Abstract
Key message
The increment duration and rate were equally important in controlling annual stem radial increments of Picea meyeri at its upper limit of distribution.
Abstract
Stem radial increment rate and duration are the most important parameters in determining the width of annual tree rings. To identify the contributions of rate and duration to annual radial increments and their relationships with environmental factors, we analyzed intra-annual stem increments of five Picea meyeri trees in a sub-alpine habitat of North-Central China over 7 years (2008–2015, except for the year 2012) with point dendrometers in this study. The results showed the following. (1) We estimated that approximately 53% of the variability in the annual radial increments is attributable to the rate of radial increment and approximately 47% to its duration. (2) The contribution of cessation time to the annual increments was more than three times that of the initiation time. (3) The initiation of radial increment was primarily controlled by soil temperature and warmer soil temperature could advance the initiation time. The cessation and rate of radial increment were mainly influenced by thermal and light-related environmental factors. During growing seasons, low temperatures and insufficient light caused by many rainy and cloudy days at the high altitudes of the Luya Mountains may result in earlier cessations and lower rates of radial increment. Overall, our results may have further applications in modeling the responses of tree stem growth to climate change in a sub-alpine habitat of North-Central China.
Similar content being viewed by others
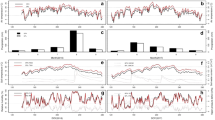
Explore related subjects
Discover the latest articles, news and stories from top researchers in related subjects.Avoid common mistakes on your manuscript.
Introduction
Forest growth is sensitive to climatic factors. Since the end of the past century, forest growth response to climate change has drawn increased global interest (Nabuurs et al. 2002). Based on meteorological data, climate warming trends over the past several decades have been reported in various regions (IPCC 2013), implying both rising temperatures and lengthening growing seasons. Some studies have demonstrated that the lengthening annual growing season of terrestrial vegetation in the Northern Hemisphere was mainly dependent on advanced growth initiation (Linderholm 2006; Menzel and Fabian 1999; Park et al. 2016), whereas recent studies reported that it was more dependent on delayed growth cessation after AD 2000 in most regions of the Northern Hemisphere (Garonna et al. 2014; Jeong et al. 2011). The benefit to forest growth due to a lengthening growing season remains unclear, because increasing respiration and drought risk may slow growth rate (Trahan and Schubert 2016).
It has been proved that terrestrial gross primary productivity (GPP) can be effectively estimated using maximum daily GPP and the initiation and cessation of the growing season (Xia et al. 2015; Zhou et al. 2016). Forests are the most important ecosystems for terrestrial productivity, and the intra-annual dynamic of wood formation represents the primary biological process through which carbon is sequestered in plants (Antonucci et al. 2017; Pan et al. 2011). The stem radial increment (ring width or xylem cell number) of trees could represent forest productivity due to the positive correlation between ring width and net ecosystem productivity (NEP) or GPP (Kulmala et al. 2017). The annual stem radial increment could also be estimated with increment initiation and cessation times and rates. Of the three parameters, initiation, and cessation times represent the duration of increment (the difference between cessation and initiation times), in which period the annual stem radial increment was generated, and increment rate represents tree physiological status. Similar annual increments can be achieved through different combinations of increment rate and duration, which are controlled by different environmental factors (Duchesne et al. 2012). The parameters of intra-annual stem radial increment dynamic (here, we refer to initiation, cessation, and rate of increment) have already been accurately quantified for many coniferous tree species using dendrometers or the micro-core technique (Cuny et al. 2012; Duchesne et al. 2012; Ren et al. 2015; Wang et al. 2015). With this information, researchers can estimate the relative contributions of rate and duration in modeling annual stem radial increment, i.e., tree-ring width or cell number (Cuny et al. 2012). For Picea abies, Pinus sylvestris, and Abies alba in Europe, where the mean annual temperature is approximately 10 °C and the mean annual precipitation exceeds 700 mm, higher annual increments have been mainly attributed to higher rates (Cuny et al. 2012; Rathgeber et al. 2011), but those of Picea mariana and Abies balsamea in North America, where the mean annual temperature ranges between − 2 and 4 °C and the mean annual precipitation exceeds 700 mm, have been mainly attributed to longer increment durations (Duchesne et al. 2012; Rossi et al. 2014). Furthermore, studies on the stem radial increment dynamic showed that in both Eurasia and North America, cessation time contributed more to annual increment than initiation time (Duchesne et al. 2012; Rossi et al. 2014).
A detailed analysis of the relationships between different environmental factors and increment dynamics may help us perceive the effects of changing climate on the growth of trees (Kulmala et al. 2017). However, the environmental influences on the dynamic of stem radial increments remain fragmentary. Recent studies found that at high altitudes/latitudes, increment initiation was mainly determined by when specific threshold air or soil temperatures occurred (Jiang et al. 2015; Li et al. 2017; Rossi et al. 2008). The maximum radial increment rate of conifers was either synchronized with the warmest temperatures (Makinen et al. 2003) or with the maximum day length (Rossi et al. 2006), which suggested that photoperiod or temperature could act as a growth constraint for increment rate (Makinen et al. 2003; Rossi et al. 2006). Stem increment cessation in autumn can be induced by cool temperatures or short photoperiod cessation (Ford et al. 2017).
To date, most prolonged period monitorings of intra-annual stem radial increment dynamics have been conducted in Europe and North America (Duchesne et al. 2012; Rossi et al. 2006). Although such projects in China have been conducted over recent years (Dong et al. 2011; Jiang et al. 2015; Li et al. 2017; Ren et al. 2015; Wang et al. 2015), long period data to support further research remain lacking. Considering that the response of radial increment to climate seems to be site- and species-dependent, we selected Picea meyeri in North-Central China to detect its radial increment dynamic in a sub-alpine habitat at the margin of the East Asian summer monsoon. North-Central China is a region where an increasing temperature trend is prominent in the Northern Hemisphere (Walther et al. 2002; Wang et al. 2012). P. meyeri is an important dominant coniferous species of the sub-alpine forests in this region (Wu 1980). As an endemic cold temperate evergreen conifer tree species, P. meyeri not only provides local residents with lumber production, but also acts as a major agent for soil and water resource conservation in mountainous areas (Liu 1996; Ma and Shangguan 2001). Because of its large biomass, P. meyeri also plays an important role in carbon storage for regional ecosystems. The mean annual temperature was below 0 °C and the mean annual precipitation was less 500 mm at this study site, which was different from that in Europe and North America. Therefore, we hypothesized that the contributions of radial increment rate and duration of P. meyeri to annual increment were different from those of tree species in Europe and North America. Specifically, we aim to (1) reveal the intra-annual radial increment dynamic of P. meyeri in a sub-alpine habitat, (2) assess the partial contributions of increment rate and duration to the annual radial increments, and (3) explore relationships between stem radial increment dynamics and environmental factors.
Materials and methods
Study site
The study site is located at 2737 m a.s.l. in the sub-alpine natural forest zone of the Luyashan Mountains in Shanxi Province (Fig. 1). The latitude and the longitude of the site are 38°44′N and 111°50′E, respectively. The study region is characterized by a semi-humid temperate monsoon climate. According to the automatic weather station (linear distance ca. 450 m south of the study site, 2771 m a.s.l., with recording beginning at the end of 2007) from 2008 to 2015, the mean annual temperature was − 1.48 °C, the mean annual cumulative precipitation was 439 mm, and over 80% of annual precipitation fell between May and September (Fig. 2). The vegetation is dominated by P. meyeri, and the canopy cover was approximately 70%, with almost no shrub layer and a sparse grass layer. The site corresponded to a type of abrupt treeline ecotone, and some solitary trees with a flag crown were found around the plot. At the site, a thick moss layer occurs in the understory, indicating a relatively moist environment. The site is on a north-exposed slope with an inclination of 20°.
Data collection
Measurements of stem radial increment changes
At the site, five P. meyeri trees were selected to install automatic point dendrometers (type: Radius Dendrometer, Ecomatik, Munich, Germany) to continuously measure stem radial changes from early September 2007. The selected trees were healthy adults with similar stem diameters and well-developed crowns and without visible wounds on their trunks. The average height of the selected trees was 5.87 ± 0.81 m (mean ± standard deviation), and the mean diameter was 16.3 ± 3.7 cm. According to cores taken from the selected trees at breast height, the mean age of the selected trees was 61 ± 15.1 years. Dendrometers were attached to stems at breast height. Before installing the dendrometers, the outer dead bark was carefully removed to reduce the influence of expansion and contraction processes of the bark (Deslauriers et al. 2003). The signal resolutions of the dendrometers were < 2.6 µm, and the thermal expansion coefficient of the sensor was < 0.1 µm °C− 1. Stem radial changes (µm) were registered automatically at 1-h intervals and saved in data loggers (DL15, Ecomatik, Munich, Germany). Because the thermal expansion coefficient was less than the signal resolution, no temperature correction was conducted. Daily means were calculated by averaging all daily measurements (24 values day− 1). Data were recorded from September 2007 to December 2015. The dendrometers were installed on the selected trees for a year round, and we periodically adjusted the tensions of the dendrometer sensor rods to maintain them within the manufacturer’s measurement range as the trees grew. The dendrometer data were not always continuous because of animal destruction, power failures, and other causes. The resulting data with gaps or abrupt jumps were checked carefully and corrected considering neighboring trees without corresponding inhomogeneities or replaced by linearly interpolated values (King et al. 2013; Korpela et al. 2010). We focused subsequent analysis over seven growing seasons (approximately from May to September) from 2008 to 2015. We note that the stem radial change data in the growing season of 2012 were lost completely due to animal destruction; therefore, the following analysis does not include stem radial changes from 2012.
Measurement of environmental factors
Environmental factors, including air temperature, soil temperature, and soil water content, were monitored at the study site. Air temperature (°C) was recorded at 1-h resolution using a sensor (Onset Corporation, HOBO Pro v2, U23-001) placed beneath the canopy 1.5 m above the ground. Soil water content (m3 m− 3; Theta Probes Type ML2x, Delta-T, Cambridge, England) and soil temperature (°C; ST1, Delta-T, Cambridge, England) were measured at approximately 10–20 cm depth and recorded every 1 h with a GP1 data logger (Delta-T, Cambridge, England). Daily precipitation (mm) was collected with an automatic weather station (Onset Corporation, HOBO U30-NRC) at a distance of 450 m from the study site. Daily photosynthetically active radiation (PAR) was estimated through sunshine hours of the nearest Wuzhai weather station (Zhu et al. 2010). The Pearson correlation coefficient between the estimated daily PAR and measured daily PAR at the study site for 2014 was 0.89 (p < 0.001, n = 365). The DOY (day of the year) when snow completely melted was approximately the day when soil thawed and water content increased dramatically.
Data analysis
High-frequency electronic dendrometer data have been widely used for observing initiations and cessations of tree stem radial increments (Ford et al. 2016, 2017). In this study, we fitted the intra-annual stem radial increments of P. meyeri with a Gompertz function during the growing seasons (May–September) (Rossi et al. 2006). To avoid arbitrary choices in the initial settings of the dendrometer measurements, an additional parameter (Y0) was used in the Gompertz equation (Formula 1) (Duchesne et al. 2012; Wang et al. 2015). The daily averaged raw measurement data used in fitting is
where Y represents the daily averaged cumulative increment, Y0 is the lower asymptote, A is the upper asymptote of the curve, β is the x axis placement parameter, κ is the rate of change parameter, and t is the day of the year (DOY). The parameters were estimated using an ordinary least squares method with the nonlinear regression procedure included in the Origin software package (OriginLab Corporation, Northampton, MA, USA). A total of 35 models were established for each individual tree (n = 5) and each growing season (n = 7) (see Table S1 available as Supplementary Data) (Duchesne et al. 2012; Linares et al. 2009). To evaluate the general goodness-of-fit of each regression, the residual distribution and resulting R2 were calculated (Michelot et al. 2012; Rossi et al. 2006).
Based on the Gompertz models, the weighted mean absolute rate of stem radial increment (R) and time for the maximum daily increment (TP) were calculated using the following equations (Formulae 2 and 3):
where the parameter ν was set to 0.0001 following Deslauriers et al. (2003) and Rossi et al. (2008).
For the dendrometer method, because we lack direct observations of dynamic cambium activity, the precise dates of stem radial increment initiation and cessation cannot be determined unambiguously; therefore, researchers use different estimation methods (Deslauriers et al. 2007; Duchesne et al. 2012; Lempereur et al. 2015; Makinen et al. 2008; Zweifel et al. 2010). Because the sample trees have different growth rates and stem diameters, it is difficult to compare their wood formation dynamics with cell numbers or microns (Jyske et al. 2014). To make the sample trees commensurable, we decided to transfer the cumulative stem radial increments into cumulative percentages based on curves modeled by the Gompertz function in this study (Jones et al. 2004; Jyske et al. 2014), and the DOY of initiation and cessation of stem radial increment were defined as the days when 5 and 95% of the final annual cumulative stem radial increment were reached, respectively (Gutierrez et al. 2011; Jyske et al. 2012). Consequently, duration of radial stem increment was calculated as the number of days between the dates of initiation and cessation. The DOY of increment initiation and cessation, days of increment duration, increment rate and annual increment of five sample trees in each year were averaged for the following analysis, because we were interested in the differences in the above three parameters among different years rather than individual trees.
Principal components analysis (PCA) was used to determine the relationships between increment initiation time, cessation time, rate, increment duration, and annual increment with CANOCO 5 (Microcomputer Power Company, Ithaca, NY, USA) (Smilauer and Lepš 2014). The components considered were determined on the basis of the Kaiser–Guttman criterion (eigenvalues > 1) (Cocozza et al. 2016). The log-transformed values of increment initiation time, cessation time, increment rate, increment duration and annual increment were used. These five parameters were depicted as vectors, and the directions of the vector values and the correlations among vectors and component axes were determined. Two simple physical models were applied to assess the relative contributions of increment initiation and cessation to increment duration (Formula 4) and increment initiation, cessation and rate to annual increment (Formula 5) (Cuny et al. 2014). A linear regression analysis was used to examine the relative contributions of increment initiation and cessation to duration and increment initiation, cessation and rate to annual increment (Rossi et al. 2014; Xia et al. 2015). The relative contribution of each independent variable to the dependent variable was evaluated by means of the relative weights method (Johnson and LeBreton 2004).
TI represents increment initiation time, TC represents increment cessation time, R represents increment rate, TD represents increment duration and AI represents annual increment.
To date, only temperature and precipitation have been commonly used in climate-growth relationship studies. However, factors influencing tree growth processes are known to be diversified (Dufour and Morin 2013). The most important factors include temperature, water and light-related environmental variables. We chose the environmental variables in Table 1 to explore the relationships between increment initiation, cessation and rate and environmental factors based on the expected effects in regulating seasonal tree growth processes in a cold environment according to Duchesne et al. (2012) and Dufour and Morin (2013), such as that temperatures and photoperiods have been posited as cues for tree growth initiation and cessation (Ford et al. 2016, 2017). These relationships were explored through the multiple regression model method. Every possible model was inspected by means of subset regression using the “leaps” package in the R procedure (Kabacoff 2015). The best models were selected based on the Akaike Information Criterion (AIC) (Akaike 1998).
We used logistic regression (the GLM package in R, 2017) to calculate the probability of stem radial increment being active at a given temperature in spring (Rossi et al. 2008). Binary responses were coded as non-active (value 0) or active (value 1). For each tree and year, the model was fitted with the respective temperature series (mean, minimum and maximum air temperatures and mean soil temperature), and temperature thresholds were calculated when the probability of radial increment being active was 0.5. Fitting verification included χ2 of the likelihood ratio, Wald’s χ2 for regression parameter and goodness of fit, and Hosmer–Lemeshow Ĉ for eventual lack of fit. None of the models were excluded because of a lack of fit. One-way analysis of variance (ANOVA) and Tukey multiple comparisons were carried out to test for any significant differences in the estimated thresholds among years. Normality and variance homogeneity were checked using Shapiro–Wilk and Bartlett tests.
Because over 50% of annual increment was produced within 30 days around the DOY of fast increment rate, we extracted the average increment during the 30 days around the DOY of fast increment rate (AIRmax) and corresponding environmental factors (see Table S2 available as Supplementary Data). We then calculated the Pearson correlation coefficients between AIRmax and the environmental factors.
Results
Intra-annual stem radial increment dynamic characteristics
The daily mean accumulated stem radial increments modeled using the Gompertz function (Formula 1) and associated increment rates are illustrated in Fig. 3. Intra-annual accumulated increments were well fit by the Gompertz function, which explained 81–98% of the variations in seasonal stem radial changes (see Table S1 available as Supplementary Data). Several characteristics describing seasonal increment progress are defined in Table 2. Initiation of stem radial increment was at approximately DOY 150 (May 30), whereas the increment cessation was at approximately DOY 226 (August 14). The mean duration of annual stem radial increment was approximately 76 days. The mean seasonal stem radial increment was approximately 856 µm. The mean stem radial increment rate was approximately 16.6 µm day− 1. A fast increment occurred at approximately DOY 170 (June 19), which was not significantly different with the DOY of the summer solstice (DOY 172 or 173, June 21 or 22, respectively) (one-sample t test, p < 0.05). Most of the annual accumulated increments were completed in June and July (approximately 43 and 33%, respectively).
Relative contribution of rate and duration to annual increment
Two simple physical models, TD =\(~f\)(TI, TC) and AI =\(~f\)(TI, TC, R), yielded good results in modeling mean increment duration and annual increment, with R2 exceeding 99% (p < 0.01, n = 7). The annual increment showed a stronger relationship with rate than with initiation and cessation times (Fig. 4). The relative contributions of increment rate, initiation time, and cessation time to annual increment variance were 53, 11, and 36%, respectively (Fig. 5, red line). Increment duration showed a stronger relationship with cessation time (Fig. 4). The relative contribution of cessation time to the variance in increment duration was 62%, which was much larger than for initiation time and contributed 38% to the variance in increment duration (Fig. 5, blue line).
Threshold temperatures
The threshold air and soil temperatures at which there was a 0.5 probability of stem radial increment at the study site are depicted in Fig. 6. On one hand, a significant difference was found in the daily air temperature thresholds for TI among the 7 years (ANOVA with Tukey post hoc test, p < 0.05). On the other hand, there were no significant differences in the soil temperature thresholds for TI among the 7 year, and the soil temperature threshold was approximately 1.8 ± 0.6 °C (ANOVA, p > 0.05).
Threshold minimum, mean, and maximum air temperatures and mean soil temperature corresponding to a 0.5 probability of radial increment. The error bars indicate the standard deviation among trees. The lowercase letters (a, b) indicate significant differences among years based on Tukey post hoc test (p < 0.05)
Relationships between stem radial increments and environmental factors
The relationships between the main stem radial increment dynamic parameters (initiation time, cessation time and rate of increment) and environmental factors were explored using a multivariate regression method (Table 3; Fig. 7). Increment initiation time showed a close relationship with thermal related environmental factors, i.e., the first DOY with soil temperature > 1 °C, the first DOY with PAR > 500 µmol s− 1 m− 2, and the DOY when snowmelt was complete (Table 3; Fig. 7a, p < 0.05, R2 = 0.937, p < 0.05). Among the three above environmental variables, first DOY with soil temperature > 1 °C was the primary variable with the increment initiation time independent explanation variance exceeding 56%. Increment cessation time was influenced by precipitation from May to August (Table 3; Fig. 7b, R2 = 0.781, p < 0.01). Mean increment rate was controlled by cumulative PAR from May to August (Table 3; Fig. 7c, R2 = 0.793, p < 0.01). Increment during the 30 days around the DOY of fast increment rate was negatively correlated with air relative humidity (r = − 0.933, p < 0.01) and positively correlated with photosynthetically active radiation (r = 0.954, p < 0.01) and soil temperature (r = 0.767, p < 0.05) (Table 3).
Discussion
Contributions of increment rate and time to annual stem radial increment
In this study, the increment duration and rate of P. meyeri are both important in explaining the variance of annual stem radial increments; the contributions of increment duration and rate to annual increment, 47 and 53%, respectively, were similar (Fig. 5). This result differs from those of studies of evergreen conifer trees in Europe and North America. For European spruce (Picea abies), Scots pine (Pinus sylvestris), and silver fir (Abies alba) in Europe, rate contributed 75% to annual increment variance, and duration contributed only 25% (Cuny et al. 2012; Rathgeber et al. 2011). However, for black spruce (Picea mariana) and balsam fir (Abies balsamea) in Québec, Canada, annual increment was more sensitive to duration than rate. Contributions of increment duration and rate to annual increment variance were 86% and 14% for black spruce (Rossi et al. 2014) and 76% and 24% for balsam fir, respectively (Duchesne et al. 2012). Different contributions of increment rate and duration to annual increment may indicate different adjustments to adapt to local environments by tree species in different climate regions (Cuny et al. 2012).
Equal inter-annual variances in radial increment initiation and cessation might generate different stem radial increment changes. In our study, the contributions of increment initiation and cessation to annual increment were 11% and 36%, respectively. This result means that the contribution of cessation time to annual increment was 3 times more than that of initiation time. This phenomenon was also found in A. balsamea trees in Québec, Canada, where the contribution of cessation time (61%) to annual increment was 4 times more than that of initiation time (15%) (Duchesne et al. 2012). We also calculated the Pearson correlation coefficients between annual increment for the seven conifer species in the northern hemisphere and increment initiation and cessation time, respectively (Rossi et al. 2008). The result showed that annual increment had a closer relationship with increment cessation time (r = 0.65, n = 16, p < 0.01) than with initiation time (r = − 0.18, n = 16, p = 0.50). These results imply that cessation time is more important than initiation in explaining annual increment variance. Therefore, in evaluating the influence of climate warming on forest ecosystem productivity, more attention should be paid to the influences of climate change on increment rate and cessation time. It should be noted that Lupi et al. (2010) reported that higher numbers of radial cells along tree rings produce delays in the end of xylem maturation, which was defined as the termination of cell wall thickening and lignification. However, in our study, radial increment cessation was defined as when tree-ring width stops increasing, which approximately corresponds to when xylem cells stop enlarging and is much earlier than when cell walls complete thickening and lignification (Cuny et al. 2015).
Contributions of increment initiation and cessation time to increment duration
Increment duration is the synthesis of initiation and cessation. Understanding relative contributions of increment initiation and cessation times to duration is an important step towards predicting future increment duration changes under the influence of global climate warming (Garonna et al. 2016). In this study, increment cessation time contributed more (62%) to duration than initiation time (38%) (Fig. 5). A similar result was found for A. balsamea in Québec; increment cessation time contributed more (79%) to duration than initiation time (21%) (Duchesne et al. 2012). For the seven conifer species in Europe and Canada (Rossi et al. 2008), the Pearson correlation coefficients between increment duration and initiation and cessation time were − 0.64 and 0.78 (n = 16, p < 0.01), respectively. These results indicate that increment duration variance was mainly driven by the change in cessation time.
Previous studies have verified the existence of a critical temperature for increment initiation, but the factors influencing increment cessation were more complex (Rossi et al. 2008). Therefore, variance in increment cessation time could be higher than for initiation time. Consequently, increment cessation time contributed more to duration than initiation time. According to temperature recordings at our study site, the daily mean air temperature was 10.5 °C when P. meyeri stem radial increment finished increasing, which was much higher than the temperature when stem radial increment started increasing (6.4 °C). The standard deviation of increment cessation time for P. meyeri was 16 days, which was higher than that of initiation time (12 days). Rossi et al. (2008) obtained similar results from a study of seven conifer species in Europe and Canada, where the mean daily air temperatures at increment cessation and initiation times were 13.8 and 8.4 °C, and the standard deviations of the increment cessation and initiation times were 10 and 8 days, respectively. The standard deviations of the increment cessation and initiation times were 16 and 7 days, respectively, for A. balsamea in Québec, Canada (Duchesne et al. 2012).
Main environment factors influencing stem radial increment for P. meyeri
It has been well established that temperature strongly effects growth resumption after winter dormancy in plants (Körner 2006; Swidrak et al. 2013). However, it is still inconclusive whether soil temperature or air temperature affects the initiation of tree radial increment. Different from some studies that reported that xylogenesis was initiated when air temperature rose to specific thresholds (Rossi et al. 2008; Li et al. 2017), we found in this study that stem radial increment for P. meyeri was initiated when the soil temperature increased approximately 1.8 °C (Fig. 6). By contrast, the air temperature thresholds for increment initiation were significantly different among the 7 years. This indicates that the most important environmental factor that influenced increment initiation in P. meyeri was soil temperature. The result of the multiple regression of increment initiation time in relation to the environmental variables also confirmed that warmer soil temperatures caused initiation to advance (Table 3). Warm soil temperatures accelerate root activity and promote water and nutrient uptake, thus influencing cell turgor in the cambium and advancing reaction of the cambial cell. Our previous studies on intra-annual stem radial increment in P. meyeri and Larix principis-rupprechtii int the Luyashan Mountains using point dendrometers and microcores also indicated that soil temperature determined the initiation of stem radial increment (Dong et al. 2011; Jiang et al. 2015). Previous studies have suggested that cessation of radial increment was mainly influenced by photoperiods or temperature (Fromm 2013; de Andrés et al. 2015). In our study, precipitation during the growing season led to an earlier cessation of radial increment in P. meyeri (Table 3). This was consistent with a previous study based on remote sensing, where it was found that precipitation had negative effects on the end of the vegetation growing season in colder regions (Liu et al. 2016). We speculated that this may be related to the close relationships between precipitation, light, and temperature. More precipitation at high altitudes in the Luya Mountains may lead to a shortage of thermal and radiation energy for photosynthesis during the growing season, consequently resulting in an earlier cessation of growth. The close relationship between precipitation and cessation of increment found in this study was not contradictory to any previous findings. This explanation was also suggested by a previous tree-ring study on P. meyeri at high altitudes in the Luya Mountains, which reported that radial growth for this species was negatively correlated with precipitation in June and July of the same year (Zhang et al. 2012). Increment rate of P. meyeri at high altitude showed a strong relationship with PAR during the growing season (Table 3). At the same time, increment during 30 days at a fast increment rate was significantly and positively correlated with PAR (r = 0.954, p = 0.001), but the relationship with air temperature was not significant (Table 4). At high altitudes, radiation intensity is usually higher and plants usually have a higher photosynthetic capacity, but an increase in cloudy and rainy days would decrease total radiation (Körner 2003). Therefore, an appropriate increase in PAR could promote photosynthesis and raise the increment rate. The annual increment was mainly determined by the number of tracheids; therefore, increment rate directly reflected cambium cell division rate. Above all, insufficient solar radiation at high altitudes may be among the primary factors influencing P. meyeri radial growth.
Previous studies on climate change in the study region have already demonstrated an increasing trend in mean annual air temperature (0.3 °C·decade− 1) with a decreasing trend in annual total precipitation (13 mm·decade− 1) over the past several decades (Zhang et al. 2013) and a decreasing trend in annual sunshine duration of 65 h·decade− 1 over the past 50 years (Fan and Wang 2010). Considering the positive effects of temperature and PAR on P. meyeri radial increment at high altitudes, if the current climate trend continues, increasing temperature and decreasing precipitation could extend growing season lengths. However, these positive effects on stem radial increment of trees distributed in sub-alpine regions may be offset by the negative effects of the decreases in solar radiation on increment rate.
Author contribution statement
Yuan Jiang and Mingchang Wang were responsible for the research design and the editing coordination of the paper. Data preparation and analysis were partitioned as follows: site inventory and data collection (Mingchang Wang, Wentao Zhang, Manyu Dong, Biao Wang, Yiping Zhang, and Xinyuan Ding); data analysis (Wentao Zhang, Mingchang Wang, Manyu Dong and Hui Xu). Mingchang Wang, Yuan Jiang, Hui Xu and Muyi Kang contributed to editing and reviewing the manuscript.
References
Akaike H (1998) Information theory and an extension of the maximum likelihood principle. Selected papers of Hirotugu Akaike. Springer, Berlin, pp 199–213
Antonucci S, Rossi S, Deslauriers A, Morin H, Lombardi F, Marchetti M, Tognetti R (2017) Large-scale estimation of xylem phenology in black spruce through remote sensing. Agric For Meteorol 233:92–100
Cocozza C, Palombo C, Tognetti R, La Porta N, Anichini M, Giovannelli A, Emiliani G (2016) Monitoring intra-annual dynamics of wood formation with microcores and dendrometers in Picea abies at two different altitudes. Tree Physiol 36:832–846
Cuny HE, Rathgeber CBK, Lebourgeois F, Fortin M, Fournier M (2012) Life strategies in intra-annual dynamics of wood formation: example of three conifer species in a temperate forest in north-east France. Tree Physiol 32:612–625
Cuny HE, Rathgeber CB, Frank D, Fonti P, Fournier M (2014) Kinetics of tracheid development explain conifer tree-ring structure. New Phytol 203:1231–1241
Cuny HE, Rathgeber CBK, Frank D, Fonti P, Mäkinen H, Prislan P, Rossi S, Del Castillo EM, Campelo F, Vavrčík H, Camarero JJ, Bryukhanova MV, Jyske T, Gričar J, Gryc V, De Luis M, Vieira J, Čufar K, Kirdyanov AV, Oberhuber W, Treml V, Huang J, Li X, Swidrak I, Deslauriers A, Liang E, Nöjd P, Gruber A, Nabais C, Morin H, Krause C, King G, Fournier M (2015) Woody biomass production lags stem-girth increase by over one month in coniferous forests. Nat Plants. https://doi.org/10.1038/nplants.2015.160
de Andrés EG, Camarero JJ, Büntgen U (2015) Complex climate constraints of upper treeline formation in the Pyrenees. Trees Struct Funct 29:941–952
Deslauriers A, Morin H, Urbinati C, Carrer M (2003) Daily weather response of balsam fir (Abies balsamea (L.) Mill.) stem radius increment from dendrometer analysis in the boreal forests of Québec (Canada). Trees Struct Funct 17:477–484
Deslauriers A, Rossi S, Anfodillo T (2007) Dendrometer and intra-annual tree growth: what kind of information can be inferred? Dendrochronologia 25:113–124
Dong M, Jiang Y, Zhang W, Yang Y, Yang H (2011) Effect of alpine treeline conditions on the response of the stem radial variation of Picea meyeri Rebd. et Wils to environmental factors. Pol J Ecol 59:729–739
Duchesne L, Houle D, D Orangeville L (2012) Influence of climate on seasonal patterns of stem increment of balsam fir in a boreal forest of Québec, Canada. Agric For Meteorol 162–163:108–114
Dufour B, Morin H (2013) Climatic control of tracheid production of black spruce in dense mesic stands of eastern Canada. Tree Physiol 33:175–186
Fan X, Wang M (2010) Temporal and spatial variations in sunshine duration during 1959–2008 over Shanxi, China. Ecol Environ Sci 19:605–609 (Chinese, with English abstract)
Ford KR, Harrington CA, Bansal S, Gould PJ, St. Clair JB (2016) Will changes in phenology track climate change? A study of growth initiation timing in coast Douglas-fir. Glob Change Biol 22:3712–3723
Ford KR, Harrington CA, St. Clair JB (2017) Photoperiod cues and patterns of genetic variation limit phenological responses to climate change in warm parts of species’ range: modeling diameter-growth cessation in coast Douglas-fir. Glob Change Biol 23:3348–3362
Fromm J (2013) Xylem development in trees: from cambial divisions to mature wood cells. In: Fromm J (ed) Cellular aspects of wood formation. Springer, Berlin, pp 3–39
Garonna I, De Jong R, De Wit AJW, Mucher CA, Schmid B, Schaepman ME (2014) Strong contribution of autumn phenology to changes in satellite-derived growing season length estimates across Europe (1982–2011). Glob Change Biol 20:3457–3470
Garonna I, de Jong R, Schaepman ME (2016) Variability and evolution of global land surface phenology over the past three decades (1982–2012). Glob Change Biol 22:1456–1468
Gutierrez E, Campelo F, Julio Camarero J, Ribas M, Muntan E, Nabais C, Freitas H (2011) Climate controls act at different scales on the seasonal pattern of Quercus ilex L. stem radial increments in NE Spain. Trees Struct Funct 25:637–646
IPCC (2013) Climate change 2013: The physical science basis. Cambridge University Press, Cambridge, New York
Jeong S, Ho C, Gim H, Brown ME (2011) Phenology shifts at start vs. end of growing season in temperate vegetation over the Northern Hemisphere for the period 1982–2008. Glob Change Biol 17:2385–2399
Jiang Y, Zhang Y, Guo Y, Kang M, Wang M, Wang B (2015) Intra-annual xylem growth of Larix principis-rupprechtii at Its upper and lower distribution limits on the Luyashan mountain in North-Central China. Forests 6:3809–3827
Johnson JW, LeBreton JM (2004) History and use of relative importance indices in organizational research. Organ Res Methods 7:238–257
Jones B, Tardif J, Westwood R (2004) Weekly xylem production in trembling aspen (Populus tremuloides) in response to artificial defoliation. Can J Bot 82:590–597
Jyske T, Manner M, Mäkinen H, Nöjd P, Peltola H, Repo T (2012) The effects of artificial soil frost on cambial activity and xylem formation in Norway spruce. Trees Struct Funct 26:405–419
Jyske T, Mäkinen H, Kalliokoski T, Nöjd P (2014) Intra-annual tracheid production of Norway spruce and Scots pine across a latitudinal gradient in Finland. Agric For Meteorol 194:241–254
Kabacoff R (2015) R in action: data analysis and graphics with R: Manning Publications Co, New York
King G, Fonti P, Nievergelt D, Büntgen U, Frank D (2013) Climatic drivers of hourly to yearly tree radius variations along a 6 °C natural warming gradient. Agric For Meteorol 168:36–46
Körner C (2003) Alpine plant life: functional plant ecology of high mountain ecosystems. Springer Science & Business Media, Berlin
Körner C (2006) Significance of temperature in plant life. In: Morison J.I.L., Morecroft MD (eds) Plant growth and climate change. Blackwell, Oxford, pp 48–69
Korpela M, Mäkinen H, Nöjd P, Hollmén J, Sulkava M (2010) Automatic detection of onset and cessation of tree stem radius increase using dendrometer data. Neurocomputing 73:2039–2046
Kulmala L, Read J, Nöjd P, Rathgeber CBK, Cuny HE, Hollmén J, Mäkinen H (2017) Identifying the main drivers for the production and maturation of Scots pine tracheids along a temperature gradient. Agric For Meteorol 232:210–224
Lempereur M, Martin-StPaul NK, Damesin C, Joffre R, Ourcival J, Rocheteau A, Rambal S (2015) Growth duration is a better predictor of stem increment than carbon supply in a Mediterranean oak forest: implications for assessing forest productivity under climate change. New Phytol 207:579–590
Li X, Liang E, Ar JG, Rossi S, Ufar K (2017) Critical minimum temperature limits xylogenesis and maintains treelines on the southeastern Tibetan Plateau. Sci Bull 62:804–812
Linares JC, Camarero JJ, Carreira JA (2009) Plastic responses of Abies pinsapo xylogenesis to drought and competition. Tree Physiol 29:1525–1536
Linderholm HW (2006) Growing season changes in the last century. Agric For Meteorol 137:1–14
Liu L (1996) Vegetation of hebei province. Science Press, Beijing (in Chinese)
Liu Q, Fu YH, Zhu Z, Liu Y, Liu Z, Huang M, Janssens IA, Piao S (2016) Delayed autumn phenology in the Northern Hemisphere is related to change in both climate and spring phenology. Glob Change Biol 22:3702–3711
Lupi C, Morin H, Deslauriers A, Rossi S (2010) Xylem phenology and wood production: resolving the chicken-or-egg dilemma. Plant Cell Environ 33:1721–1730
Ma Z, Shangguan GT (2001) Vegetation of shanxi province. Science and Technology Press, Beijing (Chinese)
Makinen H, Nojd P, Saranpaa P (2003) Seasonal changes in stem radius and production of new tracheids in Norway spruce. Tree Physiol 23:959–968
Makinen H, Seo J, Nojd P, Schmitt U, Jalkanen R (2008) Seasonal dynamics of wood formation: a comparison between pinning, microcoring and dendrometer measurements. Eur J For Res 127:235–245
Menzel A, Fabian P (1999) Growing season extended in Europe. Nature 397:659–659
Michelot A, Simard S, Rathgeber C, Dufrene E, Damesin C (2012) Comparing the intra-annual wood formation of three European species (Fagus sylvatica, Quercus petraea and Pinus sylvestris) as related to leaf phenology and non-structural carbohydrate dynamics. Tree Physiol 32:1033–1045
Nabuurs G, Pussinen A, Karjalainen T, Erhard M, Kramer K (2002) Stemwood volume increment changes in European forests due to climate change-a simulation study with the EFISCEN model. Glob Change Biol 8:304–316
Pan Y, Birdsey RA, Fang J, Houghton R, Kauppi PE, Kurz WA, Phillips OL, Shvidenko A, Lewis SL, Canadell JG, Ciais P, Jackson RB, Pacala SW, McGuire AD, Piao S, Rautiainen A, Sitch S, Hayes D (2011) A large and persistent carbon sink in the World’s Forests. Science 333:988–993
Park T, Ganguly S, Tommervik H, Euskirchen ES, Hogda K, Karlsen SR, Brovkin V, Nemani RR, Myneni RB (2016) Changes in growing season duration and productivity of northern vegetation inferred from long-term remote sensing data. Environ Res Lett 11:084001
R Core Team (2017) R: a language and environment for statistical computing. R Foundation for Statistical Computing, Vienna, Austria. http://www.R-project.org/. Accessed 14 Oct 2017
Rathgeber CBK, Rossi S, Bontemps JD (2011) Cambial activity related to tree size in a mature silver-fir plantation. Ann Bot 108:429–438
Ren P, Rossi S, Gricar J, Liang E, Cufar K (2015) Is precipitation a trigger for the onset of xylogenesis in Juniperus przewalskii on the north-eastern Tibetan Plateau? Ann Bot 115:629–639
Rossi S, Deslauriers A, Anfodillo T, Morin H, Saracino A, Motta R, Borghetti M (2006) Conifers in cold environments synchronize maximum growth rate of tree-ring formation with day length. New Phytol 170:301–310
Rossi S, Deslauriers A, Griçar J, Seo J, Rathgeber CB, Anfodillo T, Morin H, Levanic T, Oven P, Jalkanen R (2008) Critical temperatures for xylogenesis in conifers of cold climates. Global Ecol Biogeogr 17:696–707
Rossi S, Girard M, Morin H (2014) Lengthening of the duration of xylogenesis engenders disproportionate increases in xylem production. Glob Change Biol 20:2261–2271
Smilauer P, Lepš J (2014) Multivariate analysis of ecological data using CANOCO 5. Cambridge University Press, Cambridge
Swidrak I, Schuster R, Oberhuber W (2013) Comparing growth phenology of co-occurring deciduous and evergreen conifers exposed to drought. Flora 208:609–617
Trahan MW, Schubert BA (2016) Temperature-induced water stress in high-latitude forests in response to natural and anthropogenic warming. Glob Change Biol 22:782–791
Walther GR, Post E, Convey P, Menzel A, Parmesan C, Beebee T, Fromentin JM, Hoegh-Guldberg O, Bairlein F (2002) Ecological responses to recent climate change. Nature 416:389–395
Wang Q, Fan X, Qin Z, Wang M (2012) Change trends of temperature and precipitation in the Loess Plateau Region of China, 1961–2010. Glob Planet Change 92:138–147
Wang Z, Yang B, Deslauriers A, Bräuning A (2015) Intra-annual stem radial increment response of Qilian juniper to temperature and precipitation along an altitudinal gradient in northwestern China. Trees Struct Funct 29:25–34
Wu Z (1980) Vegetation of China. Science Press, Beijing (Chinese)
Xia J, Niu S, Ciais P, Janssens IA, Chen J, Ammann C, Arain A, Blanken PD, Cescatti A, Bonal D, Buchmann N, Curtis PS, Chen S, Dong J, Flanagan LB, Frankenberg C, Georgiadis T, Gough CM, Hui D, Kiely G, Li J, Lund M, Magliulo V, Marcolla B, Merbold L, Montagnani L, Moors EJ, Olesen JE, Piao S, Raschi A, Roupsard O, Suyker AE, Urbaniak M, Vaccari FP, Varlagin A, Vesala T, Wilkinson M, Weng E, Wohlfahrt G, Yan L, Luo Y (2015) Joint control of terrestrial gross primary productivity by plant phenology and physiology. Proc Natl Acad Sci USA 112:2788–2793
Zhang W, Jiang Y, Dong M, Kang M, Yang H (2012) Relationship between the radial growth of Picea meyeri and climate along elevations of the Luyashan Mountain in North-Central China. For Ecol Manage 265:142–149
Zhang L, Yan J, Liu L (2013) Climate change and drought and flood disasters trend in Shanxi. J Arid Land Res Environ 27:120–125 (Chinese)
Zhou S, Zhang Y, Caylor KK, Luo Y, Xiao X, Ciais P, Huang Y, Wang G (2016) Explaining inter-annual variability of gross primary productivity from plant phenology and physiology. Agric For Meteorol 226–227:246–256
Zhu X, He H, Liu M, Yu G, Sun X, Gao Y (2010) Spatio-temporal variation of photosynthetically active radiation in China in recent 50 years. J Geog Sci 20:803–817
Zweifel R, Eugster W, Etzold S, Dobbertin M, Buchmann N, Hasler R (2010) Link between continuous stem radius changes and net ecosystem productivity of a subalpine Norway spruce forest in the Swiss Alps. New Phytol 187:819–830
Acknowledgements
We are grateful to Liang Jiao, Haochun Yang, Yan’gang Yang, Yan Wen, Zijian Zhou and Yuanyuan Guo for their help in the fieldwork. This work was supported by the National Science Foundation of China (Grant Nos. 41630750 and 41771051). Special thanks to the anonymous referees for their valuable comments and suggestions.
Author information
Authors and Affiliations
Corresponding author
Ethics declarations
Conflict of interest
The authors declare that they have no conflict of interest.
Additional information
Communicated by E. Liang.
Electronic supplementary material
Below is the link to the electronic supplementary material.
Rights and permissions
About this article
Cite this article
Wang, M., Jiang, Y., Dong, M. et al. The contributions of rate and duration of stem radial increment to annual increments of Picea meyeri in a sub-alpine habitat, North-Central China. Trees 32, 1029–1041 (2018). https://doi.org/10.1007/s00468-018-1693-0
Received:
Accepted:
Published:
Issue Date:
DOI: https://doi.org/10.1007/s00468-018-1693-0