Abstract
Abdominal aortic aneurysm (AAA) has been associated with the dysfunction of vascular smooth muscle cells (VSMCs) and extracellular matrix (ECM) remodelling. Runt-related transcription factor 3 (RUNX3) has been reported to be up-regulated in aneurysmal aorta samples compared with normal aorta. However, its function in VSMCs and the mechanism of function remains unknown. Therefore, our study aimed to investigate the role of RUNX3 in ECM remodelling and VSMC function, and further explore the underlying mechanism. Our results verified that RUNX3 was increased in aortic samples of AAA compared with healthy controls. Overexpression vectors of RUNX3 (ov-RUNX3) and siRNA of RUNX3 (si-RUNX3) were transfected into Human aortic smooth muscle cells (HAoSMCs). The results indicated that ov-RUNX3 promoted cell proliferation, migration, and MMP-2/3/9 secretion, and suppressed TIMP-1, collagen I/III, SM22, MYH11 and CNN1 expression in HAoSMCs. The silencing of RUNX3 has the opposite effect. Furthermore, we found that RUNX3 targets TGF-β1 and suppressed its transcription. The silencing of TGF-β1 increased cell proliferation, migration and MMP-2/3/9 expression, and inhibited TIMP-1, Collagen I/III, SM22, MYH11 and CNN1 expression. In addition, TGF-β1 reversed the effect of RUNX3 overexpression on HAoSMCs. Hence, our study indicated that RUNX3 promotes cell proliferation, migration, and ECM remodelling through suppressing TGF-β1.
Similar content being viewed by others
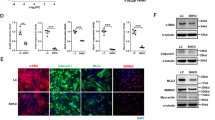
Avoid common mistakes on your manuscript.
Introduction
Abdominal aortic aneurysm (AAA) is a degenerative disease with irreversible and progressive dilation of the artery (Chen et al. 2020). Progressive aortic dilation leads to the eventual rupture of the aorta, accompanied by great threat to life (Golledge 2019). In adults older than 50 years, AAA affects approximately 5–7% of men, and 1.3% of women (Guirguis-Blake et al. 2019). It causes 150,000–200,000 deaths every year worldwide. The development of AAA is a complex process, characterised by the depletion and dysfunction of vascular smooth muscle cells (VSMCs), inflammation, and aortic wall extracellular matrix (ECM) remodelling (Klaus et al. 2017; Xu et al. 2019). In healthy aorta, ECM is constantly remodelled to maintain the integrity of the vessel wall, and provides a physical scaffold for the cells that make up the aorta (Kim et al. 2009; Wagenseil and Mecham 2009). In AAA, the ECM homeostasis is imbalanced, characterised by the abnormal deposition of collagen, increased ratio of collagen I/III, and disorganised collagen arrangement (Huang et al. 2016). Previous studies have reported that matrix metalloproteinases (MMPs) were up-regulated in the aortic wall of AAA patients, including MMP-1, MMP-2, MMP-3, MMP-9, and MMP-13 (Rabkin 2017). It is known that MMPs contribute to the degradation of ECM. However, the pathogenic mechanism of ECM remodelling in VSMCs during AAA progression has not been fully elucidated.
Runt-related transcription factors (RUNX) play crucial roles in multiple signalling pathways and cellular processes through multiple protein-interacting partners (Chuang et al. 2013). Three RUNX genes have been identified in mammals: RUNX1, RUNX2, and RUNX3 (Rahmanian et al. 2017). All of these Runt-related genes were found to be up-regulated in aneurysmal aorta samples, compared with normal aorta (Dubis et al. 2016). RUNX2 was reported to participate in the AAA formation by regulating the microcalcification of VSMCs (Li et al. 2020). Deficient RUNX2 mice exhibited a lower incidence of AAA after stimulation by angiotensin II (Li et al. 2020). RUNX3 exhibits an anti-tumour effect in most tumours (Li et al. 2019; Milner et al. 2017; Sun et al. 2018); nevertheless, it promotes cancer in ovarian cancer and human epithelial cancer cells (Date and Ito 2020; Lee et al. 2011). In addition, RUNX3 overexpression facilitated endothelial-mesenchymal transition and endothelial cell dysfunction (Liu et al. 2017). A previous study of whole genome expression profiling in human AAA showed that RUNX3 was presented as an upregulated gene for immune function in AAA and confirmed the increased expression of RUNX3 by Q-RT-PCR analysis in RNA specimen obtained from AAA tissues (Lenk et al. 2007). However, its role in AAA remains unclear.
In this study, the expression of RUNX3 in AAA tissues was measured, and the effect of the overexpression or inhibition of RUNX3 in human aortic smooth muscle cell (HAoSMC) proliferation, apoptosis, migration, and extracellular matrix remodelling was analysed. In addition, the underlying mechanism of RUNX3 function in HAoSMCs was further explored.
Materials and methods
Subjects
A total of 22 patients with AAA in Weihai Municipal Hospital from 2018 were included. Patient inclusion criteria included: (1) no history of malignancies; (2) no other types of severe disease, for instance, severe mental disorders, and vascular diseases; and (3) newly diagnosed cases which had received no treatment before sampling. The patients included 13 males and 9 females, and ages ranged from 42 to 67 years, with a mean age of 52.3 ± 4.6 years. The control group was consisted of the adjacent non–aneurysmal segment/pieces of aorta from the same patients, according to previous studies (He et al. 2019; Song et al. 2020). Aortic biopsy was performed and aortic samples were collected from each participant. This study was approved by the Ethics Committee of Weihai Municipal Hospital, and all participants signed informed consent.
Cell culture
HAoSMCs (catalog number: PCS-100-012) were provided by the American Type Culture Collection (ATCC, Manassas, VA), and were cultured in medium 231 with smooth muscle growth supplements (Cascade Biologics, Portland, OR) at 37 °C in a classic CO2 incubator. The cryopreserved cells were at the second passage of culture to guarantee the cell viability and experimental efficiency for the highest level, avoiding effects occurred on our research by adverse cell performance.
Plasmid construction and stable transfection
Overexpression vectors of pCMV6-RUNX3 (OV-RUNX3), RUNX3 siRNA oligo duplex (si-RUNX3) and TGF-β1 siRNA oligo duplex (si-TGF-β1) were purchased from ORIGENE (OriGene, USA). Cell transfection was performed using Lipofectamine 3000 (Invitrogen) following the manufacturer's instructions. Negative control scramble siRNA (si-NC) or empty overexpression vectors (ov-NC) were transfected into cells as a negative control. The sequences are as follows: si-RUNX3 forward, 5'-TTTGCGGAGTAGTTCTCGTCATACAATGACGAGAACTACTCCGCTTTTT-3'; si-RUNX3 reverse, 5'-CTAGAAAAAGCGGAGTAGTTC.
TCGTCATTGTATGACGAGAACTACTCCG-3'.
RNA preparation and quantitative real-time PCR
Total RNA from aortic media specimens and HAoSMCs were extracted using TRIzol reagent (Thermo Fisher Scientific, Inc., Rockford, IL). Complementary DNA (cDNA) was synthesised by reverse transcription using the SuperScript IV Reverse Transcriptase Kit (Thermo Fisher Scientific). Quantitative PCR analysis was performed using SYBR Green Real-Time PCR Master Mix (Thermo Fisher Scientific) on CFX96 Touch Real-Time PCR Detection System (Bio-Rad, Hercules, CA). The following primers were used in this study: RUNX3 forward 5'-AGGCAATGACGAGAACTACTCC-3', and reverse: 5'-CGAAGGTCGTTGAACCTGG-3'; TGF-β1 forward 5'-TACAGCACGGTATGCAAGCC-3', and reverse: 5'-GCAACCGATCTAGCTCACAGAG-3'; and GAPDH forward 5'-TGTGGGCATCAATGGATTTGG-3', and reverse: 5'-ACACCATGTATTCCGGGTCAAT-3'. The 2−ΔΔtC method was used to process all data, and GAPDH was adopted as an endogenous control.
Dual-luciferase reporter assay
Wild-type and mutant of TGF-β1 promoter cDNA fragments were amplified and inserted downstream of the pGL4.1 luciferase reporter plasmid. Luciferase reporter plasmid and the overexpression vectors of RUNX3 or empty overexpression vectors were co-transfected into HAoSMCs cells using Lipofectamine 3000 (Invitrogen) following the manufacturer's instructions. The luciferase activity was measured through the Dual Luciferase Reporter System Kit (Promega, Madison, WI, USA).
Cell proliferation assay
Cell counting kit-8 (CCK-8, Dojindo, Tokyo, Japan) was adopted in this study to analyse the cell proliferation. HAoSMCs were plated onto 96-well plates and cultured overnight. After being incubated with TGF-β1 for 24 h, CCK-8 reagent (10 μL) was added to each well and cultured for 2 h. The OD values at 450 nm were measured using a microplate reader.
Cell apoptosis assay
Cell apoptosis was measured through Roche’s Cell Death Detection ELISAPLUS Kit (Roche Diagnostics Corporation, Indianapolis, IN) and Caspase-3 activity assay kit (Cell Signalling Technology, Danvers, MA, USA) following the manufacturer's instructions.
Cell migration test
The migration of HAoSMCs was analysed through the transwell chamber migration assay. HAoSMC mixed serum-free culture media were planted into the upper chamber, while culture medium with 10% serum was added to the lower chamber. After incubated for 48 h at 37 °C, cells in the upper surface of chamber were removed by cotton swabs; the migrated cells in the lower chamber were fixed with paraformaldehyde (4%, w/v) for 20 min and then stained with crystal violet for 15 min. Images were obtained in an inverted microscope and the migrated cells were counted.
ELISA test
Culture medium of HAoSMCs was collected and the content of MMP-2, MMP-3, and MMP-9 was measured using the human MMP2 ELISA Kit (ab267813), human MMP3 ELISA Kit (ab269371), and human MMP9 ELISA Kit (ab246539) according to the manufacturer's instructions (Abcam, Cambridge, UK).
Western blot analysis
Western blot was performed as previously described (Hu et al. 2019). Antibodies used in this study include the anti-RUNX3 antibody (ab53066, 1:1000, Abcam), anti-TGF-β1 antibody (ab40278, 1:800, Abcam), anti-caspase 3 antibody, active (cleaved) form (AB3623, 1:200, Sigma-Aldrich), anti-Bax antibody (B3428, 1:500, Sigma-Aldrich), anti-Bcl-2 antibody (B3170, 1:1000, Sigma-Aldrich), anti-TIMP-1 antibody (ab211926, 1:1000, Abcam), anti-Collagen I antibody (ab34710, 1:500, Abcam), anti-Collagen III antibody (ab6310, 1:500, Abcam), goat anti-rat IgG H&L (HRP) (ab7097, 1:2000, Abcam) and goat anti-mouse IgG H&L (HRP) (ab97040, 1:2000, Abcam). Anti-GAPDH antibody (ab9485, 1:1000, Abcam) was used as the loading control.
Statistical analysis
Data are presented as the mean of three independent biological replicates. Data were processed by GraphPad software; differences between 2 groups were explored using the unpaired t-test, and more than two groups were analysed by one-way ANOVA combined with the Tukey test. P < 0.05 was statistically significant.
Results
RUNX3 is up-regulated in AAA patients
The expression of RUNX3 in aortic media from AAA patients and healthy controls was detected through quantitative PCR analysis. The results show that RUNX3 was significantly increased in AAA patients (n = 22), compared with normal controls (n = 15, P < 0.001, Fig. 1A). Meanwhile, three aortic tissue samples were randomly selected from AAA patients and healthy controls; the protein expression of RUNX3 in these six samples was measured. As shown in Fig. 1 B and C, RUNX3 protein expression was markedly higher in AAA patients than in normal controls (P < 0.001).
Effect of RUNX3 overexpression and inhibition on cell proliferation, apoptosis and phenotype marker expression
To explore the role of RUNX3 in AAA progression, the function of RUNX3 in HAoSMCs was explored. Overexpression vectors of RUNX3 (ov-RUNX3) and siRNA of RUNX3 (si-RUNX3) were transfected into HAoSMCs to promote or suppress RUNX3 expression (Fig. 2A and B. As shown in Fig. 2C, RUNX3 overexpression promoted the cell proliferation of HAoSMCs, while RUNX3 inhibition suppressed HAoSMC proliferation. Also, the silencing of RUNX3 promoted HAoSMC apoptosis (Fig. 2D and E). The protein expression of cleaved-caspase 3 and Bax were promoted by RUNX3 inhibition, while Bcl-2 was suppressed by RUNX3 inhibition in HAoSMCs (Fig. 2F). In addition, overexpression of RUNX3 markedly inhibited SM22, MYH11 and CNN1 expression (Fig. 2G). Conversely, the silence of RUNX3 elicited the opposite effect of SM22, MYH11 and CNN1 expression (Fig. 2G).
Effect of RUNX3 overexpression and inhibition on cell proliferation and apoptosis. A and B Transfection efficiency of overexpression vectors of RUNX3 (ov- RUNX3) and siRNA of RUNX3 (si-RUNX3) in HAoSMC cells. C Cell proliferation was measured by CCK-8 assay kit. D and E Cell apoptosis was analyzed through Roche’s Cell Death Detection ELISAPLUS Kit and Caspase 3 activity kit. F The expression of cleaved-Caspase 3, BCL-2 and Bax were detected through Western blotting. G. The expression of SM22, MYH11 and CNN1 were detected using qPCR. **P < 0.01, compared with ov-NC group. ##P < 0.01, compared with si-NC group
Effect of RUNX3 overexpression and inhibition on cell migration and extracellular matrix remodelling
Next, we found that RUNX3 overexpression promoted the migration of HAoSMCs, while the silencing of RUNX3 has an opposite effect (Fig. 3A). The levels of MMP-2, MMP-3, and MMP-9 were markedly increased in the culture medium of HAoSMCs after the overexpression of RUNX3, and decreased after the silencing of RUNX3 (Fig. 3B–D). Meanwhile, we found that the protein expression of TIMP-1 (tissue inhibitor of metalloproteinases 1), Collagen I and Collagen III was decreased in the ov-RUNX3 transfected group, and increased in the si-RUNX3 transfected group (Fig. 3E and F).
Effect of RUNX3 overexpression and inhibition on cell migration and extracellular matrix remodeling. A Cell migration was measured through the transwell assay. B–D Relative expression of MMP-2, MMP-3 and MMP-9 in the culture medium of HAoSMC cells. E and F Protein expression of TIMP-1, Collagen I and Collagen III in HAoSMC cells were detected through Western blotting. *P < 0.05, **P < 0.01, compared with ov-NC group. #P < 0.05, ##P < 0.01, compared with si-NC group
RUNX3 targets TGF-β1 and suppressed its transcription
A previous study reported that RUNX3 binds with the promoter of TGF-β1 at base pairs 1154-1296 in SW480 cells (Zhang et al. 2020). The luciferase reporter assay results verified that RUNX3 overexpression inhibited the promoter luciferase activity of TGF-β1 in HAoSMCs (Fig. 4A). Furthermore, we found that transfection with ov-RUNX3 into HAoSMCs significantly suppressed the mRNA and protein expression of TGF-β1; meanwhile, TGF-β1 was up-regulated in HAoSMCs after the silencing of RUNX3 (Fig. 4B and C). Also, the effect of TGF-β1 on the expression of RUNX3 in HAoSMCs was explored. The results indicated that TGF-β1 at a dose of 0–40 ng/mL made no significant changes on the expression of RUNX3 in HAoSMCs (Fig. 4D–F).
RUNX3 targets TGF-β1 and suppressed its transcription. A Relative luciferase activity of TGFB1 promoter was measured after transfection with ov-RUNX3 or ov-NC. **P < 0.01. B and C Expression of TGF-β1 mRNA and protein in HAoSMC cells were measured after transfection with ov-RUNX3 or si-RUNX3. **P < 0.01, compared with ov-NC group. ##P < 0.01, compared with si-NC group. D–F Effect of TGF-β1 on RUNX3 expression
Effect of TGF-β1 silence on cell proliferation, migration, extracellular matrix remodelling and phenotype marker expression
As shown in Fig. 5A and B, TGF-β1 was inhibited by transfection with si-TGF-β1. TGF-β1 inhibition promoted cell proliferation and the migration of HAoSMCs (Fig. 5C and D). At the same time, the levels of MMP-2, MMP-3, and MMP-9 were notably increased in HAoSMCs after the silencing of TGF-β1 (Fig. 5E). The expression of TIMP-1, Collagen I and Collagen III was decreased in the si-TGF-β1 transfected group, compared with the si-NC transfected group (Fig. 5F and G).
Effect of si-TGF-β1 on HAoSMC cells function. A and B Transfection efficiency of TGF-β1 siRNA (si-TGF-β1) in HAoSMC cells. C–G Cell proliferation (C), migration (D), contents of MMP-2, MMP-3 and MMP-9 (E), protein expression of TIMP-1, Collagen I and Collagen III (F and G) in HAoSMC cells were detected after transfection with si-TGF-β1. *P < 0.05, **P < 0.01, compared with si-NC group
Enhanced TGF-β1 reversed the effect of RUNX3 overexpression on HAoSMCs
To explore the role of TGF-β1 in RUNX3-mediated cell proliferation, migration, extracellular matrix remodeling and phenotype marker expression in HAoSMCs. TGF-β1 (20 ng/mL) was added into the culture medium of HAoSMCs and incubated for 24 h. The results suggested that TGF-β1 suppressed cell proliferation and migration in HAoSMCs (Fig. 6A–B). Meanwhile, TGF-β1 reversed the promoting effect ov-RUNX3 on cell proliferation and migration in HAoSMCs (Fig. 6A–B). Also, TGF-β1 treatment suppressed the expression of MMP-2, MMP-3, and MMP-9 in HAoSMCs, and also partially reversed the promoting effect of ov-RUNX3 on MMP-2, MMP-3, and MMP-9 production (Fig. 6C–E). In addition, TGF-β1 stimulation promoted TIMP-1, Collagen I and Collagen III expression, and suppressed the expression of TIMP-1, Collagen I and Collagen III induced by RUNX3 overexpression in HAoSMCs (Fig. 6F and G).
Enhanced TGF-β1 reversed the effect of RUNX3 overexpression on HAoSMC cells. Cell proliferation (A), migration (C), content of MMP-2, MMP-3 and MMP-9 (C–E), protein expression of TIMP-1, Collagen I and Collagen III (F and G) in HAoSMC cells were detected. *P < 0.05, **P < 0.01, compared with the control group, #P < 0.05, compared with ov-RUNX3 transfected group
Discussion
In this study, we found that RUNX3 was up-regulated in AAA patients and its up-regulation promoted HAoSMC proliferation and migration. Meanwhile, the silencing of RUNX3 suppressed the proliferation and migration of HAoSMCs, and induced cell apoptosis. SMCs are the main intrinsic cells in the aortic wall; their dysfunction contributes to the gradual dilatation and eventual rupture of the aorta (Wang et al. 2018). They perform their functions through proliferation, migration, and the secretion of various cytokines (Xue et al. 2019), while VSMC hyperplasia was regarded as critical for AAA (Li et al. 2018). Hence, it is predicted that RUNX3 is involved in the initiation and progression of AAA.
Another major function of SMCs is the maintenance of ECM homeostasis. The results in this study indicated that RUNX3 promoted the secretion of MMP-2, MMP-3, and MMP-9, and inhibited the expression of TIMP-1, and collagens I/III. Previous studies suggested that RUNX3 up-regulated MMP-2 and MMP-9 expression in colorectal tumour cells (Kim et al. 2016; Xue et al. 2020). Accumulating evidence indicates that MMPs are overexpressed in AAA and promote its progression. MMP-3 deficiency attenuates ECM damage and suppresses the susceptibility of mice to develop AAA (Hadi et al. 2018). Meanwhile, SMC-derived MMP-3 promotes AAA progression (Hadi et al. 2018). Also, MPPs can destruct the orderly collagen and elastin network of the aorta. MMP-2/9 can degrade solubilised monomers of type I and III collagen (Van Doren 2015). TIMP-1 as an inhibitor of MMP-1, -3, and -9 could suppress the activity of MMPs (Rabkin 2014). Collagens I/III are the building blocks for the aortic wall, and play an important role in keeping the strength and stiffness of the aortic wall (Deguchi et al. 2009). Hence, it is indicated that RUNX3 may be involved in ECM remodelling through promoting MMPs and inhibiting TIMP-1 and collagen I/III accumulation. In addition, inflammation is also a pivotal factor in the development and progression of AAA (Xu et al. 2019). Runx3 is involved in many important immune processes. In Runx3 knockout mice, Runx3 deletion resulted in multiple immunological defects (Lotem et al. 2017). Besides, it has been shown that RUNX3 is associated with immune-related diseases of the gastrointestinal tract and several other organs (Guo et al. 2010; Weersma et al. 2008). These data demonstrate the significance of RUNX3 in inflammation. In this work, we focused only on the role of RUNX3 in VSMC proliferation, migration, and ECM remodelling. In the next study, we will devote to explore the role of RUNX3 in AAA inflammatory process.
TGF-β1 was found to be elevated in the AAA tissues of humans and experimental animals (Doyle et al. 2015; Spin et al. 2011). In addition, TGF-β1 regulates vascular remodelling by promoting collagen synthesis and suppressing collagen degradation (Jones et al. 2009). Meanwhile, TGF-β1 participates in regulation of the growth, migration, and differentiation of VSMCs (Pardali et al. 2010). Activated TGF-β1 could enhance the transcription of collagen, and MMPs. Previous studies suggested that TGF-β signalling in VSMCs plays a protective role in abdominal aortic health (Angelov et al. 2017). In our study, we found that the overexpression of RUNX3 suppressed the transcription of TGF-β1, while RUNX3 inhibition promoted TGF-β1 expression. This result is consistent with a previous report that RUNX3 binds to the promoter region of TGF-β1 in colorectal cancer cells and suppresses the transcription of TGF-β1 (Zhang et al. 2020). Besides, knockdown of RUNX3 in hypoxia-induced human cardiac microvascular endothelial cells (HCMECs) increased the level of TGF-β1 isoform TGF-β2 (Liu et al. 2016). Given the important role of TGF-β1 in the AAA process and the few studies on RUNX3 and TGF-β1 isoforms, herein, we mainly investigated whether RUNX3 regulates TGF-β1 expression to influence AAA onset and progression. In addition, our study found that TGF-β1 reversed the promoting effect of RUNX3 on VSMC proliferation and migration, and MMP production, and promoted collagen protein expression. These results suggest that RUNX3 aggravates VSMC proliferation, migration, and ECM remodelling, at least in part by suppressing TGF-β1 transcription.
In conclusion, we discovered that RUNX3 was increased in AAA patients, and its overexpression induced VSMC proliferation, migration, and ECM remodelling, while the silencing of RUNX3 has the opposite effect. The underlying mechanism might involve binding to the promoter of TGF-β1 and suppressing its transcription. These findings suggest that RUNX3 may be a promising therapeutic target for VSMC hyperplasia and vascular ECM remodelling in AAA patients.
Data availability
The analyzed data sets generated during the study are available from the corresponding author on reasonable request.
Abbreviations
- AAA:
-
Abdominal aortic aneurysm
- ECM:
-
Extracellular matrix
- VSMCs:
-
Vascular smooth muscle cells
- MMPs:
-
Matrix metalloproteinases
- TIMP-1:
-
Tissue inhibitor of metalloproteinases 1
- RUNX:
-
Runt related transcription factors
- HAoSMCs:
-
Human aortic smooth muscle cells
- OV-RUNX3:
-
Overexpression vectors of RUNX3
- si-RUNX3:
-
RUNX3 siRNA oligo duplex
References
Angelov SN, Hu JH, Wei H, Airhart N, Shi M, Dichek DA (2017) TGF-β (transforming growth factor-β) signaling protects the thoracic and abdominal aorta from angiotensin II-induced pathology by distinct mechanisms. Arterioscler Thromb Vasc Biol 37:2102–2113
Chen C, Wang Y, Cao Y, Wang Q, Anwaier G, Zhang Q, Qi R (2020) Mechanisms underlying the inhibitory effects of probucol on elastase-induced abdominal aortic aneurysm in mice. Br J Pharmacol 177:204–216
Chuang LS, Ito K, Ito Y (2013) RUNX family: regulation and diversification of roles through interacting proteins. Int J Cancer 132:1260–1271
Date Y, Ito K (2020) Oncogenic RUNX3: a link between p53 deficiency and MYC dysregulation. Mol Cells 43:176–181
Deguchi JO, Huang H, Libby P, Aikawa E, Whittaker P, Sylvan J, Lee RT, Aikawa M (2009) Genetically engineered resistance for MMP collagenases promotes abdominal aortic aneurysm formation in mice infused with angiotensin II. Lab Invest 89:315–326
Doyle AJ, Redmond EM, Gillespie DL, Knight PA, Cullen JP, Cahill PA, Morrow DJ (2015) Differential expression of Hedgehog/Notch and transforming growth factor-β in human abdominal aortic aneurysms. J Vasc Surg 62:464–470
Dubis J, Litwin M, Michalowska D, Zuk N, Szczepanska-Buda A, Grendziak R, Baczynska D, Barc P, Witkiewicz W (2016) Elevated expression of runt-related transcription factors in human abdominal aortic aneurysm. J Biol Regul Homeost Agents 30:497–504
Golledge J (2019) Abdominal aortic aneurysm: update on pathogenesis and medical treatments. Nat Rev Cardiol 16:225–242
Guirguis-Blake JM, Beil TL, Senger CA, Coppola EL (2019) Primary care screening for abdominal aortic aneurysm: updated evidence report and systematic review for the US preventive services task force. JAMA 322:2219–2238
Guo C, Yao F, Wu K, Yang L, Zhang X, Ding J (2010) Chromatin immunoprecipitation and association study revealed a possible role of Runt-related transcription factor 3 in the ulcerative colitis of Chinese population. Clin Immunol 135:483–489
Hadi T, Boytard L, Silvestro M, Alebrahim D, Jacob S, Feinstein J, Barone K, Spiro W, Hutchison S, Simon R, Rateri D, Pinet F, Fenyo D, Adelman M, Moore KJ, Eltzschig HK, Daugherty A, Ramkhelawon B (2018) Macrophage-derived netrin-1 promotes abdominal aortic aneurysm formation by activating MMP3 in vascular smooth muscle cells. Nat Commun 9:5022
He X, Wang S, Li M, Zhong L, Zheng H, Sun Y, Lai Y, Chen X, Wei G, Si X, Han Y, Huang S, Li X, Liao W, Liao Y, Bin J (2019) Long noncoding RNA GAS5 induces abdominal aortic aneurysm formation by promoting smooth muscle apoptosis. Theranostics 9:5558–5576
Hu W, Wang Z, Li Q, Wang J, Li L, Jiang G (2019) Upregulation of lincRNA-p21 in thoracic aortic aneurysms is involved in the regulation of proliferation and apoptosis of vascular smooth muscle cells by activating TGF-β1 signaling pathway. J Cell Biochem 120:4113–4120
Huang Y, Shen Z, Chen Q, Huang P, Zhang H, Du S, Geng B, Zhang C, Li K, Tang C, Du J, Jin H (2016) Endogenous sulfur dioxide alleviates collagen remodeling via inhibiting TGF-β/Smad pathway in vascular smooth muscle cells. Sci Rep 6:19503
Jones JA, Spinale FG, Ikonomidis JS (2009) Transforming growth factor-beta signaling in thoracic aortic aneurysm development: a paradox in pathogenesis. J Vasc Res 46:119–137
Kim HJ, Kim MY, Jin H, Kim HJ, Kang SS, Kim HJ, Lee JH, Chang KC, Hwang JY, Yabe-Nishimura C, Kim JH, Seo HG (2009) Peroxisome proliferator-activated receptor delta regulates extracellular matrix and apoptosis of vascular smooth muscle cells through the activation of transforming growth factor-{beta}1/Smad3. Circ Res 105:16–24
Kim BR, Kang MH, Kim JL, Na YJ, Park SH, Lee SI, Kang S, Joung SY, Lee SY, Lee DH, Min BW, Oh SC (2016) RUNX3 inhibits the metastasis and angiogenesis of colorectal cancer. Oncol Rep 36:2601–2608
Klaus V, Tanios-Schmies F, Reeps C, Trenner M, Matevossian E, Eckstein HH, Pelisek J (2017) Association of matrix metalloproteinase levels with collagen degradation in the context of abdominal aortic aneurysm. Eur J Vasc Endovasc Surg 53:549–558
Lee CW, Chuang LS, Kimura S, Lai SK, Ong CW, Yan B, Salto-Tellez M, Choolani M, Ito Y (2011) RUNX3 functions as an oncogene in ovarian cancer. Gynecol Oncol 122:410–417
Lenk GM, Tromp G, Weinsheimer S, Gatalica Z, Berguer R, Kuivaniemi H (2007) Whole genome expression profiling reveals a significant role for immune function in human abdominal aortic aneurysms. BMC Genomics 8:237
Li FF, Shang XK, Du XL, Chen S (2018) Rapamycin treatment attenuates angiotensin II -induced abdominal aortic aneurysm formation via VSMC phenotypic modulation and down-regulation of ERK1/2 activity. Curr Med Sci 38:93–100
Li X, Zhong M, Wang J, Wang L, Lin Z, Cao Z, Huang Z, Zhang F, Li Y, Liu M, Ma X (2019) miR-301a promotes lung tumorigenesis by suppressing Runx3. Mol Cancer 18:99
Li Z, Zhao Z, Cai Z, Sun Y, Li L, Yao F, Yang L, Zhou Y, Zhu H, Fu Y, Wang L, Fang W, Chen Y, Kong W (2020) Runx2 (runt-related transcription factor 2)-mediated microcalcification is a novel pathological characteristic and potential mediator of abdominal aortic aneurysm. Arterioscler Thromb Vasc Biol 40:1352–1369
Liu Y, Li B, Wang Y, Wang D, Zou J, Ke X, Hao Y (2016) Knockdown of RUNX3 inhibits hypoxia-induced endothelial-to-mesenchymal transition of human cardiac microvascular endothelial cells. Xi Bao Yu Fen Zi Mian Yi Xue Za Zhi 32:1627–1631
Liu Y, Zou J, Li B, Wang Y, Wang D, Hao Y, Ke X, Li X (2017) RUNX3 modulates hypoxia-induced endothelial-to-mesenchymal transition of human cardiac microvascular endothelial cells. Int J Mol Med 40:65–74
Lotem J, Levanon D, Negreanu V, Bauer O, Hantisteanu S, Dicken J, Groner Y (2017) Runx3 in immunity, inflammation and cancer. Adv Exp Med Biol 962:369–393
Milner JJ, Toma C, Yu B, Zhang K, Omilusik K, Phan AT, Wang D, Getzler AJ, Nguyen T, Crotty S, Wang W, Pipkin ME, Goldrath AW (2017) Runx3 programs CD8(+) T cell residency in non-lymphoid tissues and tumours. Nature 552:253–257
Pardali E, Goumans MJ, ten Dijke P (2010) Signaling by members of the TGF-beta family in vascular morphogenesis and disease. Trends Cell Biol 20:556–567
Rabkin SW (2014) Differential expression of MMP-2, MMP-9 and TIMP proteins in thoracic aortic aneurysm - comparison with and without bicuspid aortic valve: a meta-analysis. Vasa 43:433–442
Rabkin SW (2017) The role matrix metalloproteinases in the production of aortic aneurysm. Prog Mol Biol Transl Sci 147:239–265
Rahmanian N, Tarighi P, Gharghabi M, Torshabi M, Tarfiei GA, Mohammadi Farsani T, Ostad SN, Ghahremani MH (2017) Truncated forms of RUNX3 unlike full length protein alter cell proliferation in a TGF-β context dependent manner. Iran J Pharm Res 16:1194–1203
Song H, Xu T, Feng X, Lai Y, Yang Y, Zheng H, He X, Wei G, Liao W, Liao Y (2020) Itaconate prevents abdominal aortic aneurysm formation through inhibiting inflammation via activation of Nrf2. EBioMedicine 57:102832
Spin JM, Hsu M, Azuma J, Tedesco MM, Deng A, Dyer JS, Maegdefessel L, Dalman RL, Tsao PS (2011) Transcriptional profiling and network analysis of the murine angiotensin II-induced abdominal aortic aneurysm. Physiol Genomics 43:993–1003
Sun J, Li B, Jia Z, Zhang A, Wang G, Chen Z, Shang Z, Zhang C, Cui J, Yang W (2018) RUNX3 inhibits glioma survival and invasion via suppression of the β-catenin/TCF-4 signaling pathway. J Neurooncol 140:15–26
Van Doren SR (2015) Matrix metalloproteinase interactions with collagen and elastin. Matrix Biol 44–46:224–231
Wagenseil JE, Mecham RP (2009) Vascular extracellular matrix and arterial mechanics. Physiol Rev 89:957–989
Wang YD, Liu ZJ, Ren J, Xiang MX (2018) Pharmacological therapy of abdominal aortic aneurysm: an update. Curr Vasc Pharmacol 16:114–124
Weersma RK, Zhou L, Nolte IM, van der Steege G, van Dullemen HM, Oosterom E, Bok L, Peppelenbosch MP, Faber KN, Kleibeuker JH, Dijkstra G (2008) Runt-related transcription factor 3 is associated with ulcerative colitis and shows epistasis with solute carrier family 22, members 4 and 5. Inflamm Bowel Dis 14:1615–1622
Xu X, Zhang F, Lu Y, Yu S, Sun W, Sun S, Cheng J, Ma J, Zhang M, Zhang C, Zhang Y, Zhang K (2019) Silencing of NONO inhibits abdominal aortic aneurysm in apolipoprotein E-knockout mice via collagen deposition and inflammatory inhibition. J Cell Mol Med 23:7449–7461
Xue J, Wu X, Qu M, Guo F, Han L, Sun G, Yuan Z, Fan S, Li T (2020) RUNX3 inhibits the invasion and metastasis of human colon cancer HT-29 cells by upregulating MMP-2/9. Evid Based Complement Alternat Med 2020:5978131
Xue M, Li G, Li D, Wang Z, Mi L, Da J, Jin X (2019) Up-regulated MCPIP1 in abdominal aortic aneurysm is associated with vascular smooth muscle cell apoptosis and MMPs production. Biosci Rep. https://doi.org/10.1042/BSR20191252
Zhang Y, Wang S, Lai Q, Fang Y, Wu C, Liu Y, Li Q, Wang X, Gu C, Chen J, Cai J, Li A, Liu S (2020) Cancer-associated fibroblasts-derived exosomal miR-17-5p promotes colorectal cancer aggressive phenotype by initiating a RUNX3/MYC/TGF-β1 positive feedback loop. Cancer Lett 491:22–35
Funding
None.
Author information
Authors and Affiliations
Contributions
ZZ and HZ: conception, design and analysis of data, performed the data analyses, and wrote the manuscript. XZ: contributed to the conception of the study. XW: contributed significantly to analysis and manuscript preparation. All authors contributed to interpretation of date and review of the manuscript, and approved this manuscript for submission.
Corresponding author
Ethics declarations
Conflict of interest
The authors declare that they have no conflict of interest.
Additional information
Publisher's Note
Springer Nature remains neutral with regard to jurisdictional claims in published maps and institutional affiliations.
Rights and permissions
About this article
Cite this article
Zhou, Z., Zhou, H., Zou, X. et al. RUNX3 is up-regulated in abdominal aortic aneurysm and regulates the function of vascular smooth muscle cells by regulating TGF-β1. J Mol Histol 53, 1–11 (2022). https://doi.org/10.1007/s10735-021-10035-9
Received:
Accepted:
Published:
Issue Date:
DOI: https://doi.org/10.1007/s10735-021-10035-9