Abstract
Trichomoniasis is a great public health burden worldwide and the increase in treatment failures has led to a need for finding alternative molecules to treat this disease. In this study, we present in vitro and in silico analyses of two 2,8-bis(trifluoromethyl) quinolines (QDA-1 and QDA-2) against Trichomonas vaginalis. For in vitro trichomonacidal activity, up to seven different concentrations of these drugs were tested. Molecular docking, biochemical, and cytotoxicity analyses were performed to evaluate the selectivity profile. QDA-1 displayed a significant effect, completely reducing trophozoites viability at 160 µM, with an IC50 of 113.8 µM, while QDA-2 at the highest concentration reduced viability by 76.9%. QDA-1 completely inhibited T. vaginalis growth and increased reactive oxygen species production and lipid peroxidation after 24 h of treatment, but nitric oxide accumulation was not observed. In addition, molecular docking studies showed that QDA-1 has a favorable binding mode in the active site of the T. vaginalis enzymes purine nucleoside phosphorylase, lactate dehydrogenase, triosephosphate isomerase, and thioredoxin reductase. Moreover, QDA-1 presented a level of cytotoxicity by reducing 36.7% of Vero cells’ viability at 200 µM with a CC50 of 247.4 µM and a modest selectivity index. In summary, the results revealed that QDA-1 had a significant anti-T. vaginalis activity. Although QDA-1 had detectable cytotoxicity, the concentration needed to eliminate T. vaginalis trophozoites is lower than the CC50 encouraging further studies of this compound as a trichomonacidal agent.
Similar content being viewed by others
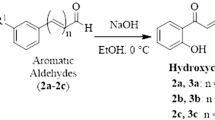
Avoid common mistakes on your manuscript.
Introduction
Trichomoniasis is the most incident and prevalent non-viral sexually transmitted infection (STI) worldwide (WHO 2012, 2018; Menezes et al. 2016). This STI is associated with HIV acquisition and transmission (McClelland et al. 2007; van der Pol et al. 2008; Mavedzenge et al. 2010) and with a higher incidence and prevalence of herpes simplex virus type 2 (Allsworth et al. 2009), increasing its impact on public health. Trichomoniasis is caused by the flagellated anaerobic protozoon Trichomonas vaginalis and has a global incidence of over 156 million cases per year (WHO 2018). The African and American continents have the highest prevalence rates (WHO 2018). In the USA, prevalence in women is about 2% (Flagg et al. 2019) while in Brazil, rates vary from 2.6 to 20% (Bruni et al. 2015; Ambrozio et al. 2016; Gatti et al. 2017).
Treatment for trichomoniasis is achieved at average to good rates when using 5-nitroimidazole drugs [i.e., metronidazole (MTZ) and tinidazole (TNZ)], the only Food and Drug Administration (FDA)-approved drugs for clinical use (Workowski and Bolan 2015; Menezes et al. 2016). However, there has been a constant rise in T. vaginalis isolates that are resistant to these drugs, currently representing 2.5 to 9.6% of clinical cases (Das et al. 2005; Schwebke and Barrientes 2006; Kirkcaldy et al. 2012). Moreover, allergies to 5-nitroimidazoles and recurrent infections have negatively influenced treatment regimens, consequently leading to increased treatment failure and adverse reactions (Cudmore et al. 2004; Bosserman et al. 2011; Seña et al. 2014).
This scenario has encouraged studies of drug discovery and development that aim to provide selective and effective new trichomonacidal drugs as treatment alternatives. In that sense, recent studies have described T. vaginalis enzymes as attractive chemotherapeutic targets for new drug candidates due to their impact on parasites’ survival (Setzer et al. 2017). Purine nucleoside phosphorylase (TvPNP) (Rinaldo-Matthis et al. 2007), lactate dehydrogenase (TvLDH) (Leitsch et al. 2010), triosephosphate isomerase (TvTIM) (Vique-Sánchez et al. 2020), and thioredoxin reductase (TvTrxR) (Hopper et al. 2016) are examples of enzymes being studied. TvTrxR, for instance, helps regulate levels of oxidative stress and defend parasites against oxidative damage and its inhibition could lead to unbalanced oxidative stress impairing parasites’ survival (Meyer et al. 2009). In addition, TvTrxR contributes to the mechanism of action of 5-nitroimidazole drugs, showing its importance as a drug target (Leitsch et al. 2009). Thus, molecules capable of selectively inhibiting these enzymes can be suitable drug candidates.
Quinolines containing trifluoromethyl substituents are found in a variety of bioactive compounds and exhibit an interesting pharmacological profile, since they have been described as antiviral (Briguglio et al. 2015; Barbosa-Lima et al. 2017), antimicrobial (Sumangala et al. 2010), and antimalarial (Hamann et al. 2014) drugs. Due to their interesting pharmacological profile, this study reports in vitro and in silico analyses of two 2,8-bis(trifluoromethyl) quinoline analogs against Trichomonas vaginalis.
Materials and methods
Chemistry
Synthesis of 2,8-bis(trifluoromethyl)-quinoline diamine derivatives (QDA) was conducted by Barbosa-Lima et al. (2017) and aliquots were kindly provided by Dr. Marcus Vinicius Nora de Souza from Instituto de Tecnologia em Fármacos—Far-Manguinhos, Fiocruz. Briefly, a mixture of 4-methoxy-trifluoromethylquinoline and diaminoalkane was gradually heated to 90–130 °C, with constant stirring, and maintained in this condition until complete consumption of the starting material was confirmed by thin-layer chromatography. Then, water was added, and the mixture was submitted to extraction with methyl acetate. Subsequently, the organic phase was dried and evaporated under reduced pressure to provide an oil that was purified through chromatographic purification on silica gel resulting in, among other derivatives, compounds 3a and 3d (Barbosa-Lima et al. 2017), from now on referred to as QDA-1 and QDA-2, respectively (Fig. 1).
Parasite and culture conditions
T. vaginalis isolate 30236 (from American Type Culture Collection—ATCC), sensitive to MTZ, was used in this study. Trophozoites were axenically cultured in trypticase-yeast extract-maltose (TYM) medium, without agar (pH 6.0), supplemented with 10% (v/v) heat-inactivated sterile bovine serum (SBS) and with 3% (v/v) of streptomycin (5 mg/mL) and incubated at 37 °C (Diamond 1957; Alves et al. 2020). Trophozoites’ motility, morphology, and viability were analyzed under light microscopy (× 400 magnification) through trypan blue (0.4%) exclusion assay. Subsequently, cultures exhibiting over 95% viability, logarithmic growth phase, and normal motility and morphology were harvested, centrifuged, and resuspended on fresh TYM medium for the antiparasitic and biochemical assays. All T. vaginalis assays described next were performed as at least three independent experiments in triplicate and results were expressed as the percentage of viable trophozoites in comparison with the negative control.
Anti-Trichomonas vaginalis assay
Two 2,8-bis(trifluoromethyl)-quinoline diamine derivatives (QDA-1 and QDA-2) were tested for in vitro activity against T. vaginalis following a method previously described (Sena-Lopes et al. 2017) with adaptations. Trophozoites were counted on a hemocytometer and adjusted to an initial density of 2.6 × 105 trophozoites/mL of TYM medium. All compounds were diluted in 100% dimethyl sulfoxide (DMSO). Then, 96-well plates were seeded with 150 µL of T. vaginalis trophozoites/well (2.6 × 105 trophozoites/mL), QDA-1 and QDA-2 were added at 100, 200, and 300 µM and plates were incubated at 37 °C with 5% CO2 for 24 h. After incubation, trophozoites were counted with trypan blue (0.4%) (1:1, v/v) on a hemocytometer to determine trophozoites’ motility, morphology, and viability. Three controls were used: a negative control containing only trophozoites in TYM medium, a positive control containing 100 µM MTZ (Sigma-Aldrich), and a 0.6% DMSO control (vehicle for solubilization). Only compounds that reduced the viability of parasites by 100% were used in the following experiments.
Minimum inhibitory concentration and 50% inhibitory concentration determination
Minimum inhibitory concentration (MIC) and 50% inhibitory concentration (IC50) against T. vaginalis were determined following the same experimental conditions described above, with compounds added to the 96-well plates (100 to 200 µM). MIC confirmation was performed by transferring trophozoites treated for 24 h with QDA-1 at MIC and a higher and lower concentration, as well as untreated trophozoites, to tubes containing 1.5 mL of fresh TYM medium, with 10% SBS and 3% streptomycin. Tubes were then re-incubated at 37 °C with 5% CO2 for 96 h and trophozoites were counted on a hemocytometer every 24 h to confirm MIC. The IC50 was calculated using GraphPad Prism 7.03 software.
Kinetic growth curve
To understand the antiparasitic activity of compounds against T. vaginalis cultures in a time-related manner, we established a kinetic growth curve. 96-well plates were seeded as described for the anti-T. vaginalis assay, compounds were added at respective MIC, and plates were incubated at 37 °C with 5% CO2 for 96 h. Trophozoites were observed under light microscopy and their growth and viability were evaluated at 1, 6, 12, 24, 48, 72, and 96 h by trypan blue (0.4%) dye exclusion and motility and morphology analysis.
Biochemical assays
DPPH radical scavenging assay
1,1-diphenyl-2-picryl-hydrazyl (DPPH) is a stable free radical frequently used as a substrate to evaluate in vitro antioxidant activity of compounds in a cell-free assay that is based on a single-electron transfer (SET) reaction and a hydrogen-atom removal. DPPH assay was carried out using a modified method (Choi et al. 2002). Briefly, QDA-1 (25–200 µM) was incubated in an ethanol solution containing 50 µM DPPH. The tubes were kept in the dark for 30 min and absorbance was measured at 517 nm. DPPH solution plus distilled water was used as a negative control and 100 µM Trolox (6-hydroxy-2,5,7,8-tetramethylchromane-2-carboxylic acid, Sigma Aldrich) was used as a positive control. Results were expressed as DPPH radical scavenging percentage (%) compared to the negative control.
Reactive oxygen species assay
The increased production and accumulation of reactive oxygen species (ROS) can also indicate oxidative stress. Therefore, ROS production by T. vaginalis after treatment with QDA was evaluated by measuring the oxidation of 2′-7′-dichlorofluorescein diacetate (DCF-DA) to dichlorofluorescein (DCF) as an indication of intracellular ROS (adapted from Cheng et al. 2015; Casaril et al. 2020). Trophozoites (5 × 105 trophozoites/mL) were incubated with compound at MIC and IC50 for 24 h at 37 °C, 5% CO2. The negative control consisted of untreated trophozoites and the positive control comprised trophozoites treated with hydrogen peroxide (H2O2, 5 mM). After incubation, 10 μM of DCF-DA was added to each well for 1 h at 37 °C. Then, samples were transferred to a 10 mM Tris–HCl pH 7.4 buffer and DCF fluorescence was immediately detected using a fluorescence spectrophotometer (excitation/emission 480/520 nm). ROS levels were expressed as arbitrary fluorescence units.
Thiobarbituric acid reactive substances assay
Lipid peroxidation is an indicator of oxidative stress that can be estimated by measuring the formation of malondialdehyde (MDA), a biomarker for lipid peroxidation, through the thiobarbituric acid reactive substances (TBARS) assay. Hence, we evaluated lipid peroxidation in T. vaginalis after treatment with QDA-1 and controls following a method previously described (Ohkawa et al. 1979) with modifications. Briefly, a 96-well plate was seeded with 150 µL of T. vaginalis (2.6 × 105 trophozoites/mL), compound at MIC and controls were added, and plates were incubated at 37 °C with 5% CO2 for 24 h. After that, T. vaginalis controls and treatment groups were incubated with 500 µL of 0.8% TBA, 500 µL of acetic acid/HCl (pH 3.4), and 200 µL of 8.1% SDS at 95 °C for 2 h. Samples’ absorbance was measured at 532 nm and results were expressed as nmol MDA/105 trophozoites.
Nitric oxide metabolites detection assay
Nitric oxide (NO) is a highly unstable reactive species that can be easily oxidized into its metabolites nitrate and nitrite. Therefore, to investigate if treatment with QDA-1 could lead to NO production and/or accumulation by T. vaginalis, NO metabolites (NOx) levels were detected through Griess’ reaction using an adapted method (Cheng et al. 2015; Birmann et al. 2020). First, parasites (5 × 105 trophozoites/mL) were seeded in a 96-well plate and treated with compound at MIC and IC50 for 24 h at 37 °C, 5% CO2. A negative control of untreated trophozoites was used. After that, an equal volume of Griess reagent was added to the wells for 5 min at 25 °C, and the NOx concentration was determined by measuring the optical density at 492 nm and plotted against a standard curve of NaNO2. Results were expressed as nmol NOx/105 trophozoites.
Cell culture
African green monkey kidney (Vero) cells were obtained from the Rio de Janeiro Cell Bank (PABCAM, Federal University of Rio de Janeiro, RJ, Brazil) and cultured in RPMI 1640 (Vitrocell Embriolife, Campinas, Brasil), supplemented with 10% fetal bovine serum (FBS) (Vitrocell Embriolife, Campinas, Brasil), 1% L-glutamine, and 1% penicillin/streptomycin at 37 °C, 95% humidified air, and 5% CO2.
Cytotoxicity assay
To evaluate how QDA-1 affects mammalian cells and better understand its selectivity, we performed a cytotoxicity assay following a method previously described (Alves et al. 2020). Monolayers of 2 × 104 VERO cells were cultured in 96-well culture plates and grown for 24 h at 37 °C, 5% CO2. After incubation, 100 µL/well of compound at 12.5, 25, 50, 100, and 200 µM was added and plates were re-incubated for another 24 h. Besides the negative control (untreated cells), 0.6% DMSO and MTZ at 100 μM were added as controls. After that, 100 μL/well of 5 mg/mL 3-(4,5-dimethylthiazol-2-yl)-2,5-diphenyltetrazolium bromide (MTT) (Sigma-Aldrich) was added and plates were re-incubated for 3 h at 37 °C, 5% CO2. Finally, 100 μL of DMSO was added per well to solubilize formazan crystals. Plates were analyzed on a microplate reader at 492 nm. Percent proliferation inhibition was determined according to the formulae:
Half maximal cytotoxic concentration (CC50) was calculated by analyzing the relationship between concentrations and inhibitions rates (%) using GraphPad Prism 7.03 Software (La Jolla, CA, USA). The selectivity index (SI) was calculated as the ratio between the CC50 measured on mammalian cells over the IC50 determined on T. vaginalis trophozoites (SI = CC50/IC50). The assay was performed at least three independent times in triplicate.
Molecular docking and in silico drug-likeness
We next turned our attention to further assessing the possible mechanism of action of QDA-1 by using a molecular docking approach to predict the interactions of QDA-1 with four proteins important for T. vaginalis survival. For this inquiry, we used the following protein structures of T. vaginalis: purine nucleoside phosphorylase (TvPNP; PDB 1Z36; TVAG_127180), lactate dehydrogenase (TvLDH; PDB 5A1T; TVAG_495880), and triosephosphate isomerase (TvTIM; PDB 3QST; TVAG_096350), which were retrieved from RSCB protein data bank (http://www.rcsb.org/). The structure of T. vaginalis thioredoxin reductase (TvTrxR) was constructed using the server SWISS-MODEL (Waterhouse et al. 2018) based on the Uniprot entry A2DSU2 (TVAG_348010). Before docking, protein structures were prepared by removing water molecules and other ligands using CHIMERA 1.5.3 (Pettersen et al. 2004) software. A grid box size covering the residues in the active site of the proteins was implemented. Docking to the active site was preferred over blind docking since defining the binding site directly affects possible binding modes and binding affinity, which in consequence reflects on the result of the prediction (Stanzione et al. 2021). QDA-1 was built and optimized in the software Avogadro 1.1.1. (Hanwell et al. 2012). The lowest binding energy of each molecule conformer was calculated using the default parameters of Autodock Vina 1.1.2. (Trott and Olson 2010) and the docking poses of the investigated compound were visualized using Accelrys Discovery Studio 3.5.
In addition, the drug-likeness of QDA-1 was assessed using the criteria established by Lipinski and known as Lipinski’s rule of five. Such criteria evaluate the theoretical oral bioavailability of the compound by considering four physicochemical parameters (partition coefficient (miLogP) ≤ 5.0, molecular weight ≤ 500, hydrogen bond acceptors ≤ 10, and donors ≤ 5) that are associated with orally active drugs. Compounds that violate one or more rules are said to show an unattractive oral bioavailability (Lipinski 2004).
Statistical analysis
Statistical analysis was carried out by one-way analysis of variance (ANOVA), followed by Tukey’s post-test, for all assays except the kinetic growth curve, which was analyzed by two-way ANOVA, followed by Tukey’s post-test. All tests were performed using GraphPad Prism 7.03 Software with p < 0.05. Data were expressed as mean ± standard deviation (SD).
Results
Anti-Trichomonas vaginalis assay
The antiparasitic assay against T. vaginalis was performed using three concentrations for each compound (Fig. 2A and B) and the results demonstrated the trichomonacidal activity of QDA-1 at 200 µM and 300 µM, with complete elimination of parasites (Fig. 2A). On the other hand, QDA-2 reduced trophozoites’ growth by 26.8%, 48.3%, and 76.9% at 100 µM, 200 µM, and 300 µM, respectively, but exposure to this compound was not enough to be comparable with MTZ nor to eliminate trophozoites (Fig. 2B). As expected, the negative and 0.6% DMSO controls did not affect trophozoites’ viability (no staining with trypan blue), motility, and morphology, while MTZ exposure led to a positive trypan blue staining and no motility, indicating a complete decrease in viability. Considering such results, only QDA-1 was chosen to proceed with the remaining tests.
In vitro antiparasitic activity of QDA-1 (A) and QDA-2 (B) at 100, 200, and 300 µM against Trichomonas vaginalis ATCC 30236. Results were confirmed by the trypan blue (0.4%) exclusion assay after 24 h of exposure. Control (untreated trophozoites), DMSO (vehicle for solubilization at 0.6%), MTZ (metronidazole at 100 µM). Viability of 100% in control corresponds to an initial density of 2.6 × 105 trophozoites/mL. Data are represented as mean ± SD of at least three independent experiments. Different letters (a–e) show a significant difference with p < 0.05
MIC and IC50
A more detailed analysis of QDA-1, using a wider range of concentrations, allowed the determination of MIC and IC50 for this compound’s antiparasitic activity against T. vaginalis. QDA-1 reduced trophozoites viability by 31.7%, 54.4%, 84.5%, 91.2%, 94.9%, and 100% at 100 µM, 120 µM, 140 µM, 160 µM, 180 µM, and 200 µM, respectively (Fig. 3A). In view of this, IC50 was determined as being 113.8 µM and MIC was determined as being 160 µM (Fig. 3A) because this is the lowest concentration that completely inhibited trophozoites in 24 h, which was confirmed by the absence of parasite growth in samples transferred to fresh TYM medium, and showed no significant difference from MTZ.
(A) MIC and IC50 of QDA-1 against Trichomonas vaginalis ATCC 30236 after exposure to 100, 120, 140, 160, 180, and 200 µM concentrations for 24 h. (B) Kinetic growth curve of Trichomonas vaginalis ATCC 30236 during (0 h to 24 h) and after (24 h to 96 h) treatment with QDA-1 at 160 µM. Growth was completely inhibited at 24 h. Control (untreated trophozoites), DMSO (vehicle for solubilization at 0.6%), MTZ (metronidazole at 100 µM). Viability of 100% in control corresponds to a minimum of 2.6 × 105 trophozoites/mL. Data are represented as mean ± SD of at least three independent experiments. Different letters (a–e) show a significant difference with p < 0.05 (A). (****) p < 0.0001 when compared with the control group and (#) p < 0.05 and (####) p < 0.0001 when compared with MTZ group (B)
Kinetic growth curve
Data obtained from this assay demonstrated that QDA-1 at MIC reduced 17.9% of trophozoites viability in the first hour and exhibited a significant difference between control and MTZ groups. However, reduction rates were not comparable to MTZ at 6 h and 12 h, with QDA-1 reducing 21.3% and 34%, and MTZ decreasing 55.2% and 100% of growth, respectively (Fig. 3B). Although there seems to have been a residual 8.8% viability at 24 h, it can be said that QDA-1 (at 160 µM) completely reduced trophozoites’ growth at 24 h because there was no growth during the MIC confirmation assay. In other words, the trophozoites were damaged beyond repair after 24 h, but not all of them had died at that time point.
Biochemical assays
Considering the significant impact oxidative stress can have on T. vaginalis trophozoites’ survival, a series of biochemical assays were performed to shed light on whether QDA-1 affects redox pathways and oxidative stress regulation.
DPPH radical scavenging assay
To determine whether QDA-1 had any intrinsic antioxidant activity, a DPPH reduction assay was performed. Data revealed only one concentration of QDA-1 (50 µM) had a minor scavenging ability (16.6%) when compared to the negative control, indicating a slight antioxidant activity (Fig. 4A). However, such activity is significantly smaller than the one observed for the positive control, demonstrating that overall QDA-1 did not have an antioxidant profile according to this method. In addition, IC50 values (sample concentration required to inhibit 50% of the radicals) could not be calculated since QDA-1 concentrations inhibited less than 20% of DPPH radicals.
QDA-1 radical scavenging ability and effect on oxidative stress markers. (A) DPPH radical scavenging activity of QDA-1. (B) Lipid peroxidation, (C) ROS, and (D) NOx levels measured after 24 h of exposure to QDA-1 at IC50 and/or MIC. Data are represented as mean ± SD of at least three independent experiments. (####) p < 0.0001 when compared with positive control Trolox (A); (#) p < 0.05 and (###) p < 0.001 when compared with QDA-1 group (B); (*) p < 0.05, (**) p < 0.01, (****) p < 0.0001 when compared with the control group (A-D). MIC = 160 µM; IC50 = 113.8 µM
ROS assay
ROS are highly reactive and oxidant agents naturally produced (and efficiently eliminated) by cells’ metabolic pathways. However, ROS overproduction and/or accumulation may cause oxidative stress and consequent cell damage that could lead to cell death (Grotto et al. 2009; Katerji et al. 2019). Considering the role of ROS in oxidative stress and its possible consequences on parasites’ survival, we investigated whether treatment with QDA-1 could stimulate ROS production by T. vaginalis. QDA-1 at MIC significantly induced ROS production by T. vaginalis trophozoites when compared to control and ROS levels were equivalent to those generated by the positive control (H2O2) (Fig. 4C).
TBARS assay
Lipid peroxidation is a chain of reactions that leads to the deterioration of polyunsaturated fatty acids and, as a result, oxidative damage of cell membranes; as such, it has been used as a biomarker for oxidative stress (Grotto et al. 2009; Ayala et al. 2014). Considering how vital membrane integrity is for survival, we analyzed whether lipid peroxidation levels were altered after treatment with QDA-1. Results showed that QDA-1 caused a significant (p < 0.01) increase in lipid peroxidation, measured through MDA formation, when compared with the control (untreated trophozoites). Additionally, MDA levels for QDA-1 treatment were also significantly higher than for MTZ and 0.6% DMSO (Fig. 4B). Unsurprisingly, exposure to 0.6% DMSO did not alter MDA levels when compared to the control. The same happened for MTZ exposure (Fig. 4B).
NO metabolites detection assay
Considering that NO accumulation causes cellular damage, immune effector cells, such as macrophages and neutrophils, normally upregulate the production of NO and other reactive nitrogen species (RNS) as a defense mechanism. Then, NO and RNS act as cytotoxic effectors against microorganisms and parasites, such as T. vaginalis (Brunet 2001; Mallo et al. 2020). However, trophozoites also produce NO as part of their metabolism and use alternative pathways than those known for mammalian cells (Cheng et al. 2015, 2020).
In this sense, we analyzed whether QDA-1 treatment could induce NO accumulation in T. vaginalis. Results revealed that there was no accumulation of NO in trophozoites treated with QDA-1 at IC50 and MIC (Fig. 4D). Interestingly, there was a slight, but statistically significant (p < 0.05 for QDA-1 IC50 and p < 0.0001 for QDA-1 MIC), reduction in the concentration of NOx in treated groups when compared to the control (Fig. 4D). This reduction could depend on concentration, since treated groups were also statistically different from each other (p < 0.0001).
Cytotoxicity assay
The cytotoxic activity of QDA-1 was evaluated against the Vero cell line and data obtained from the MTT assay demonstrated that QDA-1 inhibited 36.7% of cell growth at 200 µM, 12.6% at 100 µM, and 8.5% at 50 µM. Concentrations equal to or lower than 50 µM were statistically comparable to MTZ. The CC50 was established as 247.4 µM ± 1.1 µM (standard error), which is higher than the MIC (160 µM), and all the concentrations that caused some sort of cytotoxicity affected less than 50% of the cells (Fig. 5). These results allowed the calculation of our compound’s SI (CC50/IC50), which was defined as being 2.2. As expected, 0.6% DMSO did not alter cell growth.
Cytotoxicity of QDA-1 at 12.5, 25, 50, 100, and 200 µM against Vero cells investigated by MTT assay after 24 h of exposure. DMSO (vehicle for solubilization at 0.6%), MTZ (metronidazole at 100 µM). Data are represented as mean ± standard error means of at least three independent experiments. Different letters (a–c) show a significant difference between treatments with p < 0.05
Molecular docking and in silico drug-likeness
In order to explore the possible mechanism of action of QDA-1 against T. vaginalis, a molecular docking experiment was carried out. From an overall determination of root-mean-square deviations (RMSD) and estimated binding free energy (ΔGbind), the best docking pose for QDA-1 with each protein was selected. Two-dimensional and three-dimensional binding modes are represented in Figs. 6 and 7 and the binding mode of QDA-1 into the active site of the enzymes is demonstrated in Fig. 8.
Putative binding mode of QDA-1 into the active site of TvPNP (A), TvLDH (B), TvTrxR (C), and TvTIM (D). Dashed lines represent interactions between QDA-1 and the specific residues. Green lines represent hydrogen bonds and cyan lines represent halogen bonds. All other colors represent hydrophobic interactions (following the same representation as in Figs. 6 and 7)
As depicted in Fig. 6 A, QDA-1 interacts with the active site of TvPNP with a ΔGbind of − 8.4 kcal/mol (RMSD of 2.51 Å). Interestingly, PHE159, VAL178, and ILE206, which are residues present in the purine binding site of TvPNP (Fig. 8A), are involved in halogen bond and hydrophobic interaction with QDA-1 in silico. In addition, the best binding mode of QDA-1 with TvLDH is represented in Fig. 6 B, with a docking score of − 7.4 kcal/mol and an RMSD of 2.67 Å. In silico, QDA-1 makes one hydrogen and two halogen bonds with ASN130 and one hydrogen bond with THR239, which are components of the active site of TvLDH involved in the binding of NADH and oxamate (a TvLDH inhibitor) (Fig. 8B). Additionally, one hydrogen bond is formed with LEU91, also present in the active site of the enzyme (Fig. 8B).
The binding mode of QDA-1 with TvTrxR is depicted in Fig. 7 A. Visual inspection of the pose of QDA-1 in the TvTrxR binding site displayed favorable in silico binding affinity (ΔGbind of − 7.2 kcal/mol and RMSD of 1.43 Å) to the enzyme via a variety of interactions, including a hydrophobic interaction with VAL190 and van der Waals forces with GLY191 and GLY192, which are a part of the enzyme active site (Fig. 8C). In addition, QDA-1 formed three hydrogen bonds with ARG215 and ILE276 (Figs. 7A and 8C). Molecular docking studies also predicted that QDA-1 binds to the active site of TvTIM (Fig. 7B) with a docking score of − 6.4 kcal/mol and RMSD of 2.61 Å. QDA-1 interacted through van der Waals forces with the catalytic residues LYS11 and HIS94, the latter of which is found in the active site of the enzyme (Fig. 8D).
Furthermore, QDA-1 presented in silico interaction with several other residues of TvPNP, TvLDH, TvTrxR, and TvTIM, besides the abovementioned ones, through a variety of chemical bonds that are described in more detail in Table 1. Finally, drug-likeness in silico analysis demonstrated that QDA-1 does not break any of Lipinski’s rule-of-five.
Discussion
Trichomoniasis treatment has been performed for at least five decades with the 5-nitroimidazoles (MTZ and TNZ) following FDA recommendations (Meites et al. 2015; FDA 2021). However, MTZ-resistant isolates of T. vaginalis and allergic reactions (Kirkcaldy et al. 2012; Workowski and Bolan 2015), among other things, have been impairing treatment and leading to failures. These problems reinforce the need for new compounds with trichomonacidal potential. Herein, we analyzed the in vitro and in silico potential of two 2,8-bis(trifluoromethyl) quinoline analogs against T. vaginalis (Figs. 2 and 3), from which we identified one, QDA-1, with a significant activity (Fig. 2A).
Antiparasitic assays showed that QDA-1 was able to inhibit trophozoites with a MIC of 160 µM and an IC50 of 113.8 µM after 24 h of exposure (Fig. 3A). Additionally, kinetic growth analysis revealed that the compound had a different impact on trophozoites growth when compared to MTZ, taking a few hours longer to kill the trophozoites but leading to no viability (Fig. 3B).
Compounds containing 2,8-bis-trifluoromethyl-quinoline-4-yl moiety have been previously tested against four bacterial strains (Escherichia coli, Staphylococcus aureus, Pseudomonas aeruginosa, and Klebsiella pneumoniae) and were found to have significant activity against at least one of them at 3.125 and 6.25 µM (Sumangala et al. 2010). Another study showed the potential of 2,8-bis(trifluoromethyl) quinoline analogs against Plasmodium falciparum, with all compounds displaying a significant activity in concentrations ranging from 1 to 31.8 µM (Hamann et al. 2014). These studies corroborate with 2,8-bis(trifluoromethyl) quinolines being biologically active molecules with antiprotozoal potential, as showed in our study.
In a more recent study, QDA-1 and QDA-2 were tested against the ZIKA virus and QDA-1 inhibited 78.6% ± 4.3% of the virus replication at 10 µM (Barbosa-Lima et al. 2017). The same study tested this compound’s cytotoxicity in Vero cells and reported a CC50 of 195 µM, which is similar to the CC50 (247.4 µM) observed here, also for Vero cells. Therefore, our results corroborated previous findings and showed that this compound’s cytotoxicity would not, thus far, impair its investigation and possible application as a trichomonacidal agent. In addition, these results demonstrated the chemotherapeutical potential of 2,8-bis(trifluoromethyl) quinoline analogs corroborating the results found in our study.
In addition, we calculated the SI, a parameter set to characterize the relative effectiveness of a compound in inhibiting parasite proliferation and/or inducing parasite death as compared to inducing mammalian cell death. Although authors have described a SI > 1 as indicative of selectivity toward the parasite (Tiuman et al. 2005; Sosa et al. 2020), others defend that a SI > 10 is an indication that the primary antiprotozoal activity is not due to the intrinsic cytotoxicity of a compound (Colín-Lozano et al. 2017; Rocha-Garduño et al. 2020). The SI found for QDA-1 anti-T. vaginalis activity was 2.2, which, considering the aforementioned, can represent a level of selectivity toward T. vaginalis. However, such a level is still lower than it would be desired, because higher SIs represent maximal antiparasitic activity with the lowest cellular toxicity. Nevertheless, the concentration needed to kill T. vaginalis trophozoites is lower than the CC50 found in this study and resulted in a moderate SI. Therefore, the trichomonacidal activity presented by QDA-1 cannot be denied or dismissed.
Structurally, QDA-1 and QDA-2 have quite a lot in common and Fig. 1 shows that the differences rely on the presence of distinct types of amine groups. QDA-1 has a radical constituted by a primary (NH2) and a secondary (NH) amine group connected by a carbon chain, while QDA-2 only has a secondary amine group followed by a carbon chain in the same position. The NH2 group is highly reactive, binding to other molecules and allowing several chemical reactions, such as the formation of hydrogen bonds. Since this radical is only present in QDA-1 and considering such reactivity, this functional group might be important to the interactions between the compound and the parasite and at some level influence its activity. Corroborating that, the primary amine group in QDA-1 seems to be involved in a hydrogen bond with ILE276 in TvTrxR, as shown by molecular docking. This interaction may account for a possible modulation of this antioxidant enzyme.
As one of the most widely used computational approaches for the rational selection of compounds, a molecular docking study was used to predict the binding pose of QDA-1 with binding sites of four T. vaginalis proteins. T. vaginalis and other parasitic protozoa lack de novo synthesis of purine nucleosides and, therefore, rely on the functions of nucleoside phosphorylase (PNP) and nucleoside kinase (Rinaldo-Matthis et al. 2007). This metabolic deficiency makes the purine salvage pathway a target for antiparasitic chemotherapy. Overall, the best docking pose of QDA-1 with TvPNP involved interactions with several residues within the purine-binding site (active site), suggesting that this binding mode could account for the significant docking score between these molecules and that TvPNP might be modulated by QDA-1.
It has been shown that a loss of activity of hydrogenosomal enzymes is involved in the resistance acquired by some strains of T. vaginalis, making them dependent on glycolytically generated lactate for energy metabolism (Harmych et al. 1996; Leitsch et al. 2009, 2010). In light of this, inhibition of the enzyme lactate dehydrogenase (LDH), which catalyzes the interconversion of lactate to pyruvate, appears as a rational approach to the development of new molecules with anti-T. vaginalis activity. QDA-1 demonstrated strong interactions with three residues in the active site of TvLDH, including residues involved in interactions with well-known inhibitors of this enzyme. These interactions could be relevant for the modulation of TvLDH by QDA-1, impairing the energy metabolism and reducing the survival of T. vaginalis.
The oxygen metabolism and redox signaling in T. vaginalis may yield a pro-oxidative environment, and since this species lacks glutathione and catalase, the protection against oxidative damage in T. vaginalis depends, at least partially, on the flavin enzyme thioredoxin reductase (TrxR) (Meyer et al. 2009). Considering that TvTrxR is a rational target for trichomoniasis chemotherapy (Leitsch et al. 2009) and in silico interactions between QDA-1 and TvTrxR involved interactions with three residues that are a part of the enzyme active site, modulation of this enzyme might be related to the mechanism of action of QDA-1. In line with that, results from the TBARS assay revealed that QDA-1 induced lipid peroxidation, and since this process can lead to oxidative deterioration of lipids and membrane damage (Grotto et al. 2009), authors suggest that this may be one of the mechanisms involved in parasites’ death. Together, these findings suggest that the increased MDA levels found after exposure to QDA-1 could be, at least in part, due to the impairment of the antioxidant system in T. vaginalis, more specifically, the inhibition of TvTrxR.
The increased production of ROS in trophozoites treated with QDA-1 at MIC also points to an unbalanced oxidative stress cell environment and corroborates the hypothesis that the compound is impairing antioxidant defenses. In addition, the lack of DPPH radical scavenging ability for this compound at MIC indicated the absence of intrinsic antioxidant activity, suggesting that QDA-1 did not positively affect the T. vaginalis redox system. Interestingly, QDA-1 did not lead to the direct accumulation of NO in trophozoites, indicating this was not a mechanism involved in T. vaginalis death.
Recently, the enzyme triosephosphate isomerase (TIM) has been acknowledged as an additional “moonlighting” metabolic enzyme in T. vaginalis (Miranda-Ozuna et al. 2016). This means that TvTIM has more than one function depending on its cellular localization or its concentration and therefore, arises as a promising target for antiparasitic chemotherapy. TvTIM mainly functions as a cytoplasmic enzyme involved in glycolysis, gluconeogenesis, and the pentose phosphate pathway (Wierenga et al. 2010). Here, we reported that QDA-1 interacted in silico with TvTIM through residues previously described as catalytic (Figueroa-Angulo et al. 2012) and residues in the active site of the enzyme. Hence, such in silico interactions could indicate a possible mechanism of action for the in vitro anti-T. vaginalis activity described here.
The trifluoromethyl-substituent–containing quinoline nucleus is a structural constituent of several bioactive compounds that have been tested as therapeutic agents in a wide range of diseases, such as malaria (Hamann et al. 2014), leukemia (Shin et al. 2014), and viral infections (Barbosa-Lima et al. 2017). Keeping this in view, our study aimed to investigate the potential anti-T. vaginalis activity of quinoline derivatives. Particularly, the binding mode of QDA-1 with the enzymes evaluated here may shed light on the mechanism of action of this molecule against T. vaginalis, and it could be translated to other parasitic protozoa that share the activity of these enzymes for their survival. Therefore, we suggest that the in vitro activity of QDA-1 might be related to the modulation of TvPNP, TvLDH, TvTrxR, and TvTIM enzymatic activities.
Nevertheless, further studies must be conducted to address this hypothesis and elucidate the mechanism of action of QDA-1. Hopper et al. (2016), for instance, analyzed how auranofin interacts with TvTrxR and how that at least partially induces parasites death, and Vique-Sánchez et al. (2020) used virtual screening and molecular docking to find three compounds that can selectively inhibit TvTIM. These studies present excellent strategies that can be applied to further investigating the effect QDA-1 has on T. vaginalis.
Drug-likeness in silico analysis showed QDA-1 did not violate any of Lipinski’s rule-of-five and could, therefore, have favorable properties to be administered orally in humans and to be used as a candidate in the development of a novel antiparasitic agent (Lipinski 2004).
Finally, data from this study revealed that QDA-1 had a significant antiparasitic activity against T. vaginalis and is a promising molecule for studies on new trichomonacidal agents. Although QDA-1 had a slightly cytotoxic effect on Vero cells, the MIC for anti-T. vaginalis activity is still within a safe limit when compared to the CC50 in mammalian cells. Besides that, some techniques can be explored to enhance the delivery and absorption of this compound, potentially increasing its selectivity and antiparasitic activity.
In this sense, an approach for future studies may be the nanoencapsulation of this molecule, allowing its controlled release and improving uptake by trophozoites (Mora-Huertas et al. 2010). This method may help to diminish the cytotoxicity observed in our study, as it has been previously shown for other molecules (Sattar et al. 2017; Esfandiari et al. 2019). Nanoencapsulation also allows the targeting of specific tissues, and it can sometimes induce increased efficacy and bioavailability (Mora-Huertas et al. 2010; Frank et al. 2015), which can consequently allow the use of lower concentrations, reducing cytotoxic effects. On another note, a recent study showed that compounds applied as self-nanoemulsifying drug delivery systems had improved selectivity indexes and efficacy when compared to free compounds (Bezerra-Souza et al. 2019). Thus, nanoemulsification techniques can be another alternative for future studies with QDA-1.
Conclusions
This study showed that QDA-1 had significant antiparasitic activity against Trichomonas vaginalis and, although a slight cytotoxicity was found on mammalian cells, the antiparasitic potential for this compound is still noteworthy and should be studied in more detail. Molecular docking studies have revealed that QDA-1 interacted with the active site of TvPNP, TvLDH, TvTrxR, and TvTIM, suggesting pathways that might be involved in the in vitro activity against T. vaginalis. Nonetheless, further studies are required to confirm the trichomonacidal mechanism of action of QDA-1.
Data availability
The datasets generated during and/or analyzed during the current study are available from the corresponding author on reasonable request.
References
Allsworth JE, Ratner JA, Peipert JF (2009) Trichomoniasis and other sexually transmitted infections: results from the 2001–2004 national health and nutrition examination surveys. Sex Transm Dis 36:738–744. https://doi.org/10.1097/OLQ.0b013e3181b38a4b
Alves MSD, dasNeves RN, Sena-Lopes A et al (2020) Antiparasitic activity of furanyl N-acylhydrazone derivatives against Trichomonas vaginalis: in vitro and in silico analyses. Parasit Vectors 13:59. https://doi.org/10.1186/s13071-020-3923-8
Ambrozio CL, Nagel AS, Jesk S et al (2016) Trichomonas vaginalis prevalence and risk factors for women in southern Brazil. Rev Inst Med Trop Sao Paulo 58:1–5. https://doi.org/10.1590/S1678-9946201658061
Ayala A, Muñoz MF, Argüelles S (2014) Lipid peroxidation: production, metabolism, and signaling mechanisms of malondialdehyde and 4-hydroxy-2-nonenal. Oxid Med Cell Longev 2014:1–31
Barbosa-Lima G, Moraes AM, A da Araújo S et al (2017) 2,8-bis(trifluoromethyl)quinoline analogs show improved anti-Zika virus activity, compared to mefloquine. Eur J Med Chem 127:334–340. https://doi.org/10.1016/j.ejmech.2016.12.058
Bezerra-Souza A, Fernandez-Garcia R, Rodrigues GF et al (2019) Repurposing butenafine as an oral nanomedicine for visceral leishmaniasis. Pharmaceutics 11:353. https://doi.org/10.3390/pharmaceutics11070353
Birmann PT, Casaril AM, Hartwig D et al (2020) A novel pyrazole-containing selenium compound modulates the oxidative and nitrergic pathways to reverse the depression-pain syndrome in mice. Brain Res 1741:146880. https://doi.org/10.1016/j.brainres.2020.146880
Bosserman EA, Helms DJ, Mosure DJ et al (2011) Utility of antimicrobial susceptibility testing in Trichomonas vaginalis-infected women with clinical treatment failure. Sex Transm Dis 38:983–987. https://doi.org/10.1097/OLQ.0b013e318224db39
Briguglio I, Loddo R, Laurini E et al (2015) Synthesis, cytotoxicity and antiviral evaluation of new series of imidazo[4,5-g]quinoline and pyrido[2,3-g]quinoxalinone derivatives. Eur J Med Chem 105:63–79. https://doi.org/10.1016/J.EJMECH.2015.10.002
Brunet LR (2001) Nitric oxide in parasitic infections. Int Immunopharmacol 1:1457–1467. https://doi.org/10.1016/S1567-5769(01)00090-X
Bruni MP, Lopes ÂS, Stauffert D et al (2015) Trichomonas vaginalis infection among women attending frequency, risk factors and clinical signs. DST - J Bras De Doenças Sexualmente Transmissíveis 27:86–91. https://doi.org/10.5533/DST-2177-8264-2015273404
Casaril AM, Domingues M, Lourenço DDA et al (2020) 3-[(4-chlorophenyl)selanyl]-1-methyl-1H-indole ameliorates long-lasting depression- and anxiogenic-like behaviors and cognitive impairment in post-septic mice: Involvement of neuroimmune and oxidative hallmarks. Chem Biol Interact 331:109278. https://doi.org/10.1016/j.cbi.2020.109278
Cheng WH, Huang KY, Huang PJ et al (2015) Nitric oxide maintains cell survival of Trichomonas vaginalis upon iron depletion. Parasit Vectors 8:393. https://doi.org/10.1186/s13071-015-1000-5
Cheng WH, Huang KY, Ong SC et al (2020) Protein cysteine S-nitrosylation provides reducing power by enhancing lactate dehydrogenase activity in Trichomonas vaginalis under iron deficiency. Parasit Vectors 13:477. https://doi.org/10.1186/s13071-020-04355-0
Choi CW, Kim SC, Hwang SS et al (2002) Antioxidant activity and free radical scavenging capacity between Korean medicinal plants and flavonoids by assay-guided comparison. Plant Sci 163:1161–1168
Colín-Lozano B, León-Rivera I, Chan-Bacab MJ et al (2017) Synthesis, in vitro and in vivo giardicidal activity of nitrothiazole-NSAID chimeras displaying broad antiprotozoal spectrum. Bioorg Med Chem Lett 27:3490–3494. https://doi.org/10.1016/j.bmcl.2017.05.071
Cudmore SL, Delgaty KL, Shannon F et al (2004) Treatment of infections caused by metronidazole-resistant Trichomonas vaginalis. Clin Microbiol Rev 17:783–793. https://doi.org/10.1128/CMR.17.4.783
Das S, Huengsberg M, Shahmanesh M (2005) Treatment failure of vaginal trichomoniasis in clinical practice. Int J STD AIDS 16:284–286. https://doi.org/10.1258/0956462053654258
Diamond LS (1957) The establishment of various trichomonads of animals and man in axenic cultures. J Parasitol 43:488–490
dosGatti FAA, Ceolan E, Greco FSR et al (2017) The prevalence of trichomoniasis and associated factors among women treated at a university hospital in southern Brazil. PLoS ONE 12:e0173604. https://doi.org/10.1371/journal.pone.0173604
Esfandiari F, Motazedian MH, Asgari Q et al (2019) Erratum: Paromomycin-loaded mannosylated chitosan nanoparticles: synthesis, characterization and targeted drug delivery against leishmaniasis. Acta Trop 197:105072. https://doi.org/10.1016/j.actatropica.2019.105072
Figueroa-Angulo EE, Estrella-Hernández P, Salgado-Lugo H et al (2012) Cellular and biochemical characterization of two closely related triosephosphate isomerases from Trichomonas vaginalis. Parasitology 139:1729–1738. https://doi.org/10.1017/S003118201200114X
Flagg EW, Meites E, Phillips C et al (2019) Prevalence of Trichomonas vaginalis among civilian, noninstitutionalized male and female population aged 14 to 59 years: United States, 2013 to 2016. Sex Transm Dis 46:e93. https://doi.org/10.1097/OLQ.0000000000001013
Frank LA, Contri RV, Beck RCR et al (2015) Improving drug biological effects by encapsulation into polymeric nanocapsules. Wiley Interdiscip Rev Nanomedicine Nanobiotechnology 7:623–639. https://doi.org/10.1002/wnan.1334
FDA (2021) FDA approved drug products. In: Food and Drug Administration. https://www.accessdata.fda.gov/scripts/cder/daf/. Accessed 10 Mar 2022
Grotto D, Maria LS, Valentini J et al (2009) Importance of the lipid peroxidation biomarkers and methodological aspects for malondialdehyde quantification. Quim Nova 32:169–174. https://doi.org/10.1590/S0100-40422009000100032
Hamann AR, de Kock C, Smith PJ et al (2014) Synthesis of novel triazole-linked mefloquine derivatives: biological evaluation against Plasmodium falciparum. Bioorg Med Chem Lett 24:5466–5469. https://doi.org/10.1016/j.bmcl.2014.10.015
Hanwell MD, Curtis DE, Lonie DC et al (2012) Avogadro: an advanced semantic chemical editor, visualization, and analysis platform. J Cheminformatics 4:17. https://doi.org/10.1186/1758-2946-4-17
Harmych SE, Sidawy E, Komuniecki R (1996) Lactate dehydrogenase from the protozoan parasite, Trichomonas vaginalis. Comp Biochem Physiol b: Biochem Mol Biol 115:405–409. https://doi.org/10.1016/S0305-0491(96)00164-2
Hopper M, Yunfil J, Zhou B et al (2016) Auranofin inactivates Trichomonas vaginalis thioredoxin reductase and is effective against trichomonads in vitro and in vivo. Int J Antimicrob Agents 48:690–694. https://doi.org/10.1016/j.ijantimicag.2016.09.020
Katerji M, Filippova M, Duerksen-Hughes P (2019) Approaches and methods to measure oxidative stress in clinical samples: research applications in the cancer field. Oxid Med Cell Longev 2019:1–29
Kirkcaldy RD, Augostini P, Asbel LE et al (2012) Trichomonas vaginalis antimicrobial drug resistance in 6 US cities, STD surveillance network, 2009–2010. Emerg Infect Dis 18:939–943. https://doi.org/10.3201/eid1806.111590
Leitsch D, Kolarich D, Binder M et al (2009) Trichomonas vaginalis: metronidazole and other nitroimidazole drugs are reduced by the flavin enzyme thioredoxin reductase and disrupt the cellular redox system. Implications for nitroimidazole toxicity and resistance. Mol Microbiol 72:518–536. https://doi.org/10.1111/j.1365-2958.2009.06675.x
Leitsch D, Kolarich D, Duchêne M (2010) The flavin inhibitor diphenyleneiodonium renders Trichomonas vaginalis resistant to metronidazole, inhibits thioredoxin reductase and flavin reductase, and shuts off hydrogenosomal enzymatic pathways. Mol Biochem Parasitol 171:17–24. https://doi.org/10.1016/j.molbiopara.2010.01.001
Lipinski CA (2004) Lead- and drug-like compounds: the rule-of-five revolution. Drug Discov Today Technol 1:337–341. https://doi.org/10.1016/j.ddtec.2004.11.007
Mallo N, Lamas J, Sueiro RA, Leiro JM (2020) Molecular targets implicated in the antiparasitic and anti-inflammatory activity of the phytochemical curcumin in trichomoniasis. Molecules 25:5321. https://doi.org/10.3390/molecules25225321
Mavedzenge SN, van der Pol B, Cheng H et al (2010) Epidemiological synergy of Trichomonas vaginalis and HIV in Zimbabwean and South African women. Sex Transm Dis 37:460–466. https://doi.org/10.1097/OLQ.0b013e3181cfcc4b
McClelland RS, Sangaré L, Hassan WM et al (2007) Infection with Trichomonas vaginalis increases the risk of HIV-1 acquisition. J Infect Dis 195:698–702. https://doi.org/10.1086/511278
Meites E, Gaydos CA, Hobbs MM et al (2015) A review of evidence-based care of symptomatic trichomoniasis and asymptomatic Trichomonas vaginalis infections. Clin Infect Dis 61:S837–S848. https://doi.org/10.1093/cid/civ738
Menezes CB, Frasson AP, Tasca T (2016) Trichomoniasis – are we giving the deserved attention to the most common non-viral sexually transmitted disease worldwide? Microbial Cell 3:404–418. https://doi.org/10.15698/mic2016.09.526
Meyer Y, Buchanan BB, Vignols F, Reichheld J-P (2009) Thioredoxins and glutaredoxins: unifying elements in redox biology. Annu Rev Genet 43:335–367. https://doi.org/10.1146/annurev-genet-102108-134201
Miranda-Ozuna JFT, Hernández-García MS, Brieba LG et al (2016) The glycolytic enzyme triosephosphate isomerase of Trichomonas vaginalis is a surface-associated protein induced by glucose that functions as a laminin- and fibronectin-binding protein. Infect Immun 84:2878–2894. https://doi.org/10.1128/IAI.00538-16
Mora-Huertas CE, Fessi H, Elaissari A (2010) Polymer-based nanocapsules for drug delivery. Int J Pharm 385:113–142. https://doi.org/10.1016/j.ijpharm.2009.10.018
Ohkawa H, Ohishi N, Yagi K (1979) Assay for lipid peroxides in animal tissues thiobarbituric acid reaction. Anal Biochem 95:351–358
Pettersen EF, Goddard TD, Huang CC et al (2004) UCSF Chimera? A visualization system for exploratory research and analysis. J Comput Chem 25:1605–1612. https://doi.org/10.1002/jcc.20084
Rinaldo-Matthis A, Wing C, Ghanem M et al (2007) Inhibition and structure of Trichomonas vaginalis purine nucleoside phosphorylase with picomolar transition state analogues †. Biochemistry 46:659–668. https://doi.org/10.1021/bi061515r
Rocha-Garduño G, Hernández-Martínez NA, Colín-Lozano B et al (2020) Metronidazole and secnidazole carbamates: synthesis, antiprotozoal activity, and molecular dynamics studies. Molecules 25:793. https://doi.org/10.3390/molecules25040793
Sattar A, Chen D, Jiang L et al (2017) Preparation, characterization and pharmacokinetics of cyadox nanosuspension. Sci Rep 7:2289. https://doi.org/10.1038/s41598-017-02523-4
Schwebke JR, Barrientes FJ (2006) Prevalence of Trichomonas vaginalis isolates with resistance to metronidazole and tinidazole. Antimicrob Agents Chemother 50:4209–4210. https://doi.org/10.1128/AAC.00814-06
Seña AC, Bachmann LH, Hobbs MM (2014) Persistent and recurrent Trichomonas vaginalis infections: epidemiology, treatment and management considerations. Expert Rev Anti Infect Ther 12:673–685. https://doi.org/10.1586/14787210.2014.887440
Sena-Lopes Â, das Neves RN, Bezerra FSB et al (2017) Antiparasitic activity of 1,3-dioxolanes containing tellurium in Trichomonas vaginalis. Biomed Pharmacother 89:284–287. https://doi.org/10.1016/j.biopha.2017.01.173
Setzer M, Byler K, Ogungbe I, Setzer W (2017) Natural products as new treatment options for trichomoniasis: a molecular docking investigation. Sci Pharm 85:5. https://doi.org/10.3390/scipharm85010005
Shin JW, Jung KH, Lee ST et al (2014) Mefloquine improved progressive multifocal leukoencephalopathy in a patient with immunoglobulin A nephropathy. J Clin Neurosci 21:1661–1664
Sosa AM, Álvarez AM, Bracamonte E et al (2020) Efficacy of topical treatment with (-)-epigallocatechin gallate, a green tea catechin, in mice with cutaneous leishmaniasis. Molecules 25:1–9. https://doi.org/10.3390/molecules25071741
Sumangala V, Poojary B, Chidananda N et al (2010) Synthesis and antimicrobial activity of 1,2,3-triazoles containing quinoline moiety. Arch Pharmacal Res 33:1911–1918. https://doi.org/10.1007/s12272-010-1204-3
Stanzione F, Giangreco I, Cole JC 2021 Use of molecular docking computational tools in drug discovery. In: Progress in Medicinal Chemistry. Elsevier B.V., pp 273–343. https://doi.org/10.1016/bs.pmch.2021.01.004.
Tiuman TS, Ueda-Nakamura T, Garcia Cortez DA et al (2005) Antileishmanial activity of parthenolide, a sesquiterpene lactone isolated from Tanacetum parthenium. Antimicrob Agents Chemother 49:176–182. https://doi.org/10.1128/AAC.49.11.176-182.2005
Trott O, Olson AJ (2010) AutoDock Vina: improving the speed and accuracy of docking with a new scoring function, efficient optimization, and multithreading. J Comput Chem 31:455–461. https://doi.org/10.1002/jcc.21334
van der Pol B, Kwok C, Pierre-Louis B et al (2008) Trichomonas vaginalis infection and human immunodeficiency virus acquisition in African women. J Infect Dis 197:548–554. https://doi.org/10.1086/526496
Vique-Sánchez JL, Caro-Gómez LA, Brieba LG, Benítez-Cardoza CG (2020) Developing a new drug against trichomoniasis, new inhibitory compounds of the protein triosephosphate isomerase. Parasitol Int 76:102086. https://doi.org/10.1016/j.parint.2020.102086
Waterhouse A, Bertoni M, Bienert S et al (2018) SWISS-MODEL: homology modelling of protein structures and complexes. Nucleic Acids Res 46:W296–W303. https://doi.org/10.1093/nar/gky427
WHO (2012) Global incidence and prevalence of selected curable sexually transmitted infections - 2008. World Health Organization, Geneva
WHO (2018) Report on global sexually transmitted infection surveillance, 2018. World Health Organization, Geneva
Wierenga RK, Kapetaniou EG, Venkatesan R (2010) Triosephosphate isomerase: a highly evolved biocatalyst. Cell Mol Life Sci 67:3961–3982
Workowski KA, Bolan GA (2015) Sexually Transmitted Diseases Treatment Guidelines, 2015. Centers for Disease Control and Prevention (2015) MMWR 64 (RR03):1–137
Funding
This study was partially financed by the Coordenação de Aperfeiçoamento de Pessoal de Nível Superior—Brazil (CAPES)—Finance Code 001—Ph.D. Scholarship (MSDA, grant number 88882.346971/2019–01).
Author information
Authors and Affiliations
Contributions
Conceptualization: Mirna Samara Dié Alves, Ângela Sena-Lopes, Sibele Borsuk; Methodology: Mirna Samara Dié Alves, Ângela Sena-Lopes, Angela Maria Casaril; Investigation: Mirna Samara Dié Alves, Raquel Nascimento das Neves, Emerson Teixeira da Silva, Angela Maria Casaril, Micaela Domingues, Paloma Taborda Birmann; Formal analysis: Mirna Samara Dié Alves; Writing – original draft: Mirna Samara Dié Alves; Writing – review & editing: All authors; Project administration: Mirna Samara Dié Alves, Ângela Sena-Lopes, Sibele Borsuk; Supervision: Ângela Sena-Lopes, Sibele Borsuk; and Resources: Emerson Teixeira da Silva, Marcus Vinicius Nora de Souza, Lucielli Savegnago, Sibele Borsuk.
Corresponding author
Ethics declarations
Competing interests
The authors declare no competing interests.
Ethics approval
Not applicable.
Consent to participate
Not applicable.
Consent for publication
Not applicable.
Conflict of interest
The authors declare no competing interests.
Additional information
Handling Editor: Una Ryan
Publisher's note
Springer Nature remains neutral with regard to jurisdictional claims in published maps and institutional affiliations.
Rights and permissions
About this article
Cite this article
Alves, M.S.D., Sena-Lopes, Â., das Neves, R.N. et al. In vitro and in silico trichomonacidal activity of 2,8-bis(trifluoromethyl) quinoline analogs against Trichomonas vaginalis. Parasitol Res 121, 2697–2711 (2022). https://doi.org/10.1007/s00436-022-07598-1
Received:
Accepted:
Published:
Issue Date:
DOI: https://doi.org/10.1007/s00436-022-07598-1