Abstract
Elucidating the growth responses of roots to water status will reveal physiological mechanisms underlying drought tolerance and water conservation in plants. Hydroponic experiments were conducted using two winter wheat cultivars, Wangshuibai (drought-sensitive) and Luohan 7 (drought-tolerant), and a water deficit was induced using a 20 % (m/v) aqueous solution of polyethylene glycol 6000 (−0.6 MPa). The lack of water significantly reduced the plant dry weight, leaf area, total root length (TRL) and surface area in seminal (SRs) and nodal roots (NRs), but the effects were less pronounced in Luohan 7 than in Wangshuibai. After re-watering, leaf area, TRL and surface area of Luohan 7 increased significantly, as compared to the controls, due to rapid compensatory growth of SRs, while those of Wangshuibai were still significantly reduced. Under water-deficit conditions, the concentrations of indole-3-acetic acid (IAA) and cytokinin (CTK) and their ratio (IAA/CTK) in SRs and NRs of both cultivars were significantly lower than those of controls, but increased after re-watering. However, Luohan 7 showed significantly increases in IAA/CTK of SRs as compared to the control. Net photosynthetic rate was much lower during water deficit in both cultivars, but it was enhanced significantly after re-watering, especially for Luohan 7. Moreover, sucrose content was significantly increased in leaves while reduced in roots under water-deficit conditions. After re-watering, sucrose content in leaves of both cultivars and in roots of Wangshuibai was severely reduced, while the values in roots of Luohan 7 were significantly increased as compared to the control. These results indicate that the drought-tolerant cultivar has a greater ability to maintain plant growth under water deficit and greater compensatory growth in SRs associated with higher IAA/CTK and photosynthetic products supply after re-watering.
Similar content being viewed by others
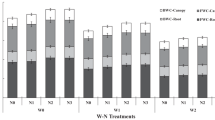
Explore related subjects
Discover the latest articles, news and stories from top researchers in related subjects.Avoid common mistakes on your manuscript.
Introduction
Drought is a major constraint to global crop production (Shane et al. 2010; Chaves and Oliveira 2004). As a worldwide planting cereal crop, winter wheat production requires more water than other crops due to its longer growth period. In China, drought occurs more frequently during winter and spring seasons, which severely affects the formation of photosynthetic organs and tiller number during seedling period, hence reduces the accumulation of materials and energy and grain yield. So breeding more tolerant cultivars to drought stress is becoming an urgent target for high yield and water-saving cultivation.
As the main organ for capturing water and nutrients from soil, the plant root system is strongly affected by the water status of the soil (Osmont et al. 2007; Hermans et al. 2006; Malamy 2005). However, it has strong morphologic plasticity (e.g., changes in length and in the number of lateral roots and root hairs) to adapt to heterogeneous and changing environments (Valladares et al. 2007; Gruber et al. 2013). For example, under drought conditions, plants develop deep and extensive root systems as an adaptive response (Serraj et al. 2004), including thick roots with an increased length and density (Siopongco et al. 2006). Deeper roots are important for plant growth under drought conditions (Sanguineti et al. 2007; Araus et al. 2008): deep roots may absorb water from deep soil layers to help plants survive under drought stress (Gowda et al. 2011). However, decreased root growth in response to drought has been reported (Vandoorne et al. 2012). These responses differ according to the crop species and the timing and intensity of drought (Kano et al. 2011). Therefore, it is important to explore the mechanisms regulating the morphology of the root system in relation to water status to increase crop yields under drought conditions (Uga et al. 2013).
The morphology of the root system is associated with endogenous hormones. Indole-3-acetic acid (IAA) and cytokinin (CTK) play important roles in regulating and modulating the formation of lateral roots in response to environmental changes (Swarup et al. 2008; Kuderová et al. 2008; Casimiro et al. 2003; Malamy 2005). CTK is mainly produced by the root cap meristem and negatively regulates meristem size and root length (Dello Ioio et al. 2007). IAA is mainly synthesized in the shoot apical meristem and is transported from shoots to roots through the phloem (Woodward and Bartel 2005; Ljung et al. 2001) to promote root branching and the formation of adventitious roots (Casimiro et al. 2001; Woodward and Bartel 2005; Quint et al. 2009). Therefore, CTK and IAA have antagonistic roles during root formation (Aloni et al. 2006). It has been reported that water stress reduced the synthesis of CTK in roots and decreased its upward transportation (Li and Li 2007). Under drought conditions, the endogenous contents of IAA usually decreased (Yang et al. 2001). Nevertheless, there are reports that drought did not change IAA levels in leaf and root tissues (Mahouachi et al. 2007) and that moderate drought stress increased IAA transport in the root tip (Xu et al. 2013). Thus, those changes in distribution and concentrations of IAA and CTK in plants would regulate root morphology under different water status. Unfortunately, the regulatory mechanism remains unclear.
The morphology of roots may also be regulated by the concentration of sucrose. In wheat, increased lateral roots are associated with increased concentrations of glucose and sucrose in the roots (Bingham et al. 1998). In tobacco, there is a relationship between the growth rate and sugar content of roots (Schiefelbein and Benfey 1991). Previous studies have also demonstrated that an increase in sucrose metabolism under osmotic stress may induce the root system to produce more branches (MacGregor et al. 2008). Karthikeyan et al. (2007) found that the exogenous supply of sucrose increased the density of lateral roots in Arabidopsis, and this effect was enhanced by the addition of IAA. Nevertheless, our understanding of these phenomena is mostly based on studies of Arabidopsis thaliana. Therefore, it is important to explore the regulatory mechanisms of food crops to improve their drought tolerance. However, our understanding of these regulatory mechanisms remains limited.
In crops, root morphology is closely associated with the growth and development of shoots because of the role of roots in water and nutrient uptake (Samejima et al. 2004; Zhang et al. 2009). Under drought conditions, rice roots produce signals to regulate leaf stomatal conductance, transpiration, and shoot growth (Siopongco et al. 2008). Two root types are distinguished in cereals: seminal roots (SRs, also called primary roots) and nodal roots (NRs, also called secondary or crown roots) (Manske and Vlek 2002). Both SRs and NRs in wheat maintain active function throughout all plant stages (Sanguineti et al. 2007). It is reasonable to speculate that SRs may be equally or even more vital than NRs under conditions of water stress for capturing water and nutrients from the soil and sustaining plant growth because they can penetrate more deeply into the soil (Manske and Vlek 2002). However, most studies of plant responses to water stress have been performed using the whole root system (Serraj et al. 2013); few studies have considered SRs under such conditions (Sahnoune et al. 2004). In addition, many studies have focused on the negative effects of a water deficit on root growth (Rostamza et al. 2013). Information on the effects of water stress and re-watering conditions on the morphologies of SRs and NRs of wheat, and the relationship with shoot growth, is unavailable.
Therefore, the objectives of the present study were to: (i) examine the responses of wheat in terms of SR and NR morphology to water deficit and re-watering conditions and elucidate the regulatory mechanism, and (ii) to clarify the relationship between root morphology and shoot growth. Our results may be used to uncover physiological mechanisms of drought tolerance and to select or breed wheat cultivars that are adapted to water-deficit conditions.
Materials and methods
Plant materials and experimental design
Two wheat cultivars with different responses to drought (Luohan 7, a drought-tolerant cultivar; and Wangshuibai, a drought-sensitive cultivar) were used for hydroponic experiments (Ma et al. 2012). Uniform seeds were surface-sterilized with 20 % hydrogen peroxide for 10 min, rinsed with distilled water, and then germinated in a Petri dish with two pieces of filter paper. The seeds were transferred to vermiculite until the bud length was approximately 1 cm. Uniform wheat seedlings at the one-leaf stage were transplanted to plastic containers (32 cm long, 21 cm wide, and 11 cm tall) filled with modified Hoagland nutrient solution containing 1 mM Ca(NO3)2·4H2O, 2 mM KNO3, 0.5 mM CaCl2, 0.5 mM (NH4)2SO4, 1 mM KH2PO4, 1 mM MgSO4·7H2O, 0.5 mM NaCl, 5 μM Fe-EDTA, 0.55 μM MnSO4·H2O, 0.0385 μM ZnSO4·7H2O, 2.35 μM H3BO3, 0.0165 μM CuSO4·5H2O, and 0.0065 μM H2MoO4. The seedlings were cultivated in a greenhouse under 16/8 h of light/dark conditions at 18/8.5 °C (day/night). The pH of the nutrient solution was controlled at 5.50 ± 0.05 by adding 0.1 mM HCl or 0.1 mM NaOH every day. The nutrient solutions were changed every 3 days.
The seedlings were divided into two batches at the four-leaf stage. One batch was treated with 20 % (m/v) polyethylene glycol 6000 (−0.6 MPa) (Michel and Kaufmann 1973) to induce a water deficit for 5 days. Another batch served as controls (CK). After inducing water deficit, the seedlings were re-watered until there was no difference in leaf relative water content (LRWC) between the control and deficit-exposed plants of cultivar Luohan 7 (Fig. 1). The experiment had a completely randomized block design with three replicates.
Changes in relative water content in leaves of Luohan 7 (L) and Wangshuibai (W) after water-deficit treatment (WD) and re-watering (RW). Uparrow represents the start of re-watering; L-CK refers to control Luohan 7 plants; L-WD + RW refers to Luohan 7 plants exposed to water-deficit conditions + re-watering; W-CK refers to control Wangshuibai plants; and W-WD + RW refers to Wangshuibai plants exposed to water-deficit conditions + re-watering. The data are given as mean ± standard error (n = 3)
Leaf samples were collected to determine the LRWC on 1, 3, and 5 days after water deficit, and on 1 and 3 days after re-watering treatment, respectively. Gas exchange was examined on the same day. After these experiments, another batch of samples was taken and divided into two groups. One group was further divided into leaves, SRs, and NRs, and all samples were frozen in liquid nitrogen and stored at −80 °C. The other group was used for dry weight measurements. These plants were divided into shoots and roots and dried at 105 °C for 20 min and then at 85 °C to obtain a constant weight.
Measurement of total root length (TRL) and root surface area (RSA)
Uniform roots were chosen after the control and experimental treatments. SRs and NRs were separated and rinsed with distilled water, and then scanned using a scanner (Epson 1680, Suwa, Japan) and finally analyzed using WinRHIZO Pro Vision 5.0 (Elmira, ON, Canada) to obtain the TRL and RSA.
The relative growth rate (RGR) of the TRL was calculated using the equation
where L1′ and L2′ are the TRLs of the water deficit-treated plants on day 5 of stress treatment and day 3 of re-watering, respectively, and L1 and L2 are the TRLs of control plants measured at the same time as L1′ and L2′, respectively.
The RGR of the RSA was calculated using the equation
where A1′ and A2′ are the RSAs of the water deficit-treated plants on day 5 of stress treatment and day 3 of re-watering, respectively, and A1 and A2 are the RSAs of control plants measured at the same time as A1′ and A2′, respectively.
Determination of the LRWC and leaf area
The LRWC was determined as described previously (Meng et al. 2013). Leaf area was measured by using a LI-3000 area meter (Li-Cor Inc., Lincoln, NE, USA).
Gas exchange measurements
The net photosynthetic rate (Pn) and stomatal conductance (Gs) were measured for the top leaf between 09:00 and 11:00 a.m. using a LI-6400 portable photosynthesis system (Li-Cor Inc.). The leaf chamber CO2 content was managed at ca. 370 μmol mol−1 and the photosynthetic active radiation was set to 1000 mmol m−2 s−1.
Determination of the sucrose content
Dried samples were ground into a powder and analyzed for the sucrose content. Dried samples (0.1 g) were extracted three times with 80 % (v/v) ethanol at 80 °C to extract sugars, and then centrifuged at 3,000 × g. The supernatant was collected to measure the sucrose content using the resorcinol method (Zhang and Qu 2003).
Measurement of endogenous hormones
The methods used for the extraction and purification of IAA, zeatin (Z) + zeatin riboside (ZR), and isopentenyladenine (iP) + isopentenyladenine riboside (iPR) were essentially as described previously (Yang et al. 2001; Liu et al. 2011). Briefly, approximately 0.5 g of frozen tissue was ground in 5 ml of 80 % (v/v) methanol extraction solution containing 1 mM butylated hydroxytoluene as an antioxidant and put into a centrifuge tube, and then incubated in the dark at 4 °C for 4 h. After incubation, the tubes were centrifuged at 10,000 × g for 15 min at 4 °C. Chromosep C18 columns (C18 Sep-Park Cartridge; Waters Corp., Milford, MA, USA), prewashed with 5 ml of 80 % methanol, were used to filter the supernatants. The filtrates were dried using a freeze dryer (ALPHA 2–4/LSC; Christ Corp., Harz, Germany) and dissolved in 1 ml of phosphate-buffered saline (pH 7.5) containing 0.1 % (v/v) Tween 20 and 0.1 % (w/v) gelatin. The endogenous hormones were analyzed using an enzyme-linked immunosorbent assay (ELISA).
All reagents used in the ELISA, including the monoclonal antigens and antibodies against IAA, Z + ZR, and iP + iPR and immunoglobulin G-horseradish peroxidase were purchased from the Phytohormones Research Institute of China Agricultural University (Beijing, China). The concentrations of IAA, Z + ZR, and iP + iPR were determined by ELISAs as described previously (Yang et al. 2001). The CTK concentration was calculated based on the Z + ZR and iP + iPR concentrations.
Statistical analysis
The results represent the means of three replicates and were subjected to a two-way analysis of variance to identify significant differences between cultivar and water status using SPSS (ver. 13.0; SPSS, Chicago, IL, USA) software.
Results
Effects of water deficit and re-watering on plant dry weight and leaf area
Water-deficit treatment significantly reduced plant dry weight and leaf area in both cultivars. Compared to controls, the magnitude of the effect was lower in Luohan 7 than in Wangshuibai (Table 1). Though the plant dry weight of the two cultivars was markedly lower after re-watering treatment, the decreases in Luohan 7 were from 38 to 35 % after re-watering, while those in Wangshuibai were from 51 to 55 %, indicating that the plant growth rate was higher in Luohan 7. Moreover, no significant change in leaf area between the stressed and control plants after re-watering in Luohan 7, while significant decreases were observed in Wangshuibai. These data suggest that the drought-tolerant cultivar maintained a greater capacity for plant growth under water-deficit conditions and exhibited a fast compensatory ability during re-watering.
Effects of water deficit and re-watering on TRL and RSA
Under water-deficit conditions, the TRL and RSA of the SRs, NRs, and total roots (TRs) in both cultivars decreased significantly, but the decreases were lighter in Luohan 7 than those in Wangshuibai (Table 2). After re-watering, the TRL and RSA of the TRs showed no significant differences in Luohan 7, as compared with the controls, but they remained lower in Wangshuibai. However, the TRL and RSA of the SRs in Luohan 7 were significantly higher than those in controls after re-watering. On the contrary, the TRL and RSA were significantly lower in the NRs of Luohan 7 as well as in both the SRs and NRs of Wangshuibai after re-watering. Furthermore, for both stressed cultivars, the growth rates of the TRL and RSA in the SRs were much higher than those in controls after re-watering, which were different from those in the NRs (Fig. 2). Moreover, the growth rates of the TRL and RSA in the SRs of Luohan 7 were 3.1 and 2.7 times greater than those of Wangshuibai, respectively, indicating that the compensatory growth speed of the SRs in Luohan 7 was much faster than that in Wangshuibai after re-watering. Thus, the growth rates of the TRL and RSA in the TRs of Luohan 7 were much higher than those of the controls after re-watering, whereas those of Wangshuibai were lower than those of the controls. Due to the compensatory growth of the SRs, the TRL and RSA in the TRs of Luohan 7 exhibited no significant difference compared to controls after re-watering.
Effects of water deficit and re-watering on the concentrations of IAA and CTK
Water-deficit treatment decreased the concentrations of IAA and CTK in the SRs and NRs of the two cultivars (Fig. 3). Furthermore, the IAA concentrations were higher in the SRs of Luohan 7 after re-watering than in controls; however, they were still lower both in the SRs and NRs of Wangshuibai (Fig. 3a, b). In addition, the CTK concentrations were lower in the SRs of Luohan 7 after re-watering, while they were higher in the NRs of Luohan 7 and both in the SRs and NRs of Wangshuibai (Fig. 3c, d).
Effects of water-deficit treatment (WD) and re-watering (RW) on the IAA and CTK concentrations in the seminal roots (SRs) and nodal roots (NRs) of two wheat cultivars. DAW refers to days after water-deficit treatment; DAR refers to days after re-watering; IAA refers to Indole-3-acetic acid; and CTK refers to cytokinin. The concentrations of CTK are the total concentrations of zeatin (Z) + zeatin riboside (ZR) and isopentenyladenine (iP) + isopentenyladenine riboside (iPR). Different small letters indicate significant differences at the 0.05 level. The data are given as means + standard error (n = 3)
Under water-deficit conditions, the IAA/CTK decreased significantly in the SRs and NRs of both cultivars (Fig. 4). Furthermore, it decreased less in Luohan 7 than in Wangshuibai both in the SRs and NRs. After re-watering, the ratio increased significantly in both cultivars. In addition, the ratio in SRs of Luohan 7 was significantly higher than that in controls after re-watering, but the ratios were lower in the NRs of Luohan 7 and in the SRs and NRs of Wangshuibai.
Effects of water-deficit treatment (WD) and re-watering (RW) on the IAA/CTK in the seminal roots (SRs, a) and nodal roots (NRs, b) of two wheat cultivars. DAW refers to days after water-deficit treatment; DAR refers to days after re-watering; IAA refers to Indole-3-acetic acid; and CTK refers to cytokinin. The concentrations of CTK are the total concentrations of zeatin (Z) + zeatin riboside (ZR) and isopentenyladenine (iP) + isopentenyladenine riboside (iPR). Different small letters indicate significant differences at the 0.05 level. The data are given as means + standard error (n = 3)
Effects of water deficit and re-watering on Pn and Gs
For two cultivars, the Pn and Gs were decreased significantly under water-deficit conditions, but the decreases were less in Luohan 7 than that in Wangshuibai (Fig. 5). After re-watering, the Pn and Gs in the deficit-exposed plants were higher than those in controls of Luohan 7, but the values were lower than those in controls of Wangshuibai.
Changes in the net photosynthetic rate (Pn, a) and stomatal conductance (Gs, b) of Luohan 7 (L) and Wangshuibai (W) after water-deficit treatment (WD) and re-watering (RW). Uparrow represents the start of re-watering; L-CK refers to control Luohan 7 plants; L-WD + RW refers to Luohan 7 plants exposed to water-deficit conditions + re-watering; W-CK refers to control Wangshuibai plants; and W-WD + RW refers to Wangshuibai plants exposed to water-deficit conditions + re-watering. The data are given as mean ± standard error (n = 3)
Effects of water deficit and re-watering on sucrose content
The sucrose content increased significantly in leaves and decreased significantly in roots in both cultivars under water-deficit conditions (Fig. 6). However, the sucrose content increased less in the shoots and decreased less in the roots of Luohan 7 compared to Wangshuibai. After re-watering, the sucrose content decreased in the leaves of the stressed plants for both cultivars (Fig. 6a). In addition, the sucrose content increased significantly in the roots of the stressed plants for Luohan 7, while it decreased significantly in Wangshuibai (Fig. 6b).
Effects of water-deficit treatment (WD) and re-watering (RW) on the sucrose content in the leaves (a) and roots (b) of two wheat cultivars. DAW refers to days after water-deficit treatment; DAR refers to days after re-watering. Different small letters indicate significant differences at the 0.05 level. The data are given as means + standard error (n = 3)
Discussion
Many studies have demonstrated that roots can change their morphology to adapt to changes in the external environment (Valladares et al. 2007; Kano et al. 2011). Chu et al. (2014) proved that the root length and surface area of paddy rice (Oryza sativa L.) decreased under alternate wetting and drying irrigation. Here, the TRL and RSA decreased significantly under water-deficit conditions (Table 2). However, prior to this study, little information was available on the morphology of the SRs and NRs in wheat cultivars with different responses to drought under re-watering. Our results showed that the SRs underwent compensatory growth during re-watering. Furthermore, the compensatory growth of the SRs in Luohan 7 occurred more rapidly than in Wangshuibai during re-watering, resulting in a longer TRL and larger RSA in the SRs of Luohan 7, as compared with the controls, after re-watering (Fig. 2; Table 2). There was no pronounced difference in the TRL and RSA of the whole plant between the stressed plants and controls for Luohan 7 after re-watering due to the extra growth of the SRs (Table 2). These results imply that during re-watering after water-deficit treatment, the extra growth of the SRs promoted overall root growth in the drought-tolerant cultivar.
Blilou et al. (2005) observed that the allocation of IAA, controlled by five PIN genes, regulates cell division and elongation in the primary root. In the present study, the IAA concentrations were higher in the SRs of Luohan 7 alone after re-watering than in the controls (Fig. 3a, b), indicating that the higher growth rate of the SRs in Luohan 7 after re-watering was partly due to the increased root IAA concentration. Furthermore, the effects of IAA on root morphology are associated with CTK (Aloni et al. 2006; Blilou et al. 2005; Dello Ioio et al. 2007). CTK is believed to play an important role in promoting shoot growth but inhibiting root growth (Dello Ioio et al. 2007; Rahayu et al. 2005; Howell et al. 2003). It has been shown that the balance between IAA and CTK regulates the formation of roots (Blilou et al. 2005; Dello Ioio et al. 2007; Garay-Arroyo et al. 2012). Here, we detected a positive relationship between TRL and IAA/CTK in the roots under water-deficit conditions and re-watering. Our results indicate that the growth rate of the TRL was significantly correlated with the root IAA/CTK, and that water status regulated the TRL (especially for SRs) by influencing the concentrations of IAA and CTK and the balance between them. Therefore, the drought-tolerant cultivar exhibited a compensatory growth of the SRs in response to the higher root IAA/CTK during re-watering.
It is thought that cultivars possessing a root system with good soil penetration ability can capture more moisture and, therefore, maintain a high LRWC under water-deficit conditions (Luo 2010; Yue et al. 2006). Moreover, under drought conditions, water shortages in leaves would decrease Pn due to stomatal closure (Medrano et al. 2002). Here, we observed that the LRWC of Luohan 7 reduced to a lesser degree under water-deficit conditions and recovered more quickly during re-watering, and these changes were closely associated with changes in TRL and RSA in SRs especially after re-watering (Fig. 1; Table 2). During re-watering, the higher growth rates of the TRL and RSA in SRs of Luohan 7 contributed to a higher LRWC, leading to a higher Pn in the deficit-treated plants, as compared with the controls (Figs. 1, 2, 5a). Therefore, Pn was closely associated with SR growth of winter wheat seedlings during re-watering. In the present study, we also observed that leaf expansion in the drought-tolerant cultivar was faster during re-watering due to promoted growth of SRs, which would contribute to the improved Pn (Table 1). These results were confirmed using data obtained from the Wangshuibai cultivar. Thus, the extra growth of the SRs in the drought-tolerant cultivar contributed to the increases in photosynthesis and shoot growth.
There is an interdependent relationship between roots and shoots. Active roots can improve shoot characteristics by supplying ample water and nutrients; in turn, the improvement in shoot functions ensures a sufficient supply of carbohydrates to the roots to promote growth (Yang et al. 2004; Zhang et al. 2009). An important carbohydrate, sucrose, accumulates in leaves through photosynthesis, and its metabolism results in energy production, required for plant growth (MacGregor et al. 2008). It has been reported that the formation of roots may also be regulated by the sucrose content in plants. In many plants, a lack of nutrition can change the root structure due to the accumulation of sucrose or starch in leaves, altering the distribution of carbon in the root system (Hermans et al. 2006). Studies of A. thaliana have shown that under osmotic deficit an increase in sucrose metabolism induces the root system to produce more branches (MacGregor et al. 2008). In this study, we observed that water-deficit treatment decreased the metabolism of sucrose, resulting in the accumulation of sucrose in leaves and inhibiting its transportation to roots (Fig. 6). In addition, a higher IAA concentration in SRs and a more active sucrose metabolism was observed in Luohan 7 after re-watering, increasing the growth rate of SRs (Figs. 3a, 6). One possible reason is that sucrose metabolism in leaves stimulates the transport of IAA to roots; thus, increased IAA in roots could promote sucrose transport from leaves to roots, stimulating root growth further, which is in accordance with the finding by Bhalerao et al. (2002).
Water-deficit treatment limits the growth and decreases the biomass of plants (Shane et al. 2010; Chaves and Oliveira 2004). In agreement with this, our results showed that the plant dry weight of both cultivars decreased significantly (P < 0.05) under water deficit (Table 1). However, the decreases were less severe in Luohan 7; this can be explained by the less decreases in the activity of the roots and Pn in the leaves under water-deficit conditions. It has been reported that deeper roots, especially deeper SRs, are important and required for plant growth under drought conditions (Sanguineti et al. 2007; Araus et al. 2008). However, little is known about those effects after water-deficit treatment. Our results indicate that the faster growth rate of SRs in Luohan 7 promoted the recovery of the whole root system during re-watering and, thus, accelerated the absorption of moisture to improve the Pn, all of which contributed to the higher growth rate of Luohan 7.
In conclusion, water-deficit treatment reduced plant growth, but the drought-tolerant cultivar possessed a greater ability to maintain plant growth. Extra growth of the SRs, regulated by the higher root IAA/CTK after re-watering, promoted the recovery of such root traits as TRL and RSA. Moreover, the higher water status promoted by the improved TRL and RSA during re-watering promoted photosynthesis, which in turn contributed to higher shoot and root growth rates in the drought-tolerant cultivar by accelerating the synthesis and transport of sucrose.
References
Aloni R, Aloni E, Langhans M, Ullrich C (2006) Role of cytokinin and auxin in shaping root architecture: regulating vascular differentiation, lateral root initiation, root apical dominance and root gravitropism. Ann Bot 97(5):883–893
Araus JL, Slafer GA, Royo C, Serret MD (2008) Breeding for yield potential and stress adaptation in cereals. Crit Rev Plant Sci 27(6):377–412
Bhalerao RP, Eklöf J, Ljung K, Marchant A, Bennett M, Sandberg G (2002) Shoot-derived auxin is essential for early lateral root emergence in Arabidopsis seedlings. Plant J 29(3):325–332
Bingham I, Blackwood J, Stevenson E (1998) Relationship between tissue sugar content, phloem import and lateral root initiation in wheat. Physiol Plant 103(1):107–113
Blilou I, Xu J, Wildwater M, Willemsen V, Paponov I, Friml J, Heidstra R, Aida M, Palme K, Scheres B (2005) The PIN auxin efflux facilitator network controls growth and patterning in Arabidopsis roots. Nature 433(7021):39–44
Casimiro I, Marchant A, Bhalerao RP, Beeckman T, Dhooge S, Swarup R, Graham N, Inzé D, Sandberg G, Casero PJ (2001) Auxin transport promotes Arabidopsis lateral root initiation. Plant Cell 13(4):843–852
Casimiro I, Beeckman T, Graham N, Bhalerao R, Zhang H, Casero P, Sandberg G, Bennett MJ (2003) Dissecting Arabidopsis lateral root development. Trends Plant Sci 8(4):165–171
Chaves M, Oliveira M (2004) Mechanisms underlying plant resilience to water deficits: prospects for water-saving agriculture. J Exp Bot 55(407):2365–2384
Chu G, Chen T, Wang Z, Yang J, Zhang J (2014) Morphological and physiological traits of roots and their relationships with water productivity in water-saving and drought-resistant rice. F Crop Res 162:108–119
Dello Ioio R, Linhares FS, Scacchi E, Casamitjana-Martinez E, Heidstra R, Costantino P, Sabatini S (2007) Cytokinins determine Arabidopsis root-meristem size by controlling cell differentiation. Curr Biol 17(8):678–682
Garay-Arroyo A, De La Paz SánchezM, García-Ponce B, Azpeitia E, Álvarez-Buylla ER (2012) Hormone symphony during root growth and development. Dev Dyn 241(12):1867–1885
Gowda VR, Henry A, Yamauchi A, Shashidhar H, Serraj R (2011) Root biology and genetic improvement for drought avoidance in rice. F Crop Res 122(1):1–13
Gruber BD, Giehl RF, Friedel S, von Wirén N (2013) Plasticity of the Arabidopsis root system under nutrient deficiencies. Plant Physiol 163(1):161–179
Hermans C, Hammond JP, White PJ, Verbruggen N (2006) How do plants respond to nutrient shortage by biomass allocation? Trends Plant Sci 11(12):610–617
Howell SH, Lall S, Che P (2003) Cytokinins and shoot development. Trends Plant Sci 8(9):453–459
Kano M, Inukai Y, Kitano H, Yamauchi A (2011) Root plasticity as the key root trait for adaptation to various intensities of drought stress in rice. Plant Soil 342(1–2):117–128
Karthikeyan AS, Varadarajan DK, Jain A, Held MA, Carpita NC, Raghothama KG (2007) Phosphate starvation responses are mediated by sugar signaling in Arabidopsis. Planta 225:907–918
Kuderová A, Urbánková I, Válková M, Malbeck J, Brzobohatý B, Némethová D, Hejátko J (2008) Effects of conditional IPT-dependent cytokinin overproduction on root architecture of Arabidopsis seedlings. Plant Cell Physiol 49(4):570–582
Li T, Li S (2007) Leaf responses of micropropagated apple plants to water stress: changes in endogenous hormones and their influence on carbohydrate metabolism. Agric Sci China 6(1):58–67
Liu Y, Wang Q, Ding Y, Li G, Xu J, Wang S (2011) Effects of external ABA, GA3 and NAA on the tiller bud outgrowth of rice is related to changes in endogenous hormones. Plant Growth Regul 65(2):247–254
Ljung K, Bhalerao RP, Sandberg G (2001) Sites and homeostatic control of auxin biosynthesis in Arabidopsis during vegetative growth. Plant J 28(4):465–474
Luo L (2010) Breeding for water-saving and drought-resistance rice (WDR) in China. J Exp Bot 61:3509–3517
Ma F, Li D, Cai J, Jiang D, Cao W, Dai T (2012) Responses of wheat seedlings root growth and leaf photosynthesis to drought stress. J Appl Ecol 23(3):724–730 (in Chinese with English abstract)
MacGregor DR, Deak KI, Ingram PA, Malamy JE (2008) Root system architecture in Arabidopsis grown in culture is regulated by sucrose uptake in the aerial tissues. Plant Cell 20(10):2643–2660
Mahouachi J, Arbona V, Gómez-Cadenas A (2007) Hormonal changes in papaya seedlings subjected to progressive water stress and re-watering. Plant Growth Regul 53:43–51
Malamy J (2005) Intrinsic and environmental response pathways that regulate root system architecture. Plant Cell Environ 28(1):67–77
Manske GGB, Vlek PLG (2002) Root architecture-wheat as a model. In: Waisel Y, Eshel A (eds) Plant roots: the hidden half. Marcel Dekker, New York, pp 249–259
Medrano H, Escalona J, Bota J, Gulias J, Flexas J (2002) Regulation of photosynthesis of C3 plants in response to progressive drought: stomatal conductance as a reference parameter. Ann Bot 89(7):895–905
Meng G, Li G, He L, Chai Y, Kong J, Lei Y (2013) Combined effects of CO2 enrichment and drought stress on growth and energetic properties in the seedlings of a potential bioenergy crop Jatropha curcas. J Plant Growth Regul 32(3):542–550
Michel BE, Kaufmann MR (1973) The osmotic potential of polyethylene glycol 6000. Plant Physiol 51(5):914–916
Osmont KS, Sibout R, Hardtke CS (2007) Hidden branches: developments in root system architecture. Annu Rev Plant Biol 58:93–113
Quint M, Barkawi LS, Fan K-T, Cohen JD, Gray WM (2009) Arabidopsis IAR4 modulates auxin response by regulating auxin homeostasis. Plant Physiol 150(2):748–758
Rahayu YS, Walch-Liu P, Neumann G, Römheld V, von Wirén N, Bangerth F (2005) Root-derived cytokinins as long-distance signals for NO3 −-induced stimulation of leaf growth. J Exp Bot 56(414):1143–1152
Rostamza M, Richards R, Watt M (2013) Response of millet and sorghum to a varying water supply around the primary and nodal roots. Ann Bot 112(2):439–446
Sahnoune M, Adda A, Soualem S, Harch MK, Merah O (2004) Early water-deficit effects on seminal roots morphology in barley. CR Biol 327(4):389–398
Samejima H, Kondo M, Ito O, Nozoe T, Shinano T, Osaki M (2004) Root-shoot interaction as a limiting factor of biomass productivity in new tropical rice lines. Soil Sci Plant Nutr 50(4):545–554
Sanguineti M, Li S, Maccaferri M, Corneti S, Rotondo F, Chiari T, Tuberosa R (2007) Genetic dissection of seminal root architecture in elite durum wheat germplasm. Ann Appl Biol 151(3):291–305
Schiefelbein JW, Benfey PN (1991) The development of plant roots: new approaches to underground problems. Plant cell 3(11):1147–1154
Serraj R, Krishnamurthy L, Kashiwagi J, Kumar J, Chandra S, Crouch J (2004) Variation in root traits of chickpea (Cicer arietinum L.) grown under terminal drought. F Crop Res 88(2):115–127
Serraj R, Gowda V, Henry A, Fujita D, Kobayashi N, Yamauchi A, Inukai Y, Suralta R, Kano-Nakata M (2013) Functional roles of the plasticity of root system development in biomass production and water uptake under rainfed lowland condition. F Crop Res 144:288–296
Shane MW, McCully ME, Canny MJ, Pate JS, Huang C, Ngo H, Lambers H (2010) Seasonal water relations of Lyginia barbata (Southern rush) in relation to root xylem development and summer dormancy of root apices. New Phytol 185(4):1025–1037
Siopongco JD, Yamauchi A, Salekdeh H, Bennett J, Wade LJ (2006) Growth and water use response of doubled-haploid rice lines to drought and rewatering during the vegetative stage. Plant Prod Sci 9(2):141–151
Siopongco JD, Sekiya K, Yamauchi A, Egdane J, Ismail AM, Wade LJ (2008) Stomatal responses in rainfed lowland rice to partial soil drying; evidence for root signals. Plant Prod Sci 11(1):28–41
Swarup K, Benková E, Swarup R, Casimiro I, Péret B, Yang Y, Parry G, Nielsen E, De Smet I, Vanneste S (2008) The auxin influx carrier LAX3 promotes lateral root emergence. Nat Cell Biol 10(8):946–954
Uga Y, Sugimoto K, Ogawa S, Rane J, Ishitani M, Hara N, Kitomi Y, Inukai Y, Ono K, Kanno N (2013) Control of root system architecture by DEEPER ROOTING 1 increases rice yield under drought conditions. Nat Genet 45(9):1097–1102
Valladares F, Gianoli E, Gómez JM (2007) Ecological limits to plant phenotypic plasticity. New Phytol 176(4):749–763
Vandoorne B, Mathieu A-S, Van den, Ende W, Vergauwen R, Périlleux C, Javaux M, Lutts S (2012) Water stress drastically reduces root growth and inulin yield in Cichorium intybus (var. sativum) independently of photosynthesis. J Exp Bot 63(12):4359–4373
Woodward AW, Bartel B (2005) Auxin: regulation, action, and interaction. Ann Bot 95(5):707–735
Xu W, Jia L, Shi W, Liang J, Zhou F, Li Q, Zhang J (2013) Abscisic acid accumulation modulates auxin transport in the root tip to enhance proton secretion for maintaining root growth under moderate water stress. New Phytol 197:139–150
Yang J, Zhang J, Wang Z, Zhu Q, Wang W (2001) Hormonal changes in the grains of rice subjected to water stress during grain filling. Plant Physiol 127(1):315–323
Yang C, Yang L, Yang Y, Ouyang Z (2004) Rice root growth and nutrient uptake as influenced by organic manure in continuously and alternately flooded paddy soils. Agric Water Manag 70(1):67–81
Yue B, Xue W, Xiong L, Yu X, Luo L, Cui K, Jin D, Xing Y, Zhang Q (2006) Genetic basis of drought resistance at reproductive stage in rice: separation of drought tolerance from drought avoidance. Genetics 172(2):1213–1228
Zhang Z, Qu W (2003) The experimental guide for plant physiology, 3rd edn. Higher Education Press, Beijing, pp 128–129 (in Chinese)
Zhang H, Xue Y, Wang Z, Yang J, Zhang J (2009) An alternate wetting and moderate soil drying regime improves root and shoot growth in rice. Crop Sci 49(6):2246–2260
Acknowledgments
We acknowledge generous financial support from the National Natural Science Foundation of China (Grant No. 31471443) and a Project Funded by the Priority Academic Program Development of Jiangsu Higher Education Institutions (PAPD).
Author information
Authors and Affiliations
Corresponding author
Rights and permissions
About this article
Cite this article
Han, H., Tian, Z., Fan, Y. et al. Water-deficit treatment followed by re-watering stimulates seminal root growth associated with hormone balance and photosynthesis in wheat (Triticum aestivum L.) seedlings. Plant Growth Regul 77, 201–210 (2015). https://doi.org/10.1007/s10725-015-0053-y
Received:
Accepted:
Published:
Issue Date:
DOI: https://doi.org/10.1007/s10725-015-0053-y