Abstract
Changes in zooplankton microhabitat use in lakes through diel migrations (vertical and horizontal) have been related to habitat heterogeneity and to predation pressure, among other factors. However, there is a controversy concerning the effectiveness of diel migrations in temporary ponds, due to the shallowness of these systems and their distinct aquatic predator communities (mainly macroinvertebrates and amphibians). In order to test if diel microhabitat selection patterns described from lakes hold in temporary ponds, we developed a study using funnel traps at different zones of the pond (high and low vegetation density; surface and bottom of the pond) that were checked during the day and at night. Additionally, we assessed predation risk by sampling the macroinvertebrate community at the same time. In the studied Mediterranean temporary pond, zooplankton exhibited diel patterns of microhabitat selection: cladocerans showed a diel horizontal pattern, whereas copepods showed a diel vertical pattern. Results suggest that microhabitat selection and the associated diel pattern may be explained by both biological (potential predation) and environmental drivers (habitat heterogeneity and protection against ultraviolet radiation).
Similar content being viewed by others
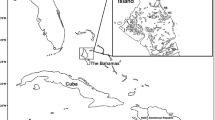
Avoid common mistakes on your manuscript.
Introduction
The conservation interest of temporary ponds is being increasingly recognized, with recent studies reporting high levels of floral and faunal diversity (e.g. Boix et al., 2001; Bagella & Caria, 2012; Rouissi et al., 2014). The singularity of these biotas is explained by the environmental constraints imposed by the alternate phases of drought and flooding; as a consequence, species are adapted to short cycles and a high variability in hydroperiod length (Grillas et al., 2004). However, biological (e.g. predation) and environmental factors other than hydroperiod length (e.g. habitat heterogeneity) also have major influences on faunal community structure in these ecosystems, and may differ from those prevalent in permanent ponds (Wellborn et al., 1996).
In lakes, zooplankton spatial structure depends on habitat heterogeneity and predation, among other factors such as light, temperature, dissolved oxygen, and pH (e.g. Timms & Moss, 1984; Lampert, 1993; Schriver et al., 1995; Lauridsen & Buenk, 1996; Burks et al., 2001a, 2002). Zooplankton is known to seek refuge in structurally complex habitats such as macrophytic beds, or in dark deep habitats, to avoid fish predation. However, zooplankton resources (mainly phytoplankton) are found in structurally simple habitats (i.e. macrophyte-free or with low macrophyte densities), or near the pond surface. Thus, zooplankton is usually found in vegetated habitats or at depth during the day to avoid visual predators, migrating to resource-rich open waters or near the surface at night. Therefore, freshwater zooplankton can show two different diel patterns: (i) diel horizontal patterns of microhabitat selection (DHMS) between macrophytic refuge and open waters; and (ii) diel vertical patterns of microhabitat selection (DVMS) between deep and surface waters. However, the generality of this framework is under discussion. Some recent studies have pointed out that in lakes with small zooplanktivorous fishes, these predators often show an affinity for macrophytic habitats, and therefore macrophytes are a poor refuge to zooplankton (Meerhoff et al., 2006; Meerhoff et al., 2007a; González-Sagrario & Balseiro, 2010). This could also apply to temporary ponds, since they present different abiotic (i.e. shallowness of the basin) and biotic characteristics (i.e. different main predators) than most lakes.
High zooplankton predation risk has sometimes been reported from temporary and ephemeral ponds (Brendonck et al., 2002; Boix et al., 2006), but there is a consensus that temporary environments generally present lower predation risk than permanent habitats (Schneider & Frost, 1996; Wellborn et al., 1996). In permanent freshwaters, top predators are usually fishes, but the dry phase of temporary ponds normally excludes this group (although some exceptions exist; e.g. Laufer et al., 2009; Pinceel et al., 2015). In contrast, some amphibian and macroinvertebrate predators are adapted to the alternating dry and aquatic phases of temporary ponds (Boix et al., 2006; Blaustein et al., 2014). Therefore, the role that top predator amphibians and macroinvertebrates play in temporary waters may be analogous to that of fish in permanent ponds. However, some studies (Zimmer et al., 2001; Brucet et al., 2010) suggest that predation effects of top predator amphibians and macroinvertebrates on zooplankton may differ from effects of fish, as measured by species composition, size diversity, and zooplankton biomass.
It has been described that many macroinvertebrates, including predators, show high affinities for macrophytic habitats (Della Bella et al., 2005; Bazzanti et al., 2010), which implies that vegetated areas are not always predation-free (MacIsaac & Hutchinson, 1985; González-Sagrario & Balseiro, 2003). As a consequence, a relatively higher structural complexity of macrophytes in temporary habitats may not offer valuable refuge to zooplankton, similarly to what has been observed in lakes with small zooplanktivorous fishes that have high affinities for macrophytes (Meerhoff et al., 2007a; González-Sagrario & Balseiro, 2010). Recent studies report diel horizontal migrations of zooplankton in fishless permanent and temporary ponds with invertebrate predators (e.g. Turbellaria, Notonectidae, Chaoborus; Gilbert & Hampton, 2001; Trochine et al., 2009; Riemer, 2012). Overall, the effectiveness of zooplankton DHMS to avoid predation in temporary ponds is still controversial.
This controversy also affects DVMS, as several studies suggest that in temporary ponds, diel vertical migrations and DVMS may be less efficient due to reduced depths (relative to lakes) that allow light to reach the bottom of the pond (Jeppesen et al., 1997; Burks et al., 2002). However, other studies have found that shallow lakes (with and without fish) and temporary ponds can be inhabited by zooplankton groups that perform diel vertical migrations and DVMS, in accordance with the lake framework (Gilbert & Hampton, 2001; Cerbin et al., 2003, Alajärvi & Horppila, 2004; Trochine et al., 2009).
Here, we investigated if diel microhabitat selection in temporary ponds may be used by zooplankton as a strategy to reduce predation pressure in these ecosystems. We carried out a study in a Mediterranean temporary pond where macroinvertebrates are the main predator group. We focused the study on two major factors: habitat (high vs. low density of macrophytes) and depth (surface vs. bottom of the pond). We used funnel traps to test if zooplankton distribution differed among habitats, depths and time of the day. We predicted that (i) the distribution of zooplankton would be generally explained by the distribution of macrophytes, with zooplankton avoiding macrophytes habitat to reduce predation risk. We also predicted that (ii) zooplankton would not show a clear diel microhabitat selection, since temporary ponds are probably too shallow to allow DVMS and predator-rich macrophytes are unlikely to provide refuge to zooplankton (thus defeating the purpose of DHMS). Additionally, as body size determines trophic interactions (Compte et al., 2012), we expected that community parameters based on body size would be affected by variation in microhabitat selection. Size diversity has been proposed as an estimate of body-size structure (Quintana et al., 2008), and previous studies have suggested that size diversity is more sensitive to biological interactions than community parameters that rely on species composition (Badosa et al., 2007; Quintana et al., 2015).
Materials and methods
Study site
The study took place in a temporary pond located on the Empordà plain (near Albera mountain range), in the NE Iberian Peninsula (42º23′45′′N, 2º54′35′′E) (Fig. 1). The climate at the study site is Mediterranean, with rainy periods occurring in autumn and spring, hot summers and mild winters. The hydroperiod usually begins by late autumn and ends at late spring (Ribera et al., 1994; Font & Vilar, 1998). The pond is located at 172 m a.s.l., has a maximum size of 0.59 ha and a maximum depth of 1.2 m. Two habitats were identified according to macrophyte density: a high macrophyte-density habitat (HM; 1.31 g dry weight m−2) in the centre and a low macrophyte-density habitat (LM; 0.31 g dry weight m−2), in the littoral zone. LM habitats were shallower than HM ones (LM = 0.5 m, HM = 1 m). The most abundant plant species in both habitats were submerged: Ranunculus aquatilis L., Ranunculus ophioglossifolius Vill., Persicaria amphibia (L.) Gray and Schoenoplectus lacustris (L.) Palla.
Sketch map of the study site and distribution of sampling traps in the pond. Twenty funnel traps were fixed at different depths (pond surface and bottom) and habitats of the pond (low macrophyte-density littoral habitat, LM; high macrophyte-density central habitat, HM). PNA: Natural Zone of Declared National Interest of the Albera
Sampling design
The study took place in April 2011. Before installing the funnel traps setup, we measured water temperature (°C), conductivity (mS cm−1) and dissolved oxygen (%) in situ in each zone of the pond: HM-surface, HM-bottom, LM-surface and LM-bottom. We installed twenty funnel traps in the pond to capture zooplankton. The traps were similar to those used in studies about microcrustaceans dynamics in lakes (Whiteside, 1974; Beladjal et al., 1992; Tremel et al., 2000). Each trap was a 500-ml transparent plastic container (30 cm length, 8 cm diameter) equipped with an inverse-funnel opening (2 cm diameter). We placed a set of traps at different depths by attaching the traps to an iron bar fixed to the pond bottom in order to study DVMS: one trap was fixed at 10 cm below the water surface, and the other at 10 cm above the bottom of the pond (Fig. 1). To study DHMS, we installed sets of traps at 5 LM sampling points and at 5 HM sampling points. Traps were active during two 10-h periods and were always oriented following the same direction: the traps in LM were oriented towards the centre of the pond, and the traps in HM were oriented towards the littoral. The first period (from 10 a.m. to 8 p.m) was used to detect the position of zooplankton during the day, and the second period (from 10 p.m. to 8 a.m.) was used to detect the position of zooplankton at night. We repeated the study three times, spaced 3 days each to reduce sampling disturbance effects. Traps were uninstalled and installed again at the same site for each run. All runs were done under similar weather conditions (sunny weather). After each 10-h period (1 h after dawn and dusk), we preserved the zooplankton captured in the traps in ethanol 96%. We subsequently counted, measured and identified the individuals using a stereomicroscope and a microscope. We identified each zooplankton specimen to species level, except for rotifers (identified to genus level), copepodites (identified to order: calanoids, cyclopoids or harpacticoids) and nauplii (not identified further). We considered the different developmental stages of copepods as separated items.
We sampled macroinvertebrates and amphibians to quantify spatial and temporal variation in predation risk. We collected five samples at each zone (HM-surface, HM-bottom, LM-surface and LM-bottom), next to where the traps were installed, at 11 a.m. and 11 p.m. on days following the zooplankton sampling. The sampling procedure consisted of a 3-m transect using a 22-cm diameter dip net (250-μm mesh size). We stored and counted the macroinvertebrates and amphibian larvae to obtain densities (individuals m−2). Using a stereomicroscope, we identified amphibians to species level, whereas macroinvertebrates were identified to family level, except for Dytiscidae and Chironomidae (identified to subfamily level), and Turbellaria (not identified further). No adult amphibians were killed; instead, they were identified, measured and released immediately. We only considered predator macroinvertebrates (classified after Tachet et al., 2000; Monakov, 2003) in our analyses.
Data analysis
First, to evaluate differences in physical and chemical parameters between day and night in each pond habitat (HM or LM) and depth (bottom or surface), we performed analyses of variance (ANOVAs). We used ANOVAs because only one replicate per zone was available, and we considered the different sampling days as replicates. Whenever significant results were obtained, we performed Tukey post hoc multiple comparison tests to identify differences between zones and between day and night. ANOVAs and post hoc tests were performed using SPSS 15.
Second, we tested whether the abundance of each zooplankton taxa and each macroinvertebrate predator family differed between day and night, for each pond depth and habitat. We also analysed the variation of zooplankton abundance, grouping them in five functional groups: calanoids, cyclopoids, harpacticoids, cladocerans (all cladocerans present in the pond belong to the phytophilous functional group) and microfilterers (rotifers and nauplii). We used linear mixed-effects models (LME) to test for these differences. LME models are recommended when dealing with repeated measures in ecological studies, presenting several advantages over repeated measures ANOVA (Wang & Goonewardene, 2004; Wainwright et al., 2007; Ma et al., 2012). These models allow a greater flexibility in modelling covariance structures, a better capability to handle missing observations and are more efficient when the number of samples and measures across times are small. In order to avoid pseudoreplication, we considered sampling day as a random effect. The different habitats, depths and times (day or night), as well as double interactions (habitat-time and depth-time), were included as explanatory variables (fixed effects). Interaction terms were interpreted first, and the main factors were only interpreted if they were not included in a significant interaction. Thus,
-
(1)
a significant habitat-time interaction (meaning that the horizontal distribution of a taxa differed over time) was interpreted as different DHMS.
-
(2)
a significant depth-time interaction (meaning that the vertical distribution of a taxa differed over time) was interpreted as different DVMS.
-
(3)
no significant interaction (meaning that the distribution of a taxa was invariant over time) combined with habitat factor being significant was interpreted as microhabitat selection differing between HM and LM.
-
(4)
no significant interaction combined with the depth factor being significant was interpreted as microhabitat selection differing between surface and bottom.
-
(5)
no significant interaction combined with neither habitat and depth factors being significant was interpreted as no microhabitat selection.
We performed LME models using the “lme” function in the nlme package (Pinheiro et al., 2007), written in R (R Development Core Team, 2014). Species with low abundance (total abundance <100 individuals) were not included in the analyses of diel patterns and microdistribution.
Finally, to assess the microhabitat selection at the community level we calculated the following community parameters: zooplankton diversity, size diversity, zooplankton richness and total abundance. We estimated the zooplankton diversity with the Shannon–Wiener index (Pielou, 1969) using abundances. We determined size diversity with the Kernel estimation (Quintana et al., 2008) using the free software available at www.limnolam.org. We calculated total abundance as the sum of all organisms’ abundances in a given sample. To calculate size diversity we used biomass, which is estimated using published length–weight relationships: Malley (1989) for copepods (nauplii, copepodites and adults), cladocerans and the rotifer Keratella sp. Bory de St. Vincent, 1822; Dumont et al. (1975) for Simocephalus vetulus (Müller, 1776) and Ruttner-Kolisko (1977) for other rotifers. To check for differences in parameters between day and night for each depth and habitat sampled, we used further LME models following the same procedure described above.
Results
Conductivity was similar across all pond habitats and depths (Table 1). Temperature was similar in all zones, although it was significantly higher at night in surface and LM zones, than in HM in bottom zones during either the day or at night. Dissolved oxygen was similar across all pond zones except for HM in bottom zones, where it was significantly lower than in the rest of zones during both day and night.
Fourteen zooplankton taxa were identified, including five copepods, five cladocerans and four rotifers. Nauplii and three copepodite orders (calanoids, cyclopoids or harpacticoids) were also identified as different taxa. However, Hemidiaptomus roubaui (Richard, 1888), Testudinella sp. Bory de St. Vincent, 1826 and Brachionus sp. Pallas, 1776 were not included in the analyses of diel patterns of microhabitat selection due to their low abundances.
Zooplankton taxa were classified into four groups according to their microhabitat selection (Table 2). The first group (nauplii, Canthocamptus staphylinus (Jurine, 1820), Acanthocyclops americanus (Marsh, 1892), Chydorus sphaericus (Müller, 1776), Coronatella rectangula (Sars, 1862) and Hexarthra sp. Schmarda, 1854) did not show a diel pattern but had differences in habitat preferences. The rotifer Hexarthra sp. and A. americanus were distributed similarly throughout the pond, indicating a lack of microhabitat preference. In contrast, nauplii and C. rectangula were more abundant in surface and LM zones. Instead, C. sphaericus was more abundant at the bottom and C. staphylinus was more abundant in HM in bottom zones. The second group (Table 2) showed DVMS and was only represented by copepods (copepodites of calanoids and harpacticoids, Mixodiaptomus kupelwieseri (Brehm, 1907) and Diacyclops bicuspidatus (Claus, 1857)). These taxa were mainly located at the bottom of the pond during the day, and were mainly observed at the surface (copepodites of calanoids, M. kupelwieseri and D. bicuspidatus) or across all zones (copepodites of harpacticoids) during the night. The third group (Table 2) presented DHMS. This group was composed of cladocerans (S. vetulus and Tretocephala ambigua (Lilljeborg, 1901)), cyclopoid copepodites and the rotifer Keratella sp. During the day, cladocerans and Keratella sp. were located in the LM, and copepodites of cyclopoids were equally found in LM and HM. However, at night all taxa in this group were mainly observed in the HM. Finally, the cladoceran Alonella excisa (Fischer, 1854) showed a combination of vertical and horizontal changes in distribution. This planktonic taxa was relatively more abundant in LM and surface zones during the day, whereas at night was similarly found at both depths in the HM.
When zooplankton were analysed in functional groups, results were similar (Table 3). Microfilterers were found in surface but they did not present diel patterns of habitat selection. Calanoids showed DVMS, while cladocerans displayed DHMS. When all cyclopoids were grouped, they showed both DVMS and DHMS. During the day they were mostly found in LM and depth, while at night they preferred HM and surface. Finally, when all harpacticoids were grouped they showed a triple interaction, where during the day they were found in HM and depth, and at night they inhabited in HM throughout all the water column.
The main predators of the pond were macroinvertebrates, although larvae and adults of the marbled newt Triturus marmoratus (Latreille, 1800) were found as well (mean ± SD individuals per transect = 1.45 ± 2.76). Microturbellarians were the most abundant predator, followed by Libellulidae, Hydroporinae and Tanypodinae. Other coleopterans (Dytiscinae, Colymbetinae, Gyrinidae, Hygrobiidae and Hydrophilidae), heteropterans (Corixidae, Notonectidae and Naucoridae) and odonates (Lestidae and Aeshnidae) were present as well, though in lower densities. None of the predator taxa showed diel patterns of microhabitat selection. Therefore, we considered all predators as a single group. Overall, predators were more abundant in the HM than in the LM (LME, F 1,115 = 16.37; P < 0.01) and at the bottom than near the surface (LME, F 1,115 = 152.50; P < 0.01) (Fig. 2).
Mean abundance of predator taxa for each habitat and depth. A high macrophyte-density central habitat (HM) and low macrophyte-density littoral habitat (LM); B surface and bottom. Other predators category includes: Dytiscinae, Colymbetinae, Gyrinidae, Hygrobiidae, Hydrophilidae, Corixidae, Notonectidae, Naucoridae, Aeshnidae, Lestidae and amphibians
Finally, zooplankton community parameters differed significantly among zones (Fig. 3). Zooplankton diversity and richness were higher in the HM than LM, while size diversity was higher at the surface (coinciding with lower predator densities) than at the bottom. Total abundance was the only parameter that differed among zones and times, being higher in the LM during the day and in the HM at night (Fig. 3).
Box plots showing variation in community parameters (A zooplankton diversity, B zooplankton richness, C total abundance, D size diversity) across pond depths (surface and bottom), habitats (low macrophyte-density littoral habitat, LM; and high macrophyte-density central habitat, HM) and times (day and night). The results of the LME and median, quartiles and data outside the 10th and 90th percentiles are also shown
Discussion
Our study shows that in Mediterranean temporary ponds, zooplankton can exhibit diel patterns of microhabitat selection to reduce exposure to predators. Therefore, our results confirm the existence of diel migrations (horizontal and vertical) in temporary ponds, although these migrations are different from those observed in other shallow lakes and temporary ponds.
We found that most cladocerans and the rotifer Keratella sp. presented a DHMS, and in the case of cladocerans, this DHMS was opposite to that reported from shallow lakes or temporary ponds elsewhere. During the day, cladocerans and Keratella sp. avoided areas of high macrophyte densities but at night they were more abundant in these habitats. The microhabitat selection we observed is likely a strategy to avoid predators that are common in macrophytic habitats. Predation risk is probably high in these habitats (MacIsaac & Hutchinson, 1985; González-Sagrario & Balseiro, 2003) due to high abundances of microturbellarians and visual predators in our case. Some turbellarians can detect prey using non-visual signals (such as hydrodynamic signals, Trochine et al., 2005), making macrophytes a poor structural refuge for prey. For example, Pickavance (1971) found that the prey capture efficiency of Girardia tigrina (Girard, 1850) was not strongly affected by the presence of a structural refuge (e.g. leaves), while De Silva (1976) and MacIsaac & Hutchinson (1985) found that zooplankton predation rates of Mesostoma lingua (Abildgaard, 1789) and Dendrocoelum lacteum (Müller, 1774) actually increased in more complex sites (e.g. areas with plants). Additionally, visual predators associated with high macrophyte-density zones, such as newts and dragonflies (Martin et al., 1974; Crespo, 2011), are inefficient at capturing cladocerans in vegetated habitats (Burks et al., 2001b). This would explain why cladocerans and Keratella sp. were mainly present in the LM during the day, as they were able to find refuge in the bare sediment of the LM habitat and migrate to macrophytic habitats at night in search of resources (Burks et al., 2002). The preference of open sediment habitats by cladocerans has been described from subtropical lakes (see Meerhoff et al., 2007b) and in laboratory experiments (using species characteristic of Mediterranean wetlands; Tavşanoğlu et al., 2012). Finally, DHMS by zooplankton could owe to the fact that in ponds with transparent water and emergent vegetation may not provide sufficient refuge from predators (Nurminen & Horppila, 2002; Romare et al., 2003). However, this did not seem to be the case in our study, since submerged plants (mainly Ranunculus spp.) dominated vegetation communities of the studied pond.
The fact that only cladocerans, cyclopoid copepodites and the rotifer Keratella sp. presented DHMS may be explained by different responses among different zooplankton groups to microturbellarians, the most abundant predator group. Microturbellarians may have greater effects on cladocerans than copepods due to their relatively low efficiency in capturing copepods in macrophytic habitats as well as a higher effect of their neurotoxins on cladocerans (Schwartz & Hebert, 1986; Rocha et al., 1990; Blaustein & Dumont, 1990 and references therein; Trochine et al., 2005, 2006). This could explain why cladocerans, but not copepods, responded to the presence of microturbellarians through habitat selection. However, cyclopoid copepodites did present DHMS. It has been described that predation by the turbellarian Mesostoma ehrenbergii (Focke, 1836) on females of the cyclopoid Acanthocyclops robustus (Sars, 1863) may be similar in habitats with and without macrophyte structure, but lower under intermediate levels of macrophyte density (Trochine et al., 2006). Therefore, macrophytes are likely not a good refuge from predation for cyclopoids. Accordingly, cyclopoid copepodites did not show habitat selection during the day; however, they migrated to HM habitats at night, when visual predators are less effective and hence predation pressure is lower. In the case of Keratella sp., diel horizontal migrations to avoid predation have been previously described from subtropical lakes (José de Paggi et al., 2012): during the day Keratella may be found in vegetated areas but may move to open waters at night. Since in our study potential predation pressure was higher in HM, we suggest that Keratella showed an “inverse” diel horizontal pattern of microhabitat selection to minimize exposure to predators.
We detected DVMS for some copepods (mainly calanoids), similar to the diel vertical distributions described from shallow lakes and other temporary ponds where macroinvertebrates are the dominant predators (e.g. Gilbert & Hampton, 2001; Trochine et al., 2009). The observed DVMS could be explained by two non-mutually exclusive causes: predation and ultraviolet radiation. With regards to predation, predator cues in the water can trigger diel vertical migration as a defence mechanism against predation (Dawidowicz et al., 1990; Neill, 1990). Therefore, even if predators were mainly macrophyte-dwelling macroinvertebrates, and the pond was not deep enough to provide suitable refuge, copepods could perform DVMS as a response to such predator cues. Another plausible explanation is that copepods may need to reduce exposure to harmful ultraviolet radiation (Williamson et al., 2011). Recent studies have suggested that during daylight hours, marine and freshwater zooplankton may move to greater depths to avoid ultraviolet radiation and then back to the surface at night (Boeing et al., 2004; Williamson et al., 2011). Tolerance of ultraviolet radiation may differ among zooplankton groups (Leech & Williamson, 2000). The fact that high sensitivity to UV radiation has been reported in some calanoid species (Williamson et al., 1994) could explain why calanoid copepods exhibited DVMS in our experiment. Both mechanisms (predation and ultraviolet radiation) may be mediated by water turbidity (e.g. Nurminen et al., 2008). Although we did not measure this variable, qualitative observations throughout our study suggest that the water transparency was high since the bottom of the pond was always visible and water was visually transparent.
Total abundance was the only community parameter that differed between day and night, coinciding with the microhabitat selection of cladocerans. Zooplankton diversity and richness were higher in HM throughout all samples, which could be caused by higher resource availability (bacterioplankton or periphyton) in the macrophytic habitat (Burks et al., 2002). Changes in zooplankton diversity, richness and total abundance could be explained by the afore-mentioned environmental factors (resource and refuge) but also by biological interactions (predation). Size diversity did not change over time, but it was dependent on water depth, coinciding with predation pressure (size diversities were higher at the surface, where predation risk was lower). This result agrees with previously reported negative relationships between predation pressure and zooplankton size diversity (Brucet et al., 2010). Our study suggests that size diversity may be more sensitive to biological interactions than parameters such as taxa diversity (as observed by Badosa et al., 2007).
In summary, our study provides evidence that diel patterns of microhabitat selection by zooplankton may exist in Mediterranean temporary ponds. These patterns may emerge as a response to stressors that differ partially from those described from permanent freshwaters, shallow lakes and temporary ponds elsewhere. In our case, cladocerans were the group most susceptible to predation and they showed a clear horizontal change in microhabitat selection. Cyclopoids were relatively less susceptible to predation, and showed diverse microhabitat selection strategies. Finally, calanoids were the group least susceptible to predation and they did not show any DHMS. However, they showed a clear vertical change in microhabitat selection that could be related to avoidance of UV radiation. While cladocerans showed an “inverse” DHMS, as described in previous studies, copepods showed a DVMS similar to that observed in other aquatic habitats. It is also interesting to note that because macrophytes did not provide refuge to zooplankton, the positive effects of aquatic plants on water transparency (commonly described from shallow lakes; Scheffer et al., 1993; Meerhoff et al., 2007a) may be reduced in Mediterranean temporary ponds. Overall, our study confirms the existence of diel patterns of microhabitat selection in zooplankton of temporary wetlands, and provides new insights on how communities function in Mediterranean temporary ponds.
References
Alajärvi, E. & J. Horppila, 2004. Diel variations in the vertical distribution of crustacean zooplankton and food selection by planktivorous fish in a shallow turbid lake. International Review of Hydrobiology 89: 238–249.
Badosa, A., D. Boix, S. Brucet, R. López-Flores, S. Gascón & X. D. Quintana, 2007. Zooplankton taxonomic and size diversity in Mediterranean coastal lagoons (NE Iberian Peninsula): influence of hydrology, nutrient composition, food resource availability and predation. Estuarine Coastal and Shelf Science 71: 335–346.
Bagella, S. & M. C. Caria, 2012. Diversity and ecological characteristics of vascular flora in Mediterranean temporary pools. Comptes Rendus Biologies 335: 69–76.
Bazzanti, M., C. Coccia & M. G. Dowgiallo, 2010. Microdistribution of macroinvertebrates in a temporary pond of Central Italy: taxonomic and functional analyses. Limnologica 40: 291–299.
Beladjal, L., J. Mertens & H. J. Dumont, 1992. A simple basket trap for estimating relative abundances of some components of hyporheic faunas: application to the Cladocera. Stygologia 7: 193–195.
Blaustein, L. & H. J. Dumont, 1990. Typhloplanid flatworms (Mesostoma and related genera): mechanisms of predation and evidence that they structure aquatic invertebrate communities. Hydrobiologia 198: 61–77.
Blaustein, J., A. Sadeh & L. Blaustein, 2014. Influence of fire salamander larvae on among-pool distribution of mosquito egg rafts: oviposition habitat selection or egg raft predation? Hydrobiologia 723: 157–165.
Boeing, W. J., D. M. Leech, C. E. Williamson, S. Cooke & L. Torres, 2004. Damaging UV radiation and invertebrate predation: conflicting selective pressures for zooplankton vertical distribution in the water column of low DOC lakes. Oecologia 138: 603–612.
Boix, D., J. Sala & R. Moreno-Amich, 2001. The faunal composition of Espolla pond (NE Iberian peninsula): the neglected biodiversity of temporary waters. Wetlands 21: 577–592.
Boix, D., J. Sala, S. Gascón & S. Brucet, 2006. Predation in a temporary pond with special attention to the trophic role of Triops cancriformis (Crustacea: Branchiopoda: Notostraca). Hydrobiologia 571: 341–353.
Brendonck, L., E. Michels, L. De Meester & B. Riddoch, 2002. Temporary pools are not ‘enemy-free’. Hydrobiologia 486: 147–159.
Brucet, S., D. Boix, X. D. Quintana, E. Jensen, L. W. Nathansen, C. Trochine, M. Meerhoff, S. Gascón & E. Jeppesen, 2010. Factors influencing zooplankton size structure at contrasting temperatures in coastal shallow lakes: implications for effects of climate change. Limnology and Oceanography 55: 1697–1711.
Burks, R. L., E. Jeppesen & D. M. Lodge, 2001a. Littoral zone structures as Daphnia refugia against fish predators. Limnology and Oceanography 46: 230–237.
Burks, R. L., E. Jeppesen & D. M. Lodge, 2001b. Pelagic prey and benthic predators: impact of odonate predation on Daphnia. Journal of the North American Benthological Society 20: 615–628.
Burks, R. L., D. M. Lodge, E. Jeppesen & T. L. Lauridsen, 2002. Diel horizontal migration of zooplankton: costs and benefits of inhabiting the littoral. Freshwater Biology 47: 343–365.
Cerbin, S., D. J. Balayla & W. J. Van de Bund, 2003. Small-scale distribution and diel vertical migration of zooplankton in a shallow lake (Lake Naardermeer, the Netherlands). Hydrobiologia 491: 111–117.
Compte, J., S. Gascón, X. D. Quintana & D. Boix, 2012. The effects of small fish presence on a species-poor community dominated by omnivores: example of a size-based trophic cascade. Journal of Experimental Marine Biology and Ecology 418–419: 1–11.
Crespo, J. G., 2011. A review of chemosensation and related behavior in aquatic insects. Journal of Insect Science 11: 1–39.
Dawidowicz, P., J. Pijanowska & K. Ciechomski, 1990. Vertical migration of Chaoborus larvae is induced by the presence of fish. Limnology and Oceanography 35: 1631–1637.
Della Bella, V., M. Bazzanti & F. Chiarotti, 2005. Macroinvertebrate diversity and conservation status of Mediterranean ponds in Italy: water permanence and mesohabitat influence. Aquatic Conservation: Marine and Freshwater Ecosystems 15: 583–600.
De Silva, P. K., 1976. The factors affecting the feeding of Dendrocoelum lacteum (Muller) (Turbellaria, Tricladida) on Asellus aquaticus (L.) (Crustacean, Isopoda). Archiv Für Hydrobiologie 77: 347–374.
Dumont, H. J., I. Vandevelde & S. Dumont, 1975. Dry weight estimate of biomass in a selection of Cladocera, Copepoda and Rotifera from plankton, periphyton and benthos of continental waters. Oecologia 19: 75–97.
Font, J. & L. Vilar, 1998. Valoració florística de les basses de la Serra de l’Albera (Alt Empordà). Acta Botanica Barcinonensia (Homenatge a Oriol de Bolòs) 45: 299–307.
Gilbert, J. J. & S. E. Hampton, 2001. Diel vertical migrations of zooplankton in a shallow, fishless pond: a possible avoidance-response cascade induced by notonectids. Freshwater Biology 46: 611–621.
González-Sagrario, M. A. & E. Balseiro, 2003. Indirect enhancement of large zooplankton by consumption of predacious macroinvertebrates by littoral fish. Archiv für Hydrobiologie 158: 551–574.
González-Sagrario, M. D. & E. Balseiro, 2010. The role of macroinvertebrates and fish in regulating the provision by macrophytes of refugia for zooplankton in a warm temperate shallow lake. Freshwater Biology 55: 2153–2166.
Grillas, P., P. Gauthier, N. Yavercovski & P. Perennou, 2004. Mediterranean Temporary Pools. Station Biologique de la Tour du Vala, Arles.
Jeppesen, E., J. P. Jensen, M. Sondergaard, T. Lauridsen, L. J. Pedersen & L. Jensen, 1997. Top-down control in freshwater lakes: the role of nutrient state, submerged macrophytes and water depth. Hydrobiologia 342: 151–164.
José de Paggi, S. B. J., S. Muñoz, D. Frau, J. C. Paggi, P. Scarabotti, M. Devercelli & M. Meerhoff, 2012. Horizontal distribution of rotifers in a subtropical shallow lake (Parana floodplain, Argentina). Fundamental and Applied Limnology 180: 321–333.
Lampert, W., 1993. Ultimate causes of diel vertical migration of zooplankton: new evidence for the predator-avoidance hypothesis. Archiv für Hydrobiologie Supplement 39: 79–88.
Laufer, G., M. Arim, M. Loureiro, J. Manuel Piñeiro-Guerra, S. Clavijo-Baquet & C. Fagundez, 2009. Diet of four annual killifishes: an intra and interspecific comparison. Neotropical Ichthyology 7: 77–86.
Lauridsen, T. L. & I. Buenk, 1996. Diel changes in the horizontal distribution of zooplankton in the littoral zone of two shallow eutrophic lakes. Archiv für Hydrobiologie 137: 161–176.
Leech, D. M. & C. E. Williamson, 2000. Is tolerance to UV radiation in zooplankton related to body size, taxon, or lake transparency? Ecological Applications 10: 1530–1540.
Ma, Y., M. Mazumdar & S. G. Memtsoudis, 2012. Beyond Repeated measures Analysis of Variance: advanced statistical methods for the analysis of longitudinal data in anesthesia research. Regional Anesthesia and Pain Medicine 37: 99–105.
MacIsaac, H. J. & T. C. Hutchinson, 1985. The Influence of benthic tundra pond Vegetation and prey behavior on zooplankton predation by the flatworm Mesostoma lingua. Canadian Journal of Zoology-Revue Canadienne De Zoologie 63: 1617–1621.
Malley, D. F., 1989. Range of variation in estimates of dry weight for planktonic crustacea and rotifera from temperate north american lakes. Canadian Technical Report of Fisheries and Aquatic Sciences 1666: 1–49.
Martin, J. B., N. B. Withersp & M. H. Keenleys, 1974. Analysis of feeding behavior in newt Notophthalmus viridescens. Canadian Journal of Zoology-Revue Canadienne De Zoologie 52: 277–281.
Meerhoff, M., C. Fosalba, C. Bruzzone, N. Mazzeo, W. Noordoven & E. Jeppesen, 2006. An experimental study of habitat choice by Daphnia: plants signal danger more than refuge in subtropical lakes. Freshwater Biology 51: 1320–1330.
Meerhoff, M., J. M. Clemente, F. T. De Mello, C. Iglesias, A. R. Pedersen & E. Jeppesen, 2007a. Can warm climate-related structure of littoral predator assemblies weaken the clear water state in shallow lakes? Global Change Biology 13: 1888–1897.
Meerhoff, M., C. Iglesias, F. T. De Mello, J. M. Clemente, E. Jensen, T. L. Lauridsen & E. Jeppesen, 2007b. Effects of habitat complexity on community structure and predator avoidance behaviour of littoral zooplankton in temperate versus subtropical shallow lakes. Freshwater Biology 52: 1009–1021.
Monakov, A. B., 2003. Feeding of Freshwater Invertebrates. Kenobi Productions, Ghent.
Neill, W. E., 1990. Induced vertical migration in copepods as a defense against invertebrate predation. Nature 345: 524–526.
Nurminen, L. K. L. & J. A. Horppila, 2002. A diurnal study on the distribution of filter feeding zooplankton: effect of emergent macrophytes, pH and lake trophy. Aquatic Science 64: 198–206.
Nurminen, L. A., J. Horppila, L. Uusitalo & J. Niemistö, 2008. Spatial variability in the abundance of pelagic invertebrate predators in relation to depth and turbidity. Aquatic Ecology 42: 25–33.
Pickavance, J., 1971. Diet of immigrant planarian Dugesia tigrina (Girard). 1. Feeding in the Laboratory. Journal of Animal Ecology 40: 623–635.
Pielou, E. C., 1969. An Introduction to Mathematical Ecology. Wiley, New York.
Pinceel, T., B. Vanschoenwinkel, P. Deckers, A. Grégoir, T. Ver Eecke & L. Brendonck, 2015. Early and late developmental arrest as complementary embryonic bet-hedging strategies in African killifish. Biological Journal of the Linnean Society 114: 941–948.
Pinheiro, J. C., D. M. Bates, S. DebRoy & D. Sarkar, 2007. nlme: linear and nonlinear mixed effects models. R Foundation for Statistical Computing: R package version 3. 1–83.
Quintana, X. D., S. Brucet, D. Boix, R. López-Flores, S. Gascón, A. Badosa, J. Sala, R. Moreno-Amich & J. J. Egozcue, 2008. A nonparametric method for the measurement of size diversity with emphasis on data standardization. Limnology and Oceanography: Methods 6: 75–86.
Quintana, X. D., M. Arim, A. Badosa, J. M. Blanco, D. Boix, S. Brucet, J. Compte, J. J. Egozcue, E. de Eyto, U. Gaedke, S. Gascón, L. G. de Solá, K. Irvine, E. Jeppesen, T. L. Lauridsen, R. López-Flores, T. Mehner, S. Romo & M. Søndergaard, 2015. Predation and competition effects on the size diversity of aquatic communities. Aquatic Sciences 77: 45–57.
R Development Core Team, 2014. R: a language and environment for statistical computing. R Foundation for Statistical Computing: ISBN 3-900051-07-0.
Riemer, K. P., 2012. The dynamics of location: influence of predation by Chaoborus larvae on rotifer diel vertical migration Patterns. Lawrence University Honors Projects, Paper. 16.
Ribera, I., J. Isart & J. A. Régil, 1994. Coleópteros acuáticos de los Estanys de Campany (Girona): hydradephaga. Scientia Gerundensis 20: 17–34.
Rocha, O., T. Matsumuratundisi, J. G. Tundisi & C. P. Fonseca, 1990. Predation on and by pelagic Turbellaria in some lakes in Brazil. Hydrobiologia 198: 91–101.
Romare, P., S. Berg, T. Lauridsen & E. Jeppesen, 2003. Spatial and temporal distribution of fish and zooplankton in a shallow lake. Freshwater Biology 48: 1353–1362.
Rouissi, M., D. Boix, S. D. Muller, S. Gascón, A. Ruhí, J. Sala, A. Bouattour, I. B. H. Jilani, Z. Ghrabi-Gammar, S. B. Saad-Limam & A. Daoud-Bouattour, 2014. Spatio-temporal variability of faunal and floral assemblages in Mediterranean temporary wetlands. Comptes Rendus Biologies 337: 695–708.
Ruttner-Kolisko, A., 1977. Suggestions for biomass calculation of plankton rotifers. Archiv für Hydrobiologie: Ergebnisse der Limnologie 8: 71–76.
Scheffer, M., S. H. Hosper, M. L. Meijer, B. Moss & E. Jeppesen, 1993. Alternative Equilibria in shallow lakes. Trends in Ecology & Evolution 8: 275–279.
Schneider, D. W. & T. M. Frost, 1996. Habitat duration and community structure in temporary ponds. Journal of the North American Benthological Society 15: 64–86.
Schriver, P., J. Bøgestrand, E. Jeppesen & M. Søndergaard, 1995. Impact of submerged macrophytes on fish-zooplankton–phytoplankton interactions: large-scale enclosure experiments in a Shallow Eutrophic Lake. Freshwater Biology 33: 255–270.
Schwartz, S. S. & P. D. N. Hebert, 1986. Prey preference and utilization by Mesostoma lingua (Turbellaria, Rhabdocoela) at a low arctic site. Hydrobiologia 135: 251–257.
Tachet, H., P. Richoux, M. Bournaud & P. Usseglio-Polatera, 2000. Invertébrés d’eau douce. Systématique, biologie, écologie. CNRS Editions, Paris.
Tavşanoğlu, Ü. N., A. I. Cakiroğlu, Ş. Erdoğan, M. Meerhoff, E. Jeppesen & M. Beklioglu, 2012. Sediments, not plants, offer the preferred refuge for Daphnia against fish predation in Mediterranean shallow lakes: an experimental demonstration. Freshwater Biology 57: 795–802.
Tremel, B., S. E. Frey, N. D. Yan, K. M. Somers & T. W. Pawson, 2000. Habitat specificity of littoral chydoridae (Crustacea, Branchiopoda, Anomopoda) in Plastic Lake, Ontario, Canada. Hydrobiologia 432: 195–205.
Timms, R. M. & B. Moss, 1984. Prevention of growth of potentially dense phytoplankton populations by zooplankton grazing, in the presence of zooplanktivorous fish, in a shallow wetland ecosystem. Limnology and Oceanography 29: 472–486.
Trochine, C., B. Modenutti & E. Balseiro, 2005. When prey mating increases predation risk: the relationship between the flatworm Mesostoma ehrenbergii and the copepod Boeckella gracilis. Archiv Fur Hydrobiologie 163: 555–569.
Trochine, C., B. Modenutti & E. Balseiro, 2006. Influence of spatial heterogeneity on predation by the flatworm Mesostoma ehrenbergii (Focke) on calanoid and cyclopoid copepods. Journal of Plankton Research 28: 267–274.
Trochine, C., B. E. Modenutti & E. G. Balseiro, 2009. Chemical signals and habitat selection by three zooplankters in Andean Patagonian ponds. Freshwater Biology 54: 480–494.
Wainwright, P. E., S. T. Leatherdale & J. A. Dubin, 2007. Advantages of mixed effects models over traditional ANOVA models in developmental studies: a worked example in a mouse model of fetal alcohol syndrome. Developmental Psychobiology 49: 664–674.
Wang, Z. & L. A. Goonewardene, 2004. The use of MIXED models in the analysis of animal experiments with repeated measures data. Canadian Journal of Animal Science 84: 1–11.
Wellborn, G. A., D. K. Skelly & E. E. Werner, 1996. Mechanisms creating community structure across a freshwater habitat gradient. Annual Review of Ecology and Systematics 27: 337–363.
Williamson, C. E., H. E. Zagarese, P. C. Schulze, B. R. Hargreaves & J. Seva, 1994. The impact of short-term exposure to UV-B radiation on zooplankton communities in north temperate lakes. Journal of Plankton Research 16: 205–218.
Williamson, C. E., J. M. Fischer, S. M. Bollens, E. P. Overholt & J. K. Breckenridge, 2011. Toward a more comprehensive theory of zooplankton diel vertical migration: integrating ultraviolet radiation and water transparency into the biotic paradigm. Limnology and Oceanography 56: 1603–1623.
Whiteside, M. C., 1974. Chydorid (Cladocera) ecology: seasonal patterns and abundance of populations in Elk Lake, Minnesota. Ecology 55: 538–550.
Zimmer, K. D., M. A. Hanson, M. G. Butler & W. G. Duffy, 2001. Size distribution of aquatic invertebrates in two prairie wetlands, with and without fish, with implications for community production. Freshwater Biology 46: 1373–1386.
Acknowledgments
This work was supported by the Spanish Ministerio de Ciencia e Innovación, Programa de Investigación Fundamental No Orientada (CGL2011-23907). We would also like to thank A. Casellas for her field and lab assistance during the study, and E. Chappuis for her help in identifying macrophytes at the study site. We would like to thank American Journal Experts and Israel Leinbach and Eric Moody for their language edits. Finally, we thank the anonymous reviewers who helped improving the manuscript.
Author information
Authors and Affiliations
Corresponding author
Additional information
Handling editor: Mariana Meerhoff
Rights and permissions
About this article
Cite this article
Compte, J., Montenegro, M., Ruhí, A. et al. Microhabitat selection and diel patterns of zooplankton in a Mediterranean temporary pond. Hydrobiologia 766, 201–213 (2016). https://doi.org/10.1007/s10750-015-2455-2
Received:
Revised:
Accepted:
Published:
Issue Date:
DOI: https://doi.org/10.1007/s10750-015-2455-2