Abstract
In this study, an MoS2/graphene composite is fabricated from bulk MoS2 and graphite rod via a facile electrochemical exfoliation method. The as-prepared samples are characterized by X-ray diffraction, field emission scanning electron microscopy, Fourier transform infrared spectroscopy and ultraviolet-visible spectroscopy techniques to confirm the formation of the MoS2/graphene composite. The electrochemical behavior of the MoS2/graphene composite is evaluated through cyclic voltammetry, galvanostatic charge/discharge and electrochemical impedance spectroscopy. It exhibits high specific capacitance of 227 F g−1 as compared with the exfoliated graphene (85 F g−1) and exfoliated MoS2 (70 F g−1) at a current density of 0.1 A g−1. This can be attributed to the synergistic effect between graphene and MoS2. Moreover, it displays high electrochemical stability and low electrical resistance.

Graphical abstract
Similar content being viewed by others
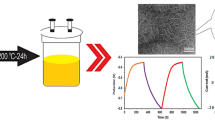
Avoid common mistakes on your manuscript.
Introduction
Two-dimensional (2D) materials, such as graphene, have garnered great interest from researchers for decades due to their outstanding mechanical, optical and physical properties [1,2,3]. Besides graphene, transition metal dichalcogenides, particularly molybdenum disulfide (MoS2), show promising potential in a wide range of applications including as catalysts and for energy storage and electronics [4, 5]. Bulk MoS2 crystals can undergo exfoliation to form MoS2 (a single, covalently bonded S–Mo–S tri-layer). This is the top-down synthesis approach for MoS2. To date, various exfoliation approaches including sonication, ion intercalation and electrochemical exfoliation have been used to produce high-quality MoS2 [6].
MoS2 is a semiconducting compound that consists of a 2D plane of a hexagonal crystal structure which is analogous to graphene [7, 8]. Each MoS2 layer consists of planes wherein the S–Mo–S atoms are combined by covalent bonds, and their neighboring layers are then attached to each other by weak van der Waals forces [9, 10]. From the other point of view, each Mo atom occupies a trigonal prism shape which is bound to six S atoms. The layered structure of MoS2 provides a good platform for ion adsorption and diffusion, rendering it a great choice for supercapacitor applications [11]. On the other hand, another layered structure material, graphene, also possesses excellent physical properties for good energy storage applications [12, 13]. However, the major concern for layered structure material is the tendency for restacking of sheets to occur, which has a detrimental effect on the energy storage capacity. In this context, the proposal for utilizing two different layered structure materials is worth investigating, as the different surface properties of two different materials could prevent surface interaction.
Herein, we report a novel electrode material, MoS2/graphene composite, for supercapacitor application. The MoS2/graphene composite is prepared via a facile electrochemical approach from the co-electrochemical exfoliation of MoS2 crystals and graphite rod. Interestingly, the incorporation of MoS2 with graphene leads to a remarkable enhancement in the specific capacitance of the electrode, with high cycling stability and low electrical resistance.
Experimental techniques
Sample preparation
MoS2, graphene and MoS2/graphene composite were synthesized using an electrochemical exfoliation method as previously reported, with some modifications [14, 15]. The bulk MoS2 crystal (99.99% purity, 429ML-AB, with size of 20 × 10 mm, SPI Supplies) was used as an anode, while a platinum wire was used as a cathode, with working distance of 2 cm in 1 M Na2SO4 solution. The preconditioning was conducted at DC voltage of 2 V for 5 min, followed by electrochemical exfoliation at 10 V for 5 min to obtain MoS2. A similar process was employed by replacing the MoS2 crystal with graphite rod (99.99% purity, 150 mm in length and diameter of 3 mm, Sigma-Aldrich) to exfoliate the graphite into graphene. Subsequently, a MoS2/graphene composite was obtained by connecting the MoS2 crystal and graphite rod as the anode using similar electrochemical conditions. Finally, exfoliated suspensions of MoS2, graphene and MoS2/graphene composite were collected separately using a polytetrafluoroethylene (PTFE) membrane (0.45 μm) and then washed with deionized water via vacuum filtration to remove the Na2SO4 solution. The collected samples were dried at 50 °C overnight in a vacuum oven.
Structural and morphological characterization
The crystal structure was analyzed using a Rigaku X-ray diffractometer (Miniflex II with Cu-Kα radiation at 40 kV, 30 mA, λ = 1.5406 Å) within the 2θ range of 5° to 80° at a scanning rate of 0.02 θ/s with a step time of 2 s. The functional groups were examined using a PerkinElmer (Spectrum 100) infrared spectrophotometer over the range of 400–4000 cm−1. UV-Vis measurements were performed using a Thermo Scientific (Genesys 10S) UV spectrophotometer at room temperature. The surface morphological analysis was conducted using a JEOL (JSM-7800F) field emission scanning electron microscope (FESEM) coupled with energy-dispersive X-ray spectroscopy (EDX).
Electrochemical characterization
Working electrodes were prepared by pressing a slurry of MoS2 or graphene or MoS2/graphene composite (90 wt%), carbon black (5 wt%) and polyvinylidene fluoride (PVDF, 5 wt%) onto a piece of nickel foam, and then drying at 70 °C for 24 h. The final mass loading of the active materials per electrode was 3–4 mg. The electrochemical performance of the electrode was investigated using a three-electrode system (3-ES), which consists of the active material as the working electrode, Ag/AgCl (CH Instruments) as a reference electrode, and Pt wire (CH Instruments) as the counter electrode. The electrochemical data were collected using an electrochemical workstation (AUTOLAB PGSTAT30, Netherlands) equipped with a frequency response analyzer. Cyclic voltammetry (CV) tests were performed in the potential range of −0.8 to 0.2 V at different scan rates. Galvanostatic charge-discharge (GCD) tests were performed at different current densities. Electrochemical impedance spectroscopy (EIS) data were collected from 50 kHz to 0.01 Hz at open-circuit potential (OCP) with a voltage signal of 10 mV amplitude. All measurements were carried out in 1.0 M Na2SO4 electrolyte at room temperature.
Results and discussion
Structural and morphological characterization
Figure 1 shows the XRD patterns of graphene, MoS2 and MoS2/graphene composite. A sharp peak can be seen at 26.38° and another small peak at 43.24°, which are related to the (002) and (100) planes of the graphene structure, respectively. The (002) plane of graphite can be detected in graphene due to the multiple layers of graphene that are formed [16]. Electrochemical exfoliation of the layered structure involves driving ionic species (H2O and SO42−) at high potential to intercalate into the interlayer distance, locally forming gas bubbles (SO2 and O2) that force the adjacent sheets to separate [17]. Furthermore, the absence of a graphene oxide peak at 2θ ~14° also signifies a negligible amount of oxidation in the samples [17]. On the other hand, all marked peaks related to the MoS2 have been indexed to the hexagonal phase of MoS2 (JCPDS no. 37-1492). The MoS2 pattern shows a sharp and visible peak at 2θ = 14°, which can be assigned to the (002) plane with d-spacing of 6.3 Å. This indicates the layered structure of MoS2 along the c axis [18]. It can be seen that the MoS2/graphene composite retains a layered crystallinity and the positions of diffraction peaks of MoS2. However, it is worth noting that the intensity of the diffraction peaks of MoS2 decrease with the incorporation of graphene, particularly the (002) peak. This indicates that the incorporation of graphene further restrains the stacking of MoS2 layers [19]. Furthermore, the presence of the graphene peak at 26.38° corresponding to the (002) plane is evidence that the graphene is incorporated into the composite.
Fourier transform infrared spectroscopy (FTIR) is employed to elucidate the functional groups of graphene, MoS2 and the MoS2/graphene composite [20]. As shown in Fig. 2, the peak at 620 cm−1 can be attributed to the Mo–S stretching vibration [21]. The multiple peaks at 700–1150 cm−1 can be assigned to the sulfate groups, while the sharp peaks at 3440 and 1610 cm−1 can be ascribed to the O–H stretching and water bending, respectively [22, 23]. This confirms the presence of sulfate and water molecules as intercalants during the electrochemical exfoliation process [24]. For the graphene spectrum, the intense peak located at 1610 cm−1 can be attributed to the aromatic C=C (stretching), and the very weak peak located at 1124 cm−1 corresponds to the C–O stretching [25]. In contrast, the absence of a C=O peak around 1715 cm−1 indicates the insignificant amount of oxidation of graphene. This further supports the XRD pattern of graphene, where the peak for graphene oxide (GO) is missing in the pattern. Interestingly, the intercalant-related peaks decrease in intensity for MoS2/graphene composite, which indicates the intercalants are removed from the interlayer structure due to the difference in surface chemistry between graphene and MoS2.
Figure 3a shows the UV-Vis spectra for MoS2, graphene and MoS2/graphene composite. Graphene exhibits a π-π* absorption band at 270 nm, which is in agreement with previous studies [26]. The results show that with the incorporation of graphene into MoS2, the absorption band at 270 nm of the MoS2/graphene composite increases. This phenomenon could be attributed to the black-body properties of graphene, which has been reported in the literature [27]. Figure 3b–c shows the relation between (αhυ)2 and hυ. The absorption coefficient (α) was calculated as α = 2.303 × 10−3Aρ/lC, where A is the measured absorbance, ρ is the density, l is the optical path length and C is the concentration [8, 28]. Extrapolation of the linear portion to zero absorption coefficient gives the value of the optical energy gap, which is found to be 1.98 and 1.75 eV for MoS2 and the MoS2/graphene composite, respectively, suggesting direct electronic transitions which are in good agreement with the MoS2 band structure. In addition, the bandgap value is closer to the reported values for monolayer MoS2 (1.9 eV) than bulk MoS2 (1.2 eV) [29], indicating the successful exfoliation of bulk MoS2. Moreover, incorporation of MoS2 with graphene leads to a decrease in the bandgap as a result of enhanced electrical conductivity of the hybrid material [30].
To reveal the morphology of all samples, FESEM analysis was employed. As illustrated in Fig. 4a and b, the FESEM images of MoS2 and graphene show nanosheet formation. The close-up view of MoS2/graphene composite in Fig. 4c shows the incorporation of MoS2 nanosheets onto the graphene nanosheets. It is clear that the MoS2 and graphene nanosheets are entwined to form an interconnected conductive network with a relatively smooth surface, which ensures optimum accessibility of electrolyte ions. Moreover, the elemental analysis for MoS2/graphene composite shows an atomic percent of 63.3, 14.5, 0.53 and 1.38% for C, O, Mo and S, respectively (Fig. S1). As we observed from FTIR findings, the oxygen content from EDX results could be related to the intercalated water molecule and sulfate ions. Overall, all of the results from XRD, FTIR, UV-Vis and FESEM confirm the successful electrochemical exfoliation to form MoS2/graphene composite.
Electrochemical characterization
Cyclic voltammetry is performed to determine the electrochemical performance of samples using a three-electrode system under a potential window from −0.8 to 0.2 V at different scan rates. Based on the XRD results, the d-spacing of the interlayer MoS2 structure is 6.3 Å. This is beneficial for rapid intercalation of hydrated Na+ ions (hydrated ionic radius of the Na(H2O)6+ is 2.43 Å) into MoS2 layers [31]. As shown in Fig. 5a, the charge storage occurs mainly by the insertion/extraction of Na+ ions into the interlayer of MoS2, which can be seen from the oxidation peak at around 0.55 V. Similar pseudocapacitance of ion insertion/extraction was observed for MoS2 in previous reports [32]. On the other hand, CV curves for graphene show an almost rectangular shape without any obvious redox peaks, which indicates the electrical double capacitance as the dominant charge storage mechanism (Fig. 5b). The slight deviation from the ideal rectangular shape may be due to the internal resistance or surface impurities. Figure 5c shows the CV curves of the MoS2/graphene composite at different scan rates. The CV curves feature strong electrical double-layer capacitance with a near-rectangular shape, as well as pseudocapacitance with reversible redox peaks. It is evident that the electrical double-layer capacitance is contributed by graphene, while the pseudocapacitance is associated with the surface redox of MoS2. Such synergistic effects between MoS2 (faradaic contribution) and graphene (non-faradaic contribution) can be represented by the following equations [32, 33]:
Furthermore, the CV curves retain their shape even at high scan rates, indicating the great high-rate performance. Noteworthily, the area of the CV curve for MoS2/graphene composite is larger than that of MoS2 and graphene at a scan rate of 25 mV s−1 (Fig. 5d), indicating that MoS2/graphene composite displays improved capacitive behavior and high charge storage. Detailed charge storage calculation is studied under galvanostatic charge-discharge.
Trasatti’s analysis method is utilized to provide insight with respect to the charge storage mechanism in MoS2/graphene composite. Based on Equations (S1–S3) [34], the capacitive charge storage (QC) can be obtained as an intercept by extrapolating the plot of voltammetric charge (QV) vs. υ−1/2, and the total amount of stored charges (QT) is obtained as intercept (υ approaching 0) through extrapolation of the plot 1/QV vs. υ1/2. As a result, the QC value of MoS2/graphene composite is measured to be 104.6 C g−1, and it is higher than of the graphene and MoS2, which are 68.8 and 6.5 C g−1, respectively (Fig. 6a). The high QC value of MoS2/graphene composite can be related to the improved electrical double-layer capacitance, probably due to the better ion accessibility within MoS2/graphene structure. On the other hand, the QT value for MoS2/graphene composite is 208.3 C g−1, which is higher than that of graphene and MoS2 (Table S1). It can be clearly seen that the charge storage in the MoS2/graphene composite originates equally from the capacitive and diffusion contributions (50.2% and 49.8%, respectively), which confirms the synergistic effect between graphene and MoS2.
The electrochemical performance of MoS2, graphene and MoS2/graphene composite is further confirmed by GCD study at different current densities, as represented in Fig. 7a–c, respectively. The charging curves are symmetrical and linear to their discharge counterpart, which indicates the excellent reversibility of the samples. The discharge time significantly increases in MoS2/graphene composite, indicating the increase in charge storage. Figure 7d compares the GCD curves of MoS2, graphene and MoS2/graphene electrodes at the current density of 1 A g−1. It is worth noting that the MoS2/graphene electrode has longer discharging time, indicating the higher specific capacitance of MoS2/graphene than that of MoS2 and graphene. The specific capacitance (Csp) in F g−1 of the as-prepared electrodes was calculated as: \( {C}_{sp}=I/m\left(\frac{dV}{dt}\right) \), where I is the discharge current, dV/dt is the slope of the discharge curve and m is the mass of active materials on the working electrode [11, 35, 36]. Based on Fig. 7e, MoS2/graphene composite exhibits the highest specific capacitance of 227 F g−1 followed by low specific capacitance values of graphene (85 F g−1) and MoS2 (70 F g−1) at a current density of 0.1 A g−1. Despite the decrease in the specific capacitance with increasing current density, the MoS2/graphene composite electrode still retains its higher capacitance as compared with other electrode materials at 5 A g−1, indicating an excellent rate performance of MoS2/graphene. A detailed comparison of specific capacitance reported with MoS2 and graphene-based nanocomposites is listed in Table 1.
The cycling stability performance of MoS2/graphene composite was determined by repeating the GCD test between −0.8 and 0.2 V at a current density of 1 A g−1 for 2000 cycles. The MoS2/graphene electrode loss is only 11% from the initial specific capacitance, with high coulombic efficiency (93%). The high stability of the MoS2/graphene composite is compared with related materials in Table 1. As shown in Fig. 8, the capacitance drop during the initial 250 cycles might be a result of the volume change due to electrolyte ion insertion. This phenomenon is widely seen in 2D materials [48].
To obtain further understanding of the electrochemical behavior of MoS2/graphene electrode, EIS results were collected in a frequency range from 50 kHz to 0.1 Hz at OCP. The Nyquist plots (Fig. 9a) show two distinct parts, with a semicircle in the high-frequency region and a linear portion in the low-frequency region. The circuit fitting parameters are tabulated in Table 2. The equivalent circuit consists of a solution resistance (RS), charge transfer resistance (RCT), capacitor (C), constant phase element (CPE) and Warburg element (W). It shows that the MoS2/graphene composite electrode possesses small values of RS (0.76 Ω.cm2) and RCT (0.65 Ω.cm2) as compared with graphene and MoS2 electrodes (Fig. S2 and Table S2). The low resistance values are beneficial, as they are responsible for the quick charge transfer and fast ion diffusion to enhance electrochemical performance. The dependence of the phase angle on the frequency is evaluated in the Bode plot as presented in Fig. 9b, where the ideal capacitor behavior is represented by the phase shift of −90° in the low-frequency region [49]. The MoS2/graphene composite electrode exhibits good capacitive performance (~ −75°), close to the ideal capacitor. Moreover, the relaxation time (τ = 1/f*) can be obtained from the frequency at a phase angle of −45° [50], to give more information regarding the frequency response of the electrode material. As shown in Fig. 9b, the MoS2/graphene composite electrode exhibits a frequency of 1.103 Hz, which corresponds to a time constant of 0.9 s. The small relaxation time indicates that the MoS2/graphene composite electrode can switch more rapidly from resistive to capacitive behavior. To investigate the electrochemical stability of the electrode material, the EIS test was employed after GCD stability, as shown in the inset in Fig. 9a. The RS and RCT values increase slightly after the GCD stability test, demonstrating the excellent electrochemical stability of the MoS2/graphene electrode [49].
Conclusions
In summary, a MoS2/graphene composite was produced by a facile electrochemical approach. The synergistic effect between MoS2 and graphene enhanced the charge storage of MoS2/graphene to yield high specific capacitance of 227 F g−1, which is higher than that of graphene (85 F g−1) and MoS2 (70 F g−1). In addition, the MoS2/graphene composite showed a long cycle life (89% after 2000 cycles) and low electrical resistance. These findings suggest that MoS2/graphene could be a potential electrode for high-performance supercapacitors.
References
Quan Q, Lin X, Zhang N et al (2017) Graphene and its derivatives as versatile templates for materials synthesis and functional applications. Nanoscale 9:2398–2416
Lee SP, Ali GAM, Algarni H et al (2019) Flake size-dependent adsorption of graphene oxide aerogel. J Mol Liq 277:175–180
Wang J, Luo Q, Luo C et al (2017) High-performance supercapacitor electrode based on a nanocomposite of polyaniline and chemically exfoliated MoS2 nanosheets. J Solid State Electrochem 21:2071–2077
Jia W, Tang B, Wu P (2018) Nafion-assisted exfoliation of MoS2 in water phase and the application in quick-response NIR light controllable multi-shape memory membrane. Nano Res 11:542–553
Li Y, Wang H, Xie L et al (2011) MoS2 nanoparticles grown on graphene: an advanced catalyst for the hydrogen evolution reaction. J Am Chem Soc 133:7296–7299
Tuteja SK, Duffield T, Neethirajan S (2017) Liquid exfoliation of 2D MoS2 nanosheets and their utilization as a label-free electrochemical immunoassay for subclinical ketosis. Nanoscale 9:10886–10896
Ganatra R, Zhang Q (2014) Few-layer MoS2: a promising layered semiconductor. ACS Nano 8:4074–4099
Aboelazm EAA, Ali GAM, Algarni H et al (2018) Flakes size-dependent optical and electrochemical properties of MoS2. Curr Nanosci 14:416–420
Theerthagiri J, Senthil R, Senthilkumar B et al (2017) Recent advances in MoS2 nanostructured materials for energy and environmental applications–a review. J Solid State Chem 252:43–71
Gusmão R, Sofer Z, Luxa J et al (2019) Antimony chalcogenide van der Waals nanostructures for energy conversion and storage. ACS Sustain Chem Eng 7:15790–15798
Zhang S, Song X, Liu S et al (2019) Template-assisted synthesized MoS2/polyaniline hollow microsphere electrode for high performance supercapacitors. Electrochim Acta 312:1–10
Raccichini R, Varzi A, Passerini S et al (2015) The role of graphene for electrochemical energy storage. Nat Mater 14:271–279
Ali GAM, Makhlouf SA, Yusoff MM et al (2015) Structural and electrochemical characteristics of graphene nanosheets as supercapacitor electrodes. Rev Adv Mater Sci 40:35–43
Liu N, Kim P, Kim JH et al (2014) Large-area atomically thin MoS2 nanosheets prepared using electrochemical exfoliation. ACS Nano 8:6902–6910
Ambrosi A, Pumera M (2018) Electrochemical exfoliation of MoS2 crystal for hydrogen electrogeneration. Chem Eur J 24:18551–18555
Jia Y, Wan H, Chen L et al (2017) Hierarchical nanosheet-based MoS2/graphene nanobelts with high electrochemical energy storage performance. J Power Sources 354:1–9
Achee TC, Sun W, Hope JT et al (2018) High-yield scalable graphene nanosheet production from compressed graphite using electrochemical exfoliation. Sci Rep 8:14525
Liu Y, Zhao Y, Jiao L et al (2014) A graphene-like MoS2/graphene nanocomposite as a highperformance anode for lithium ion batteries. J Mater Chem A 2:13109–13115
Chen Y, Ma W, Cai K et al (2017) In situ growth of polypyrrole onto three-dimensional tubular MoS2 as an advanced negative electrode material for supercapacitor. Electrochim Acta 246:615–624
Kee CW (2015) Assignment of O–O and Mo=O stretching frequencies of molybdenum/tungsten complexes revisited. J Chem 2015:439270–439279
da Silveira Firmiano EG, Rabelo AC, Dalmaschio CJ et al (2014) Supercapacitor electrodes obtained by directly bonding 2D MoS2 on reduced graphene oxide. Adv Energy Mater 4:1301380–1301387
Zhou K, Jiang S, Bao C et al (2012) Preparation of poly(vinyl alcohol) nanocomposites with molybdenum disulfide (MoS2): structural characteristics and markedly enhanced properties. RSC Adv 2:11695–11703
Teo EYL, Ali GAM, Algarni H et al (2019) One-step production of pyrene-1-boronic acid functionalized graphene for dopamine detection. Mater Chem Phys 231:286–291
Liu H, Chen B, Liao L et al (2019) The influences of mg intercalation on the structure and supercapacitive behaviors of MoS2. J Mater Sci 54:13247–13254
Tanhaei M, Mahjoub AR, Safarifard V (2019) Energy-efficient sonochemical approach for the preparation of nanohybrid composites from graphene oxide and metal-organic framework. Inorg Chem Commun 102:185–191
Zhang R, Wan W, Li D et al (2017) Three-dimensional MoS2/reduced graphene oxide aerogel as a macroscopic visible-light photocatalyst. Chin J Catal 38:313–320
Zhou Y, Zhang X, Zhang Q et al (2014) Role of graphene on the band structure and interfacial interaction of Bi2WO6/graphene composites with enhanced photocatalytic oxidation of NO. J Mater Chem A 2:16623–16631
Ali GAM, Fouad OA, Makhlouf SA (2013) Structural, optical and electrical properties of sol–gel prepared mesoporous Co3O4/SiO2 nanocomposites. J Alloys Compd 579:606–611
Wang Q, Huang J, Sun H et al (2018) MoS2 quantum dots@TiO2 nanotube arrays: an extended-spectrum-driven photocatalyst for solar hydrogen evolution. ChemSusChem 11:1708–1721
Abdi MM, Ekramul Mahmud HNM, Abdullah LC et al (2012) Optical band gap and conductivity measurements of polypyrrole-chitosan composite thin films. Chin J Polym Sci 30:93–100
Li Z, Xiang K, Xing W et al (2015) Reversible aluminum-ion intercalation in prussian blue analogs and demonstration of a high-power aluminum-ion asymmetric capacitor. Adv Energy Mater 5:1401410
Wang R, Wang S, Peng X et al (2017) Elucidating the intercalation pseudocapacitance mechanism of MoS2–carbon monolayer interoverlapped superstructure: toward high-performance sodium-ion-based hybrid supercapacitor. ACS Appl Mater Interfaces 9:32745–32755
Sarkar D, Das D, Das S et al (2019) Expanding interlayer spacing in MoS2 for realizing an advanced supercapacitor. ACS Energy Letters 4:1602–1609
Thalji MR, Ali GAM, Algarni H et al (2019) Al3+ ion intercalation pseudocapacitance study of W18O49 nanostructure. J Power Sources 438:227028
Aboelazm EAA, Ali GAM, Chong KF (2018) Cobalt oxide supercapacitor electrode recovered from spent lithium-ion battery. Chem Adv Mater 3:67–74
Ali GAM, Fouad OA, Makhlouf SA et al (2014) Co3O4/SiO2 nanocomposites for supercapacitor application. J Solid State Electrochem 18:2505–2512
Li Z, Zhou Z, Yun G et al (2013) High-performance solid-state supercapacitors based on graphene-ZnO hybrid nanocomposites. Nanoscale Res Lett 8:473
Fu M, Ge C, Hou Z et al (2013) Graphene/vanadium oxide nanotubes composite as electrode material for electrochemical capacitors. Phys B Condens Matter 421:77–82
Zhu J, He J (2012) Facile synthesis of graphene-wrapped honeycomb MnO2 nanospheres and their application in supercapacitors. ACS Appl Mater Interfaces 4:1770–1776
Chen S, Zhu J, Wu X et al (2010) Graphene oxide-MnO2 nanocomposites for supercapacitors. ACS Nano 4:2822–2830
Wang Q, Jiao L, Du H et al (2014) Fe3O4 nanoparticles grown on graphene as advanced electrode materials for supercapacitors. J Power Sources 245:101–106
Wu M-S, Lin C-J, Ho C-L (2012) Multilayered architecture of graphene nanosheets and MnO2 nanowires as an electrode material for high-performance supercapacitors. Electrochim Acta 81:44–48
Prabukumar C, Mohamed Jaffer Sadiq M, Krishna Bhat D et al (2019) SnO2 nanoparticles functionalized MoS2 nanosheets as the electrode material for supercapacitor applications. Mater Res Express 6:085526
Zhang F, Tang Y, Liu H et al (2016) Uniform incorporation of flocculent molybdenum disulfide nanostructure into three-dimensional porous graphene as an anode for high-performance lithium ion batteries and hybrid supercapacitors. ACS Appl Mater Interfaces 8:4691–4699
Patil S, Harle A, Sathaye S et al (2014) Development of a novel method to grow mono−/few-layered MoS2 films and MoS2–graphene hybrid films for supercapacitor applications. CrystEngComm 16:10845–10855
Xiao W, Zhou W, Feng T et al (2016) Simple synthesis of molybdenum disulfide/reduced graphene oxide composite hollow microspheres as supercapacitor electrode material. Materials 9:783–796
Zheng S, Zheng L, Zhu Z et al (2018) MoS2 nanosheet arrays rooted on hollow rGO spheres as bifunctional hydrogen evolution catalyst and supercapacitor electrode. Nano-Micro Lett 10:62–72
Wang J, Wu Z, Hu K et al (2015) High conductivity graphene-like MoS2/polyaniline nanocomposites and its application in supercapacitor. J Alloys Compd 619:38–43
Ali GAM, Manaf SAA, Divyashree A et al (2016) Superior supercapacitive performance in porous nanocarbons. J Energy Chem 25:734–739
Ali GAM, Yusoff MM, Shaaban ER et al (2017) High performance MnO2 nanoflower supercapacitor electrode by electrochemical recycling of spent batteries. Ceram Int 43:8440–8448
Acknowledgments
The authors would like to acknowledge the funding from the Ministry of Education Malaysia in the form of FRGS [RDU1901186: FRGS/1/2019/STG07/UMP/02/6] and Universiti Malaysia Pahang grant RDU170357. Moreover, the authors extend their appreciation to King Khalid University, the Ministry of Education–Kingdom of Saudi Arabia for supporting this research through a grant (RCAMS/KKU/002-18) under the Research Center for Advanced Material Science. In addition, Dr. Gomaa A. M. Ali would like to express his thanks to SESAME Synchrotron (Allan, Jordan), which through the EU-funded project OPEN SESAME provided training on material characterization testing and data analysis.
Author information
Authors and Affiliations
Corresponding author
Ethics declarations
Conflict of interest
The authors declare that they have no conflict of interest.
Additional information
Publisher’s note
Springer Nature remains neutral with regard to jurisdictional claims in published maps and institutional affiliations.
Highlights
• An MoS2/graphene composite is obtained via one-step electrochemical exfoliation.
• The MoS2/graphene composite is employed as electrode material for supercapacitor application.
• MoS2/graphene shows high specific capacitance of 227 F g−1 (threefold > MoS2 and graphene).
• MoS2/graphene shows high electrochemical stability and low electrical resistance.
Electronic supplementary material
ESM 1
(DOCX 410 kb)
Rights and permissions
About this article
Cite this article
Ali, G.A.M., Thalji, M.R., Soh, W.C. et al. One-step electrochemical synthesis of MoS2/graphene composite for supercapacitor application. J Solid State Electrochem 24, 25–34 (2020). https://doi.org/10.1007/s10008-019-04449-5
Received:
Revised:
Accepted:
Published:
Issue Date:
DOI: https://doi.org/10.1007/s10008-019-04449-5