2009).
I. K. Benabadji and A. M. Encadreur, (2013).
B. Saad Al-Farhan, Int. J. Nanomater. Chem. 2, 27 (2016)
D. M. Juela, AIChE Annu. Meet. Conf. Proc. 2020 (2020).
S. Bhattacharya, P. Banerjee, P. Das, A. Bhowal, S.K. Majumder, P. Ghosh, Sustain Environ. Res. 30, 17 (2020)
A. Marsal, E. Bautista, I. Ribosa, R. Pons, M.T. García, Appl. Clay Sci. 44, 151 (2009)
U.F. Alkaram, A.A. Mukhlis, A.H. Al-Dujaili, J. Hazard. Mater. 169, 324 (2009)
H.B. Senturk, D. Ozdes, A. Gundogdu, C. Duran, M. Soylak, J. Hazard. Mater. 172, 353 (2009)
S.H. Lin, M.J. Cheng, Waste Manag. 22, 595 (2002)
H. Asnaoui, Y. Dehmani, M. Khalis, E.K. Hachem, Int. J. Environ. Anal. Chem. 102, 3043 (2022)
F. Sahel, F. Sebih, S. Bellahouel, A. Bengueddach, R. Hamacha, Res. Chem. Intermed. 46, 133 (2020)
Z.R. Liu, X.S. Chen, Y. Wang, Q.Q. Tao, Res. Chem. Intermed. 41, 1683 (2015)
S. Oumnih, N. Bekkouch, E.K. Gharibi, N. Fagel, K. Elhamouti, M. El Ouahabi, Sustain Environ. Res. 1, 1 (2019)
S. Achour, S. Amokrane, S. Chegrouche, D. Nibou, O. Baaloudj, Res. Chem. Intermed. 47, 4837 (2021)
A. Phenol, R. Wei, Y. Mo, D. Fu, and H. Liu, (2023).
N. Yilmaz, H. Yilmaz, S. Yapar, Energy Sources. Part A Recover. Util. Environ. Eff. 29, 67 (2007)
Q. Zhou, H.P. He, J.X. Zhu, W. Shen, R.L. Frost, P. Yuan, J. Hazard. Mater. 154, 1025 (2008)
T.S. Anirudhan, M. Ramachandran, J. Colloid Interface Sci. 299, 116 (2006)
R. Jianmin, W. Si-wei, J. Wei, World Acad. Sci Eng. Technol. 65, 790 (2010)
F. Zahaf, N. Dali, R. Marouf, F. Ouadjenia, J. Schott, Desalin. Water Treat. 57, 21045 (2016)
N. Bougdah, N. Messikh, S. Bousba, P. Magri, F. Djazi, R. Zaghdoudi, Chem. Eng. Trans. 60, 223 (2017)
L. Juang, C. Wang, C. Lee, T. Hsu, J. Environ. Eng. Manag. 17, 29 (2007)
H.C. Yu, S.W. You, C. Wang, J.G. Deng, H.C. Hsi, Sustain Environ. Res. 32, 1–13 (2022)
P. Jing, M. Hou, P. Zhao, X. Tang, H. Wan, J. Environ. Sci. (China) 25, 1139 (2013)
Y. Xu, M.A. Khan, F. Wang, M. Xia, W. Lei, Appl. Clay Sci. 162, 204 (2018)
A. dos Santos, M.F. Viante, D.J. Pochapski, A.J. Downs, C.A.P. Almeida, J. Hazard. Mater. 355, 136 (2018)
X. Hu, B. Wang, G. Yan, B. Ge, Arch. Environ. Prot. 48, 37 (2022)
C.L. Yuan, Z.Z. Xu, M.X. Fan, H.Y. Liu, Y.H. Xie, T. Zhu, J. Chem. Pharm. Res. 6, 2233 (2014)
Y. Li, X. Hu, X. Liu, Y. Zhang, Q. Zhao, P. Ning, S. Tian, Chem. Eng. J. 334, 1214 (2018)
D.J. Kozuch, K. Ristroph, R.K. Prud’Homme, P.G. Debenedetti, ACS Nano 14, 6097 (2020)
S. Durairaj, P.N. Raveendran, B.D. Ryu, C. Hong, T.H. Seo, S. Chandramohan, Nanoscale Adv. 2, 4106 (2020)
S.R. Miditana, S.R. Tirukkovalluri, I.M. Raju, S.A. Alim, G. Jaishree, M.L.V.P. Chippada, Sustain Environ. Res. 31, 1–12 (2021)
S. Ho, Water (Switzerland) 14, (2022).
A. Matteo Jörgensen, P. Knoll, S. Haddadzadegan, H. Fabian, A. Hupfauf, R. Gust, R. Georg Jörgensen, A. Bernkop-Schnürch, Int. J. Pharm. 630, 1 (2023)
S. Richards, A. Bouazza, Appl. Clay Sci. 37, 133 (2007)
M.C. Díaz-Nava, M.T. Olguín, M. Solache-Ríos, J. Incl. Phenom. Macrocycl. Chem. 74, 67 (2012)
Z. Faiza, Thèse Dr. Mustapha Stambouli Mascara,Algérie (2017).
D. I. Nistor, N. D. Miron, and I. Siminiceanu, VII, 505 (2006).
A. Khenifi, Z. Bouberka, F. Sekrane, M. Kameche, Z. Derriche, Adsorption 13, 149 (2007)
M. El, H. Abdelkader, D. Salek, L. Youssef, and M. Guellaa, Environ. Sci. Pollut. Res. (2023).
A. Lassoued, M. S. Lassoued, B. Dkhil, A. Gadri, and S. Ammar, J. Mol. Struct. (2017).
T.S. Anirudhan, M. Ramachandran, Process. Saf. Environ. Prot. 95, 215 (2015)
S. Hillier, Clay Miner. 35, 291 (2000)
S. Lagdali, Y. Miyah, M. El-habacha, G. Mahmoudy, Case Stud. Chem Environ. Eng. 8, 100388 (2023)
A. Elgamouz, N. Tijani, I. Shehadi, K. Hasan, Heliyon 5, e02281 (2019)
S. Iaich, Y. Miyah, F. Elazhar, S. Lagdali 235, 27618 (2021)
C.Y. Cao, L.K. Meng, Y.H. Zhao, Desalin. Water Treat. 52, 3504 (2014)
D. Feng, X. Li, X. Wang, J. Li, F. Sun, Z. Sun, T. Zhang, P. Li, Y. Chen, X. Zhang, Appl. Clay Sci. 155, 126 (2018)
A. Naderi, M.A. Delavar, Y. Ghorbani, B. Kaboudin, M. Hosseini, Appl. Clay Sci. 158, 236 (2018)
A. Alshameri, H. He, J. Zhu, Y. Xi, R. Zhu, L. Ma, Q. Tao, Appl. Clay Sci. 159, 83 (2018)
M. Ahmadian, N. Yosefi, A. Toolabi, N. Khanjani, S. Rahimi, A. Fatehizadeh, Asian J. Chem. 24, 3094 (2012)
I.D. Mall, V.C. Srivastava, N.K. Agarwal, I.M. Mishra, Chemosphere 61, 492 (2005)
P.S. Nayak, B.K. Singh, Desalination 207, 71 (2007)
A.G. Espantaleón, J.A. Nieto, M. Fernández, A. Marsal, Appl. Clay Sci. 24, 105 (2003)
P. H. Chang, Z. Li, W. T. Jiang, and B. Sarkar, Clay Minerals for Pharmaceutical Wastewater Treatment (Elsevier Inc., 2018).
N.D. Mu’azu, N. Jarrah, T.S. Kazeem, M. Zubair, M. Al-Harthi, Appl. Clay Sci. 161, 23 (2018)
Q. Yang, M. Gao, W. Zang, Colloids Surfaces A Physicochem. Eng. Asp. 520, 805 (2017)
A. Kausar, M. Iqbal, A. Javed, K. Aftab, Z.I.H. Nazli, H.N. Bhatti, S. Nouren, M. Mobarak, A.Q. Selim, E.A. Mohamed, M.K. Seliem, C.S.T. Araújo, I.L.S. Almeida, H.C. Rezende, S.M.L.O. Marcionilio, J.J.L. Léon, T.N. de Matos, H. Zhang, J. Wang, B. Zhou, Y. Zhou, Z. Dai, Q. Zhou, P. Chriestie, Y. Luo, P. Nanta, K. Kasemwong, W. Skolpap, N.B.M. Hamzaoui, B. Bestani, J. Environ. Chem. Eng. 256, 794 (2018)
N. Thinakaran, P. Baskaralingam, M. Pulikesi, P. Panneerselvam, S. Sivanesan, J. Hazard. Mater. 151, 316 (2008)
Z. Rawajfih, N. Nsour, J. Colloid Interf. Sci. 298, 39 (2006)
I.M. Banat, R.S. Makkar, S.S. Cameotra, Appl. Microbiol. Biotechnol. 53, 495 (2000)
M. Sprynskyy, T. Ligor, M. Lebedynets, B. Buszewski, J. Hazard. Mater. 169, 847 (2009)
Funding
The authors declare no funding was received.
Author information
Authors and Affiliations
Contributions
The corresponding author “ H. Alidra” performed the experiments and wrote and reviewed the main manuscript text. The co-authors “ F. Djazi and B. Keskin” participated in the revision and approval of the manuscript.
Corresponding author
Ethics declarations
Conflict of interest
The authors declare no personal or financial interests that are directly or indirectly related to this work submitted for publication.
Ethical approval
Not applicable to this work.
Additional information
Publisher's Note
Springer Nature remains neutral with regard to jurisdictional claims in published maps and institutional affiliations.
Rights and permissions
Springer Nature or its licensor (e.g. a society or other partner) holds exclusive rights to this article under a publishing agreement with the author(s) or other rightsholder(s); author self-archiving of the accepted manuscript version of this article is solely governed by the terms of such publishing agreement and applicable law.
About this article
Cite this article
Alidra, H., Djazi, F. & Keskin, B. Enhanced phenol removal from aqueous media by adsorption onto organo-modified bentonite. Res Chem Intermed 50, 989–1011 (2024). https://doi.org/10.1007/s11164-023-05196-z
Received:
Accepted:
Published:
Issue Date:
DOI: https://doi.org/10.1007/s11164-023-05196-z