Abstract
The present work aims to evaluate the synergistic effect of imidazolium-based ionic liquids (IL) in the preparation and immobilization of Burkholderia cepacia lipase in a new hybrid support of poly(hydroxybutyrate-co-hydroxyvalerate) (PHBV) and silica. In addition, these biocatalysts were applied in transesterification reactions of crude coconut oil for the production of ethyl esters. Lipase immobilized on hybrid support with [C4min][NTf2] or [C6min][NTf2] ionic liquid exhibited nearly ≈ 1.6-fold immobilization yield greater than IB-Control. The use of IL during the preparation of the hybrid support promoted changes in its morphological structure as presented by B.E.T. and FTIR. Thermogravimetric analyzes were used to characterize the prepared derivatives and support the results of immobilization yields and the synergic effect of IL used. Transesterification reactions applying biocatalysts showed that the conversion maximum in esters (59%) was found under conditions of molar ratio 1:7 (oil:ethanol) in 72 h of reaction. These results were obtained using [C4min][NTf2] during the preparation of the hybrid support for the immobilization of Burkholderia cepacia lipase, thus showing the potential of these new biocatalysts.
Similar content being viewed by others
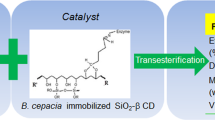
Explore related subjects
Discover the latest articles, news and stories from top researchers in related subjects.Avoid common mistakes on your manuscript.
Introduction
Conventional catalysis promotes the accumulation of toxic waste and presents a high energy expenditure [1]. In order to minimize these adversities, chemical catalysis can be replaced by biological catalysts, such as, the proposed methods with Burkholderia cepacia lipase, which allow for the formation of purer products meanwhile demanding lower energy consumption [2]. Furthermore, the biocatalysts are selective and present high versatility, being able to perform specific biocatalytic reactions, acting under mild conditions of temperature and pressure [3, 4].
Although enzymes have excellent perspectives as catalysts, their industrial application is not immediately apparent due to multiple factors that directly affect the operational stability of lipases and restrict their use on an industrial scale [5]. In view of this, the great emphasis of the last decades has been the use of different immobilization mechanisms to maximize enzymatic stability and optimize biocatalyst performance [6,7,8,9,10,11]. Enzyme immobilization enables not only reuse, but also continuous operation mode and easy product separation with greater stability [12]. At present, several arrays are accessible, including organic and inorganic materials, natural or synthetic materials and hybrid materials [13, 14].
Hybrid materials are constituted by the combination of organic and inorganic components that usually present integral properties, forming a matrix with different attributes from those that originated it [15]. This combination promotes greater stability and availability of reactive functional groups for protein interaction in the hybrid supports, exhibiting properties not observed for their individual components. Among inorganic compounds, silica stands out because it has a high potential for use in immobilization of lipases and expresses several beneficial properties, such as, the possibility of surface modification, thermal and mechanical stability and toxicological safety [16, 17]. It is one of the multifunctional materials most described in literature and can be combined with various organic or inorganic compounds, presenting new physical–chemical properties and extending their applications [14]. Currently, it has been possible to obtain multifunctional silica biomaterials combined with natural polymers for enzymes immobilization [18]. The use of poly(hydroxybutyrate-co-hydroxyvalerate) was highlighted due to its specific attributes, such as biodegradability, biocompatibility and possible use as encapsulating agent [19].
One of the possibilities for more effective biocatalyst synthesis is the use of additives (e.g., ionic liquids) during the immobilization or in the preparation of the supports [15]. Ionic Liquids (ILs), are salts with low melting points, usually below 100 °C, making them liquid at room temperature and are commonly divided in two classes of ILs: aprotic ILs where the formal charge center of the cation is typically formed from non-reversible alkylation of a heteroatom, and protic ILs, whose ion charges depend of a neutralization reaction by a Brønsted acid–base reaction through the transfer of protons [20,21,22,23,24]. The use of protic IL can stabilize enzymes, acting to protect the hydration layer around the enzyme or causing conformational changes in its structure during the hybrid support preparation process [25, 26]. Therefore, until recently, only the potential use of protic IL for hybrid support has been verified. However, the influence of the aprotic IL with different carbonic chains in hybrid support for the synthesis of ethyl esters in transesterification reactions has not yet been verified to the best of our knowledge.
Thus, to obtain a more efficient immobilized biocatalyst, this article aims to verify the influence of IL: ethyl, butyl and hexyl methylimidazolium bis(trifluoromethylsulfonyl) imide, ([Cnmin][NTf2], n = 2, 4 and 6) in the preparation of hybrid supports based on organic and inorganic compound (respectively, PHBV and silica). The Burkholderia cepacia lipase immobilized on hybrid support was analyzed for immobilization yield and biochemical characterizations (pH, temperature, thermal, operational and solvent stability). The supports were characterized by FTIR, B.E.T and thermogravimetry, and finally the biocatalysts were applied in the transesterification reaction of crude coconut oil, and the operating conditions applied were investigated.
Materials and methods
Materials
In this work, three ILs were investigated ([C2min][NTf2], short for 1-Ethyl-3-methylimidazolium bis(trifluoromethylsulfonyl)imide, [C4min][NTf2], short for 1-Butyl-3-methylimidazolium bis(trifluoromethylsulfonyl)imide and [C6min][NTf2], short for 1-Hexyl-3-methylimidazolium bis(trifluoromethylsulfonyl)imide), all of which were purchased from Sigma Aldrich Chemical Co. (St. Louis, MO, USA). Their chemical structures are depicted in Table 1. The Burkholderia cepacia lipase (BCL) was purchased from Sigma Co, St. Louis, MO, USA (≥ 30.000 U g−1, pH 7.0, 50 ºC). Poly(hydroxybutyrate-co-hydroxyvalerate)—PHBV, purchased from Serrana, SP, Brazil). The precursor used in the support preparation was tetraethylortossilicate (TEOS, C8H20O4Si), purity > 98%, purchased from Sigma Aldrich Co (Milwaukee, WI, USA). The other reagents used were hexane, purity > 99% (Synth, Brazil); commercial ethanol, purity > 99.5% (Synth, Brazil); epichlorohydrin, purity > 99% (Synth, Brazil); and ammonium hydroxide, purity > 27% (Synth, Brazil) and olive oil (purchased in the local market).
Hybrid support preparation
Four types of silica supports (Silica-Control, Silica-C2, Silica-C4 and Silica-C6) were prepared along with four types of hybrid supports involving PHBV and silica (hybrid-control, hybrid-C2, hybrid-C4 and hybrid-C6) using the sol–gel method described by Martins et al. [26]. The control was prepared without IL, and the other supports were prepared by adding IL at 1% (m v−1) (ethyl, butyl and hexyl of 3-methylimidazolium bis(trifluoromethysulphonyl)imide), designated by C2, C4 and C6, respectively. The ethanolic solution was prepared by dissolving 72 mL of tetraethylortosilicate (TEOS) in 60 mL absolute ethanol (99%) in an inert nitrogen atmosphere. The ethanolic solution was stirred for 5 min at 25 ºC. Over the course of 90 min, a solution containing 0.22 mL of hydrochloric acid (36%) diluted in 10 mL of ultra-pure water (pre-hydrolyzing solution) was added to the ethanolic solution while stirring. For the preparation of modified hybrid support, 6 g of PHBV was added after adding 1% (m v−1) IL. Then, 1 mL ammonium hydroxide dissolved in 6 mL of ethanol was added. The mixture was kept standing at 35 ºC for 1 h and then kept at 4 ºC for 24 h to complete the polycondensation. The gel was removed and washed with hexane to eliminate moisture and was kept in a desiccator for 72 h.
Immobilization of lipase
The BCL was immobilized to the control silica supports and those modified with IL (silica-control, silica-C2, silica-C4 and silica-C6), to the control PHVB supports and those modified with IL (PHVB-Control, PHVB-C2, PHVB-C4 and PHVB-C6) and to the control hybrid and those modified with IL (HY-Control, HY-C2, HY-C4 and HY-C6). The procedure was performed as described by Paula et al. [27] with some modifications. Enzyme immobilization in the hybrid support (by covalent binding) consisted of connecting the enzyme to the support with aqueous polyethylene glycol (PEG) solution (MW 1500 g mol−1) for a period of 24 h at 4 °C. The support was activated with 2.5% epichlorohydrin (v v−1) in a potassium phosphate buffer (0.1 mol L−1, pH 7.5) at the ratio of 1 g of support to 10 mL of solution. After activation 1% (m v−1) of the IL was added. Then, for each gram of support, 0.25 g of BCL and 100 μL of water containing 5 mg mL−1 of PEG 1500 (g mol−1) was added. The suspension containing enzyme, support and PEG-1500 was homogenized by soft stirring with a glass rod, followed by static contact for an additional 18 h at 4 °C. The biocatalyst was recovered by vacuum filtration separation and washed repeatedly by hexane, and then dried in the desiccator at room temperature for 24 h. In all immobilized lipase preparations, the moisture contents and enzymatic activity were quantified.
Immobilization yields
The lipase hydrolytic activity immobilized from with modifications was determined with the method presented by Soares et al. [28]. The substrate was prepared with 50 mL of olive oil and 50 mL of a sodium phosphate buffer solution (0.1 M, pH 7.0) with gum arabic (7%, w/v). After substrate preparation, a reaction mixture containing 5 mL of the oil emulsion with 4 mL of a sodium phosphate buffer solution (0.1 M, pH 7.0) was prepared. The biocatalyst was incubated in this reaction medium in a thermostatic bath with shaking for 10 min at 37 ºC. The reaction medium was stopped by a solution containing acetone, alcohol and water in equal proportions. The liberated fatty acids were titrated with potassium hydroxide solution (0.04 M) in the presence of phenolphthalein as an indicator. The activity was established as the quantity of BCL that released 1 μmol of fatty acid per minute of hydrolysis reaction. The hydrolytic activities performed on the immobilized biocatalysts (IB) were used to determine the total activity recovery yield, Ya (%), according to Eq. (1).
where U0—units of activity offered for immobilization and Us—units of total enzymatic activity present in the support (enzyme activity [U g−1] × immobilized biocatalyst mass [dry basis, g]).
Morphological and physicochemical characterization
Samples of the ILs, supports and supports with immobilized lipase were additionally analyzed by Fourier transform infrared spectroscopy (FTIR) analysis (Agilent Technologies Cary 630 FTIR instrument). The spectra shown in the present study were extracted in the wavelength range of 600–2000 cm−1. In order to further deepen the study, the secondary structure of the free enzyme and immobilized with and without ILs were studied in the spectra from 1200 to 1800 cm−1. The deconvolutions of the Amide I region (1600–1700 cm−1) were performed using the Origin 8.5 software. Porosity and structure morphological characterization of the hybrid support and IBs were calculated using the Brunauer–Emmett–Teller method (B.E.T.). The supports and IBs porosity study (volume and diameter) were calculated based on the model developed by Barret, Joyner and Halenda (BJH) for mesoporous samples and for microporous samples using the t-method as suggested by Souza et al. [29]. Surface areas were studied according to their N2 adsorption at −196.15 ºC with B.E.T. apparatus software (NOVA 1200e—Surface Area & Pore Size Analyzer, Quantschrome Instruments, version 11.0). The samples were subjected to heat treatment for 48 h at 120 ºC to eliminate any water within the pores of the solids. Thermogravimetric (TG) curves were obtained with a Shimadzu DTG-60H simultaneous DTA-TG apparatus, under a nitrogen atmosphere from 25 ºC to 1000 ºC at a heating rate of 20 ºC min−1 (each sample contained 10.0 ± 1.1 mg). Scanning electron microscopy (SEM; model Hitachi SU-70) was also used to characterize the surfaces of the samples.
pH and temperature analysis
The temperature influence study was performed under pre-established conditions of pH 7.0, in the temperature ranges from 37 to 75 ºC. Relative activity was calculated by the ratio between the immobilized enzyme at the various pH, temperature and the maximum activity determined for the immobilized IBs.
Thermal stability determination
The study of temperature on the stability of the immobilized enzyme was determined by incubating samples of the immobilized biocatalysts from 55 to 65 ºC in aqueous medium (phosphate buffer, pH 7.0, 0.1 M) for 4 h. Samples were taken at different times and evaluated at optimum pH and temperature [30].
Reusability of immobilized lipase
The operational stability of IBs was verified by hydrolysis reaction in olive oil, through subsequent batches with the reuse of the biocatalyst immobilized on hybrid support. The analysis was performed using the same biocatalyst mass and performed several 10 min cycles at 50 ºC and pH 7.0. The biocatalysts were removed by filtration, then washed once with hexane and used for the next reaction cycle. This procedure was repeated several times.
Stability at solvents
We evaluated the effect of organic solvents with different polarities (ethanol and cyclohexane) on the hydrolytic activity of the immobilized biocatalysts by covalent binding. Incubation was performed for 24 h in sodium phosphate buffer, with solvents (ethanol and cyclohexane), and pH 7 at 50 ºC. Samples were taken at 3, 7, 24, 48 and 72 h. Afterward, the activity periods of the lipase immobilized biocatalysts were evaluated by means of the olive oil hydrolysis, as described previously. The residual activity was calculated according to the activity at initial incubation time and the activity measured after incubation [31].
Transesterification reactions
The reactions were performed in sealed bottles containing 3 g of substrate with coconut oil and anhydrous ethanol without the addition of solvents and immersed in a thermostated bath with stirring of 150 rpm [31]. The systems were incubated with the IBs in the proportion of 20% (w/w) in relation to the total mass of reactants involved in the reaction and performed for a period of 120 h under constant agitation of 150 rpm. The conditions for transesterification were performed in different coconut oil:ethanol molar ratios (1:7, 1:9, 1:10, 1:12) and 40 ºC at different reaction times (24, 48, 72, 96 and 120 h). The transesterification reactions were assisted by Dubnoff agitation baths (conventional heating). In order to separate the enzyme, the mixture was centrifuged (3,000 rpm) for 5 min and the glycerol removed with a saturated NaCl solution. The ethyl esters of both phases (aqueous and organic) were extracted with hexane. The organic phase was treated with anhydrous Na2SO4. Ethyl esters quantification were performed using an Agilent Technologies 7820-A GC gas chromatography coupled to the MSD 5975 mass detector and Supelcowax column 10 [32].
Results and discussion
Immobilization yields in different supports
The Burkholderia cepacia lipase was immobilized on different supports (silica, PHBV and hybrid-silica/PHBV), with and without IL ([Cnmin][NTf2], n = 2, 4 and 6). Figure 1 shows the immobilization yield for the biocatalysts immobilized on the different supports evaluated in this work. In a first analysis, evaluating only the supports-control (without modification with IL), it can be observed that the hybrid support showed a higher capacity to maintain lipolytic activity per gram of support, with an immobilization yield of 90 ± 3% while for IB-PHBV and IB-silica immobilization yields were 71 ± 7 and 77 ± 6%, respectively. These results were fundamental for the selection of hybrid supports in this work, capable of reconciling in only one support, unique characteristics of each forming component. The efficiency of the hybrid support was also reported by Fan et al. [33]. The study presented the immobilization of Rhizomucor miehei lipase in a hybrid support formed from dendrimer coated multi-walled magnetic carbon nanotubes of polyamidoamine which showed esterification conversion 27-fold higher than those obtained for the free enzyme.
Figure 1 also shows the effect of different ILs on the immobilization yield of BCL on the different supports. The best immobilization yields were obtained to the immobilized biocatalysts in hybrid support modified with IL in the following order: [C2min][NTf2] < [C4min][NTf2] < [C6min][NTf2] with Ya = 114 ± 2; 171 ± 1; 169 ± 3%, respectively. Lipase immobilized on hybrid support with [C4min][NTf2] or [C6min][NTf2] exhibited about ≈ 1.6-fold immobilization yield greater than IB-Control (90 ± 3%). The synergistic effect in the preparation of IL modified hybrid support may have influenced this behavior. IL with higher alkyl chain (such as [C4min][NTf2] and [C6min][NTf2]) contributed to the increase of hydrophobicity of the support. This may have influenced the preservation of the hydration layer around the enzyme, promoting growth of the interfacial area, complementing the contact between substrate molecules and the enzyme's active site.
To support this data, the FTIR analysis of the IL ([C4min][NTf2] and [C6min][NTf2]) and hybrid support were carried out (Fig. 2). In Fig. 2 it is possible to observe the Si–O-Si (800–1000 cm−1) and Si–OH (900–1100 cm−1) bands characteristic of silica and also the C =O bands (at 1720 cm−1) characteristic of PHBV support [34, 35]. The [Cnmin][NTf2], n = 4 and 6 spectrum exhibited a strong band in the range of 1000 and 1100 cm−1 associated with SN group vibration in 1200 cm−1 for CF3, between 1300 and 1400 cm−1 associated with asymmetric SO2 and between 2880 and 2960 cm−1 referring to the CH elongation. These spectra confirm the presence of [C4min][NTf2] in the modified hybrid support, as indicated in Fig. 2. Thus, it is evident that IL [C4min][NTf2] still remains in the hybrid support matrix even after the wash step with hexane. However, it is clearly observed that the hybrid support prepared with [C6min][NTf2] does not have the characteristic bands that indicate its presence on the support (Fig. 2). This fact can be justified by the higher solvation power of hexane (hydrophobic solvent, measured by the partition in the 1-octanol/water system, log P is 2.22 [36]), for [C6min][NTf2] compared to [C4min][NTf2], due to its greater hydrophobic character and therefore its leaching during washing.
In addition, to understand the possible structural modifications of the lipase immobilization, the second-derivative infrared spectra (FTIR) in the region between 1700 and 1600 cm−1 (Amide I region) were evaluated (Fig. 3). The results feature that all samples of IBs (Fig. 3b–d) exhibit small changes in α-helix content when compared to free enzyme (Fig. 3a). The free enzyme exhibits β-sheet (1638 cm−1), α-helix (1660 cm−1), random coil (1645 cm−1) and β-turn (1670 cm−1) as shown in Fig. 3a. The results feature that all samples of IBs (Fig. 3b–d) exhibit changes in helical structures, when compared to free enzyme (Fig. 3a), this is due the immobilization steps. IB-Control showed an increase in the α-helix, and changes in the β-sheet, random coil, and β-turn contents (Fig. 3b) leading to the decrease observed in the activity recovery. An increase in α-helix content is also observed for IB immobilized with [C6min][NTf2]. These sensitive helical structures, responsible for enzymatic activation in the Amide I region, are in accordance with the recovery yield data observed. In particular, IB-C4 shows a higher decrease in the α-helix content when compared to IB-Control and IB-C6. Therefore, the decrease in α-helix content shows that IL present in IB promoted important conformational alterations of BCL and, thus, increased lipase catalytic performance due to the easiness of the enzyme active site, which corroborates with the increase in lipase activity.
According to Sánchez et al. [37], the catalytic performance of the BCL is conditioned by the conformational changes in its structure during interfacial activation phenomena (open conformation). The opening of α-helix structure can promote the lipase activation because it allows the substrate to come into contact with the active site. Recent studies show that the reduction of the α-helix content is related to the increase in enzyme activity due to the easier access of the substrate to the active site of BCL [10, 38, 39]. These results also agree with the FTIR observation for the absence of [C6mim][NTf2] after the hybrid support preparation step, showing that [C4mim][NTf2] acts both in the preparation of the support and during the lipase immobilization process, remaining present during all steps.
The synergistic effect of IL during support preparation and lipase immobilization has been recently reported [40]. To the best of our knowledge, nothing has been found in literature about the influence of the hydrophobic nature of ionic liquid. This feature becomes important as the synergistic use in both material preparation and enzyme immobilization allowed for a 98% increase in immobilization yield for BCL lipase using [P666(14)][NTf2], reported by Barbosa et al. [40].
B.E.T. physical characterization
The porosity study of the pure hybrid supports and the enzyme immobilized on the control support and modified with IL facilitates the understanding regarding lipase immobilization and catalytic efficiency. From the data collected on the biocatalyst immobilization yields on the hybrid control support (IB-Control) and the most efficient immobilized biocatalysts (IB-C4 and IB-C6) using ILs, [C4min][NTf2] and [C6min][NTf2] were chosen for morphological (B.E.T.), physicochemical (TGA and FTIR), biochemical and solvent stability characterizations.
Table 2 shows the influence of IL during preparation of the hybrid support favored the change in the morphological structure of the support. Although all samples evaluated presented pore size in the mesoporous scale, the presence of ILs ([C4min][NTf2] and [C6min][NTf2]) promoted an increase of surface area 87.37 m2 g−1 to 97.60 m2 g−1 and 95.81 m2 g−1, respectively, compared to the control hybrid support. As presented in FTIR analysis discussed in the previous topic, although IL [C6min][NTf2] remains absent during the immobilization step, its use in support preparation was sufficient to promote a significant modification in support compared to control hybrid support, and this may have contributed to the increase in immobilization yield (Fig. 1). This same effect was observed for the pore volume present in the hybrid support (Table 2). These results confirm the positive effect of [C4min][NTf2] and [C6min][NTf2] on the modification of the morphological characteristics of hybrid support further enhancing lipase concentration and activity in the support, which may allow enzyme accommodation and substrate access.
Studies by Meera et al. [41] obtained similar results on the surface area of an air-gel matrix using choline-based IL, attributing the variation in surface area dimensions and pore size to the influence of the hydrophobicity of the support and the IL used. One can also observe that both surface area and pore volume reduce after biocatalysts immobilization. The presence of BCL lipase in the support surface may confirm the decrease of free surface area and/or prevent access to the support pores.
From the comparison between the derivates of hybrid control absence of IB (HY-C4 and HY-C6) and with immobilized biocatalysts, a remarkable decrease in pore volume was observed, from 0.07674 (HY-C4) to 0.04371 cm3 g−1 (IB-C4) and from 0.08011 (HY-C6) to 0.06464 cm3 g−1 (IB-C6). This same effect is observed for area surface data (Table 2), suggesting that the lipase is immobilized both in the pores and on the surface of the material. These results are in accordance with the literature [42], indicating that enzymes enter the pores by diffusion and are subsequently adsorbed and/or chemically bonded to the inner surface of the support. This effect causes a higher concentration of enzymes molecules inside the hybrid support pores, which can justify higher efficiency of immobilized BCL. Martins et al. [26] obtained similar results when using modified hybrid supports with protic IL for BCL immobilization. Souza et al. [43] observed that the addition of protic IL in the BCL immobilization in silica xerogel modifies the porous structure of immobilized derivatives, causing pore enlargement and increasing surface area and pore volume.
Thermogravimetric analysis (TG)
Thermogravimetric analysis is an extremely important type of physicochemical characterization providing information on enzymatic mass loss with related materials that act as support for immobilization. The study of the degradation profile for the silica/PHBV hybrid support without IL (HY-Control) and modified with IL (HY-C4 and HY-C6) and the immobilized biocatalysts (IB-Control, IB-C4 and IB-C6) are shown in Fig. 4. The thermogravimetric analysis described in Table 3 showed that hybrid supports (HY-Control, HY-C4 and HY-C6) have similar degradation mechanisms, being thermally stable up to 200 °C and losing only 9% of initial mass due to mainly the presence of water. In this region (from 25 to 200 °C), degradation of amino groups present in lipase and the evaporation of water molecules is expected [40]. Si–O-H silanyl groups present in silica prepared by the sol–gel technique tend to absorb water spontaneously. In Table 3 shows that the hydrophobic nature of [C4min][NTf2] and [C6min][NTf2] increased the entrapment of water molecules when comparted to control samples (HY-control and IB-control). These results corroborate what was described by Souza et al. [29], whereby salts of a more hydrophobic nature promoted a stronger entrapment of water molecules linked to the silica-based matrix.
In addition, these results indicate that LIs act both for the contributions of support formation (most evident for [C6min][NTf2]), and for a synergistic effect (active in support formation and the reaction mechanisms), which is controlled by the length of the alkyl chain (in the latter case for [C4min][NTf2]). The data that support this analysis are based on the fact that [C4min][NTf2] remains present in the support after the biocatalyst preparation steps, in detriment to the [C6min][NTf2] that is leached after the hexane wash step (already discussed above). Therefore, the TG analysis confirms these observations, since a significant loss of mass is observed for HY-C4 (30.81%) compared to the HY-C6 sample (23.00%) in the region of from 200 to 400 °C (Fig. 4 and Table 3).
Mass losses in the region between 200 and 400 °C are associated with the presence of organic compounds present in PHBV, silica and IL (i.e., C, H, O and N). This included the lipase and the presence of non-reacted silanol groups of the TEOS, which are present in the silica because of incomplete sol–gel reactions [29, 44]. The substantial mass loss may also have occurred due to structural relaxation of the hybrid support caused by diffusional processes observed due to the difference between constituent types from temperature variation and removal of free volumes, as noted by Cabrera-Padilla et al. [30] for support samples prepared with PHBV. The mass loss between 400 and 600 ºC associated with carbonation and final dehydroxylation of organic compacts, leading to thermal stability of the material.
pH and temperature effect
The biochemical characterization of the IL modified hybrid IB was evaluated from buffer solutions containing different pH (4.0–8.0). Figure 5a presents the influence of pH variation on the relative activity of IB in the hybrid support. The immobilized BCL showed better hydrolytic activity at neutral pH (7.0), indicating that both the BCL immobilized on hybrid support modified with IL and Control had better hydrolytic activity at pH 7.0. At values close to pH 6.0 and 8.0, the relative activity of the biocatalysts was lower for all cases, but it is possible to observe a slight tendency for IBs immobilized with ILs to endure this pH range (pH 6.0, control = 75%, with HY-C2 reached 85% relative activity and at pH 8.0, control = 49%, with HY-C4 achieved 65%). This result shows that the presence of the IL offered protection to the immobilized enzyme when compared to IB-Control, allowing its use in higher pH ranges. Reduction in the enzymatic activity of IBs at more acid or alkaline pH may have caused alteration in protein conformation, affecting enzymatic activity [45, 46].
The influence of temperature on the hydrolysis reaction was also investigated for immobilized BCL on Control support and modified with IL (Fig. 5b). The maximum relative activity obtained for all studied IB occurred at 50 °C. It was observed that the presence of IL did not cause the optimum temperature displacement of the evaluated biocatalysts. Moreover, the same profile was presented by Cabrera-Padilla et al. [34] where immobilized lipase from Bacillus sp. using aprotic IL as a modifying agent in PHBV support was analyzed. Thus, these results can give consistency to the use of these biocatalysts in typical biocatalysts reactions, which usually operate in the 40–60 ºC range [46,47,48].
Thermal stability
The thermal stability of lipase immobilized on hybrid support modified with IL and without IL in the reaction of hydrolysis in olive oil was investigated, measuring the residual activity as a function of time at temperatures of 55 and 65 °C. The IB in IL modified hybrid support showed better thermal stability than the IB-Control for both studied temperatures (55 and 65 °C), as shown in Fig. 6a and b, respectively. The study found that the addition of IL as a support modifier influences the improvement of thermal stability (Fig. 6b), especially at 55 ºC, when compared to the control support. However, it is possible to observe a 20% reduction in enzyme activity, when compared to the initial activity of these biocatalysts (IB-C4 and IB-C6). The same profile was not observed for all IB studied at 65 °C, which showed a 40% drop in activity at the same reaction time. The effects of the system hydrophobic microenvironment, protecting the enzyme, may have caused this behavior. This structural modification may be associated with the synergism between the hydrophobic interactions of the hybrid support together with the greater hydrophobicity of the IL which restricts the movement of the lipase and thus avoids conformational changes and unfolding of the enzyme at higher temperatures [26, 49]. In combination, these properties may have collaborated to the increase of the lipase stability in this support. The literature reported the same thermal stability profile of lipase immobilized at elevated temperatures [34, 50]. Abdulla et al. [50] evaluated the influence of immobilized BCL in alginate and k-carrageenan hybrid matrix by crosslinking and entrapment in the hydrolysis reaction of p-nitrophenyl palmitate. In this study, it was noticed that the hybrid matrix used in the cross-linked immobilization can withstand temperatures up to 60 ºC and that it presents good thermal resistance, which comes as an important property in industrial applications. Martins et al. [26] presented the immobilized BCL by covalent binding in the same hybrid support under study (Silica and PHBV) modified with protic IL in the reaction of olive oil hydrolysis presenting a profile similar to the one noted in this work. The result was attributed to the use of IL as a support modifying agent, allowing an enhanced thermal stability for the hybrid support at 55 °C, once IL with higher alkyl chains have a greater hydrophobic character and may promote a hydration layer surrounding the enzyme, allowing better thermal stability for IB-C4 and IB-C6, as shown in Fig. 6a.
Operational stability
For the IBs reuse study, operational stability was evaluated in olive oil hydrolysis reactions in multiple operation cycles. The operational stability for the BCL reuse in different supports presented similar profiles for the IB-C4 and IB-C6 biocatalysts, which were reused eleven times, maintaining 50% of the initial activity. On the other hand, immobilized biocatalyst without IL showed stability for only six reuses, as shown in Fig. 7. According to the results seen in this study, the presence of IL favored the alteration in the support surface, enriching the lipases pores and increasing the catalysis environment. This is specifically noticeable when one considers the value of the immobilization yield of 169 ± 3% for the hybrid (with [C6min][NTf2]) when compared to the immobilized BCL on silica which is 77 ± 6% and BCL in PHBV which is 90 ± 3%. The same profile was presented in the study of Nyari et al. [47] using the same enzyme. The BCL was immobilized in polyurethane support and showed an increased activity of 32%, in relation to the initial activity of the enzyme during six continuous cycles, assigning this result to the type of support used, type of immobilization and to the reaction medium. Studies conducted by Wan et al. [46] presented the operational stability analysis for the lipase of Candida rugosa immobilized on hybrid inorganic supports in sulfonated macroporous resins and the activity of the immobilized lipase remained above 81.6% after ten repeated cycles. The results approached in this study can be attributed to the immobilization efficiency in the hybrid support, which presented high operational stability in relation to free lipase.
It is important to highlight that several studies presented in the literature show similar responses with the use of IL promoting increase in enzymatic stability and, consequently, increase in the reuse of IBs [32, 51]. Cabrera-Padilla et al. [30] reported 20 cycles of reuse for Bacillus sp. immobilized in PHBV by physical adsorption in the presence of aprotic IL and, in the absence, presented only 10 cycles. Barbosa et al. [51] also verified greater operational stability in the presence of the lipase additive immobilized on silica air-gel, which obtained 23 cycles for the immobilized biocatalyst in the presence of protic IL and 13 cycles for the biocatalyst immobilized in the absence of IL.
Solvent stability
The influence of different organic solvents on biocatalysts activity was studied using ethanol and cyclohexane considering various incubation times (up to 72 h) at 40 ºC. Figure 8a shows that in the presence of ethanol (log P −0.30—logarithm based on the P distribution coefficient of a solute between a solvent and water in a two-phase system), promotes a decrease of the BCL activity through incubation period (up to 72 h) for all the biocatalysts studied.
Solvents with log P < 2 break the hydration layer, what may lead to enzymatic inactivation [52]. For samples incubated with cyclohexane, the presence of IL on the hybrid support increased BCL stability compared to the control sample. Figure 8b indicates that IL prevents enzymatic deactivation by stabilizing the lipase. From Fig. 8b, one can observe that immobilized BCL stability increased in the first 7 h for all systems (about 75% for the control sample and about 100% for samples with IL). Cyclohexane (log P 3.34) is a more hydrophobic solvent than ethanol (log P −0.30), thus the former is expected to cause less impact on enzyme activity [53].
Transesterification reactions
In this study, twelve types of biocatalysts were tested (Fig. 9) in olive oil hydrolysis reaction, among them the two most efficient IB were IB-C4 and IB-C6. After selection of these two IB, they were applied to transesterification reactions under conventional heating using crude coconut oil and ethanol. Figure 9 shows the study of the effect of oil: ethanol molar ratio (1:7 (A), 1:9 (B), 1:10 (C), and 1:12 (D)) as a function of the reaction time (24, 48, 72, 96 and 120 h) at 40 °C for the different biocatalysts (IB-C4 and IB-C6) in the transesterification reaction. The best conversions of ethyl esters were presented from a comparative table with varying molar ratios (oil:ethanol) at the best times was given in Fig. 9e.
Conversion time of ethyl esters using BCL by covalent attachment. Experiments performed at 40 °C, 120 h reaction and stirring at 80 rpm at different molar ratios of oil/ethanol: 1: 7 (a), 1: 9 (b), 1: 10 (c), 1:12 (d) and conversion of ethyl esters at the best times and different molar ratios of oil/ethanol (e). Black bar—IB-Control; blue bar—IB-C4; red bar—IB-C6
Regarding the presence of IL [C4mim][NTf2] in the modification of the support, among the biocatalysts tested, the conversion of ethyl esters by IB-C4 and IB-C6 was higher than the ethyl esters converted by the IB-Control, ranging from 24 to 59%, for all investigated molar ratio and time. The highest conversion (59%) was for IB-C4 at the 1:7 (molar ratio of oil:ethanol) in 96 h of reaction. This increase in 2.27 fold is possibly due to modifications of the support with IL, which caused an increase in the surface area and pore volume of the hybrid support (Table 2), leading to greater availability of substrate access by the concentrated enzyme in the pores of support, and consequently, greater substrate accessibility to the enzyme active site [54]. The hydrophobic nature of the hybrid support has created a hydrophobic environment that may have induced the opening of the BCL cap structure, activating the enzyme and increasing its catalytic power. Moreover, these results confirm that the interfacial activation of the IB on the supports in the presence of IL [C4mim][NTf2] was strongly favored by the immobilization characteristics, highlighting the biocatalyst (IB-C4) as the most active and most promising in synthesis of ethyl esters of crude coconut oil and ethanol.
According to the literature, studies involving the production of ethyl esters from the Candida antarctica lipase transesterification reaction immobilized on macroporous acrylic resin with refined coconut oil are developed by the conventional method. Tupufia et al. [55] converted 80% ethyl esters using a molar ratio (1:3) of oil:ethanol, at 50 °C in 50 h and Ribeiro et al. [56] achieved the same values of conversion (80%) under the oil:ethanol (1:10) molar ratio conditions at 60 °C within 24 h. Assuming that the excess impurities (antioxidants, water, phospholipids, sterols) present in crude vegetable oils can negatively influence the transesterification reaction and also interfere with the process of esters and glycerol separation [57], the 59% yield of ethyl esters achieved in the present work is a favorable finding considering that coconut oil is crude and has not been refined, thus reducing the costs of the process.
Conclusions
The immobilization of the BCL on hybrid support, obtained from PHBV and silica, presented more promising results when compared to the isolated supports of origin. The synergism of IL use in the hybrid support preparation and BCL immobilization was controlled by the hydrophobic nature of IL, confirmed by TG analysis and FTIR spectra of immobilized supports.
The hybrid supports prepared with [C4min][NTf2] and [C6min][NTf2] show morphological characteristics such as increase in surface area and pore volume, resulting in an increase of 1.6-fold in yield of immobilization (Ya = 169 ± 3%), as also effect in activation interfacial of lipase by decrease α-helix content.
The biocatalysts immobilized on IL modified hybrid support ([C4min][NTf2] and [C6min][NTf2]) presented 11 reuses, maintaining more than 50% of their initial activity and the ideal temperature to reach the highest activity was 50 °C. The application of IBs (with [C4min][NTf2]) in the transesterification reactions of crude coconut oil and ethanol showed that the maximum conversion to ethyl esters was of 59%. These results confirm that the interfacial activation of the immobilized biocatalyst with [C4min][NTf2] hybrid support was strongly favored by the synergistic effect caused by the use of IL in its preparation and during the immobilization process. In addition, the data obtained in this work are encouraging, once that coconut oil has not undergone a refining process.
References
Ben Bacha A, Moubayed NMS, Al-Assaf A. An organic solvent-stable lipase from a newly isolated Staphylococcus aureus ALA1 strain with potential for use as an industrial biocatalyst. Biotechnol Appl Biochem. 2016;63(3):378–90. https://doi.org/10.1002/bab.1381.
Badgujar VC, Badgujar KC, Yeole PM, Bhanage BM. Immobilization of Rhizomucor miehei lipase on a polymeric film for synthesis of important fatty acid esters: kinetics and application studies. Bioprocess Biosyst Eng. 2017;40(10):1463–78. https://doi.org/10.1007/s00449-017-1804-0.
Barbosa MS, Freire CCC, Almeida LC, Freitas LS, Souza RL, Pereira EB et al. Optimization of the enzymatic hydrolysis of Moringa oleifera Lam oil using molecular docking analysis for fatty acid specificity. Biotechnol Appl Biochem. 2019. https://doi.org/10.1002/bab.1793.
Brady D, Jordaan J, Simpson C, Chetty A, Arumugam C, Moolman FS. Spherezymes: A novel structured self-immobilisation enzyme technology. BMC Biotechnol. 2008;8(1):8. https://doi.org/10.1186/1472-6750-8-8.
Ma L, Zhou L, Jiang Y, He Y, Wang L, Gao J. Lipase based static emulsions as efficient biocatalysts for biodiesel production. J Chem Technol Biotechnol. 2017;92(6):1248–55. https://doi.org/10.1002/jctb.5118.
Barsé LQ, Graebin NG, Cipolatti EP, Robert JM, Pinto MCC, Pinto JCCS, et al. Production and optimization of isopropyl palmitate via biocatalytic route using home-made enzymatic catalysts. J Chem Technol Biotechnol. 2019;94(2):389–97. https://doi.org/10.1002/jctb.5782.
Balcão VM, Paiva AL, Xavier MF. Bioreactors with immobilized lipases: State of the art. Enzyme Microb Technol. 1996;18(6):392–416. https://doi.org/10.1016/0141-0229(95)00125-5.
Kennedy JF, Cabral JMS. Enzyme Technology. In: Rehm HJ, Reed G, editors. Biotechnology—a comprehensive treatise. Weinheim: VCH Verlagsgesellscbaft; 1987. p. 347–404.
Kumar GS, Rather GM, Gurramkonda C, Reddy BR. Thermostable α-amylase immobilization: Enhanced stability and performance for starch biocatalysis. Biotechnol Appl Biochem. 2016;63(1):57–66. https://doi.org/10.1002/bab.1350.
Alves NR, Pereira MM, Giordano RLC, Tardioli PW, Lima ÁS, Soares CMF, et al. Design for preparation of more active cross-linked enzyme aggregates of Burkholderia cepacia lipase using palm fiber residue. Bioprocess Biosyst Eng. 2020. https://doi.org/10.1007/s00449-020-02419-0.
Padilha GS, Tambourgi EB, Alegre RM. Evaluation of lipase from Burkholderia cepacia immobilized in alginate beads and application in the synthesis of banana flavor (isoamyl acetate). Chem Eng Commun. 2018;205(1):23–33. https://doi.org/10.1080/00986445.2017.1370707.
Shuai W, Das RK, Naghdi M, Brar SK, Verma M. A review on the important aspects of lipase immobilization on nanomaterials. Biotechnol Appl Biochem. 2017;64(4):496–508. https://doi.org/10.1002/bab.1515.
Simões AS, Mori RY, Faria R, Castro HF, Mendes AA. Desempenho da matriz híbrida SiO2-quitosana na imobilização da lipase microbiana de Candida rugosa. Quím Nova. 2011;34:33–8. https://doi.org/10.1590/S0100-40422011000100007.
Zdarta J, Meyer SA, Jesionowski T, Pinelo M. A General overview of support materials for enzyme immobilization: Characteristics, properties, practical utility. Catalysts. 2018;8(2). doi:https://doi.org/10.3390/catal8020092.
Kołodziejczak-Radzimska A, Zdarta J, Ciesielczyk F, Jesionowski T. An organofunctionalized MgO·SiO2 hybrid support and its performance in the immobilization of lipase from Candida rugosa. Korean J Chem Eng. 2018;35(11):2220–31. https://doi.org/10.1007/s11814-018-0146-1.
Llerena Suster CR, Toledo MV, Fittipaldi AS, Morcelle SR, Briand LE. Lipase B of Candida antarctica co-adsorbed with polyols onto TiO2 nanoparticles for improved biocatalytic performance. J Chem Technol Biotechnol. 2017;92(11):2870–80. https://doi.org/10.1002/jctb.5305.
Gascón V, Castro-Miguel E, Díaz-García M, Blanco RM, Sanchez-Sanchez M. In situ and post-synthesis immobilization of enzymes on nanocrystalline MOF platforms to yield active biocatalysts. J Chem Technol Biotechnol. 2017;92(10):2583–93. https://doi.org/10.1002/jctb.5274.
Benvenutti EV, Moro CC, Costa TMH, Gallas MR. Silica based hybrid materials obtained by the sol-gel method. Quím Nova. 2009;32:1926–33. https://doi.org/10.1590/S0100-40422009000700039.
Nowacka M, Klapiszewski Ł, Norman M, Jesionowski T. Dispersive evaluation and surface chemistry of advanced, multifunctional silica/lignin hybrid biomaterials. Cent Eur J Chem. 2013;11(11):1860–73. https://doi.org/10.2478/s11532-013-0322-4.
Naushad M, Alothman ZA, Khan AB, Ali M. Effect of ionic liquid on activity, stability, and structure of enzymes: A review. Int J Biol Macromol. 2012;51(4):555–60. https://doi.org/10.1016/j.ijbiomac.2012.06.020.
Zhao H. Methods for stabilizing and activating enzymes in ionic liquids—a review. J Chem Technol Biotechnol. 2010;85(7):891–907. https://doi.org/10.1002/jctb.2375.
Gao J, Ndong RS, Shiflett MB, Wagner NJ. Creating nanoparticle stability in ionic liquid [C4mim][BF4] by inducing solvation layering. ACS Nano. 2015;9(3):3243–53. https://doi.org/10.1021/acsnano.5b00354.
Putz A-M, Len A, Ianăşi C, Savii C, Almásy L. Ultrasonic preparation of mesoporous silica using pyridinium ionic liquid. Korean J Chem Eng. 2016;33(3):749–54. https://doi.org/10.1007/s11814-016-0021-x.
Greaves TL, Drummond CJ. Protic ionic liquids: properties and applications. Chem Rev. 2008;108(1):206–37. https://doi.org/10.1021/cr068040u.
Karimi B, Tavakolian M, Akbari M, Mansouri F. Ionic liquids in asymmetric synthesis: an overall view from reaction media to supported ionic liquid catalysis. ChemCatChem. 2018;10(15):3173–205. https://doi.org/10.1002/cctc.201701919.
Martins SRS, dos Santos A, Fricks AT, Lima ÁS, Mattedi S, Silva DP, et al. Protic ionic liquids influence on immobilization of Lipase Burkholderia cepacia on hybrid supports. J Chem Technol Biotechnol. 2017;92(3):633–41. https://doi.org/10.1002/jctb.5044.
Paula AV, Moreira ABR, Braga LP, Castro HFd, Bruno LM. Comparative performance of Candida rugosa lipase immobilized on polysiloxane polyvinyl alcohol hybrid support using different methodologies. Quím Nova. 2008;31:35–40. https://doi.org/10.1590/S0100-40422008000100007
Soares CMF, De Castro HF, De Moraes FF, Zanin GM. Characterization and utilization of Candida rugosa lipase immobilized on controlled pore silica. Appl Biochem Biotechnol. 1999;79(1):745–57. https://doi.org/10.1385/ABAB:79:1-3:745.
Souza RL, Faria ELP, Figueiredo RT, Mettedi S, Santos OAA, Lima AS, et al. Protic ionic liquid applied to enhance the immobilization of lipase in sol–gel matrices. J Therm Anal Calorim. 2017;128(2):833–40. https://doi.org/10.1007/s10973-016-5950-4.
Cabrera-Padilla RY, Lisboa MC, Pereira MM, Figueiredo RT, Franceschi E, Fricks AT, et al. Immobilization of Candida rugosa lipase onto an eco-friendly support in the presence of ionic liquid. Bioprocess Biosyst Eng. 2015;38(5):805–14. https://doi.org/10.1007/s00449-014-1322-2.
Cabrera-Padilla RY, Lisboa MC, Fricks AT, Franceschi E, Lima AS, Silva DP, et al. Immobilization of Candida rugosa lipase on poly(3-hydroxybutyrate-co-hydroxyvalerate): a new eco-friendly support. J Ind Microbiol Biot. 2012;39(2):289–98. https://doi.org/10.1007/s10295-011-1027-3.
Freitas L, Da Rós PCM, Santos JC, de Castro HF. An integrated approach to produce biodiesel and monoglycerides by enzymatic interestification of babassu oil (Orbinya sp). Process Biochem. 2009;44(10):1068–74. https://doi.org/10.1016/j.procbio.2009.05.011.
Fan Y, Wu G, Su F, Li K, Xu L, Han X, et al. Lipase oriented-immobilized on dendrimer-coated magnetic multi-walled carbon nanotubes toward catalyzing biodiesel production from waste vegetable oil. Fuel. 2016;178:172–8. https://doi.org/10.1016/j.fuel.2016.03.071.
Cabrera-Padilla RY, Melo EB, Pereira MM, Figueiredo RT, Fricks AT, Franceschi E, et al. Use of ionic liquids as additives for the immobilization of lipase from Bacillus sp. J Chem Technol Biotechnol. 2015;90(7):1308–16. https://doi.org/10.1002/jctb.4438.
Almeida RM, Marques AC. Characterization of Sol-Gel materials by infrared spectroscopy. In: Klein L, Aparicio M, Jitianu A, editors. Handbook of Sol-Gel Science and Technology: Processing, Characterization and Applications. Cham: Springer International Publishing; 2018. p. 1121–51.
Chemspider. The free chemical database. http://www.chemspider.com. 03 January 2020.
Sánchez DA, Tonetto GM, Ferreira ML. Burkholderia cepacia lipase: A versatile catalyst in synthesis reactions. Biotechnol Bioeng. 2018;115(1):6–24. https://doi.org/10.1002/bit.26458.
Qin J, Zou X, Lv S, Jin Q, Wang X. Influence of ionic liquids on lipase activity and stability in alcoholysis reactions. RSC Adv. 2016;6(90):87703–9. https://doi.org/10.1039/C6RA19181A.
Pan S, Liu X, Xie Y, Yi Y, Li C, Yan Y, et al. Esterification activity and conformation studies of Burkholderia cepacia lipase in conventional organic solvents, ionic liquids and their co-solvent mixture media. Bioresource Technol. 2010;101(24):9822–4. https://doi.org/10.1016/j.biortech.2010.07.107.
Barbosa MS, Santos AJ, Carvalho NB, Figueiredo RT, Pereira MM, Lima ÁS, et al. Enhanced activity of immobilized lipase by phosphonium-based ionic liquids used in the support preparation and immobilization process. ACS Sustain Chem Eng. 2019;7(18):15648–59. https://doi.org/10.1021/acssuschemeng.9b03741.
Meera KMS, Sankar RM, Jaisankar SN, Mandal AB. Mesoporous and biocompatible surface active silica aerogel synthesis using choline formate ionic liquid. Colloid Surface B. 2011;86(2):292–7. https://doi.org/10.1016/j.colsurfb.2011.04.011.
Guisan JM. Immobilization of Enzymes and Cells. 2nd ed. Totowa-New Jersey: Humana Press; 2006.
Souza RL, Faria ELP, Figueiredo RT, Freitas LdS, Iglesias M, Mattedi S et al. Protic ionic liquid as additive on lipase immobilization using silica sol–gel. Enzyme Microb Technol. 2013;52(3):141–50. https://doi.org/10.1016/j.enzmictec.2012.12.007.
Mukherjee I, Mylonakis A, Guo Y, Samuel SP, Li S, Wei RY, et al. Effect of nonsurfactant template content on the particle size and surface area of monodisperse mesoporous silica nanospheres. Micropor Mesopor Mat. 2009;122(1):168–74. https://doi.org/10.1016/j.micromeso.2009.02.030.
Zou B, Song C, Xu X, Xia J, Huo S, Cui F. Enhancing stabilities of lipase by enzyme aggregate coating immobilized onto ionic liquid modified mesoporous materials. Appl Surf Sci. 2014;311:62–7. https://doi.org/10.1016/j.apsusc.2014.04.210.
Wan D, Tian L, Li X, Li B, Zhang Q. A versatile strategy for enzyme immobilization: Fabricating lipase/inorganic hybrid nanostructures on macroporous resins with enhanced catalytic properties. Biochem Eng J. 2018;139:101–8. https://doi.org/10.1016/j.bej.2018.08.010.
Nádia Ligianara Dewes N, Alessandro Rogério P, Clarice S, Marcelo M, Jamile Z, Rogério Marcos D. Optimization of olive oil hydrolysis process using immobilized lipase from Burkholderia cepacia sp. in Polyurethane. Acta Sci-Technol. 2017;39(4). doi:https://doi.org/10.4025/actascitechnol.v39i4.30770.
Carvalho BN, Vidal TB, Barbosa SA, Pereira MM, Mattedi S, Freitas DSL et al. Lipase immobilization on silica xerogel treated with protic ionic liquid and its application in biodiesel production from different oils. Int J Mol Sci. 2018;19(7). doi:https://doi.org/10.3390/ijms19071829.
Izrael Živković LT, Živković LS, Babić BM, Kokunešoski MJ, Jokić BM, Karadžić IM. Immobilization of Candida rugosa lipase by adsorption onto biosafe meso/macroporous silica and zirconia. Biochem Eng J. 2015;93:73–83. https://doi.org/10.1016/j.bej.2014.09.012.
Abdulla R, Ravindra P. Characterization of cross linked Burkholderia cepacia lipase in alginate and κ-carrageenan hybrid matrix. J Taiwan Inst Chem Eng. 2013;44(4):545–51. https://doi.org/10.1016/j.jtice.2013.01.003.
Barbosa AS, Lisboa JA, Silva MAO, Carvalho NB, Pereira MM, Fricks AT, et al. The novel mesoporous silica aerogel modified with protic ionic liquid for lipase immobilization. Quím Nova. 2016;39:415–22. https://doi.org/10.5935/0100-4042.20160042.
Pizarro C, Brañes MC, Markovits A, Fernández-Lorente G, Guisán JM, Chamy R, et al. Influence of different immobilization techniques for Candida Cylindracea lipase on its stability and fish oil hydrolysis. J Mol Catal B Enzym. 2012;78:111–8. https://doi.org/10.1016/j.molcatb.2012.03.012.
Martínez-Aragón M, Burghoff S, Goetheer ELV, de Haan AB. Guidelines for solvent selection for carrier mediated extraction of proteins. Sep Purif Technol. 2009;65(1):65–72. https://doi.org/10.1016/j.seppur.2008.01.028.
Cui JD, Jia SR. Optimization protocols and improved strategies of cross-linked enzyme aggregates technology: current development and future challenges. Crit Rev Biotechnol. 2015;35(1):15–28. https://doi.org/10.3109/07388551.2013.795516.
Tupufia SC, Jeon YJ, Marquis C, Adesina AA, Rogers PL. Enzymatic conversion of coconut oil for biodiesel production. Fuel Process Technol. 2013;106:721–6. https://doi.org/10.1016/j.fuproc.2012.10.007.
Ribeiro BD, Coelho MAZ, Barreto DW. Production of concentrated natural beta-carotene from buriti (Mauritia vinifera) oil by enzymatic hydrolysis. Food Bioprod Process. 2012;90(2):141–7. https://doi.org/10.1016/j.fbp.2011.02.003.
Michelin S, Penha FM, Sychoski MM, Scherer RP, Treichel H, Valério A, et al. Kinetics of ultrasound-assisted enzymatic biodiesel production from Macauba coconut oil. Renew Energy. 2015;76:388–93. https://doi.org/10.1016/j.renene.2014.11.067.
Acknowledgements
This study ws financed in part by the Coordenação de Aperfeiçoamento de Pessoal de Nível Superior—Brasil (CAPES)—Finance Code 001; and Conselho Nacional de Desenvolvimento Científico e Tecnológico (CNPq).
Author information
Authors and Affiliations
Corresponding author
Ethics declarations
Conflicts of interest
The authors have no conflicts of interest to declare that are relevant to the content of this article.
Additional information
Publisher's Note
Springer Nature remains neutral with regard to jurisdictional claims in published maps and institutional affiliations.
Rights and permissions
About this article
Cite this article
Martins, S.R.S., Andrade, S.M.S., Fricks, A.T. et al. Ionic liquid synergistic effect between preparation of hybrid supports and immobilization of lipase applied to esters production. J Therm Anal Calorim 147, 1143–1156 (2022). https://doi.org/10.1007/s10973-020-10408-4
Received:
Accepted:
Published:
Issue Date:
DOI: https://doi.org/10.1007/s10973-020-10408-4