Abstract
Ice decline is believed to benefit the pelagic food chain in Arctic shelf ecosystems, but the impacts of ice decline are usually difficult to detect owing to the overlap of ice decline with natural variability. To evaluate the responses of zooplankton communities to sea ice reduction in the Chukchi Sea, we combined zooplankton samples collected in the early summers of 2003, 2008, 2010, and 2012 and compared the inter-annual changes in the subregions with various physical and biological characteristics. Three geographically separate communities were identified by a hierarchical cluster analysis. The Bering Sea water influenced the central (CCS) and south (SCS) Chukchi Sea communities, which had total abundances that were approximately ten times higher than that of the north (NCS) Chukchi Sea community, and the inter-annual variability was dramatic. The SCS and CCS shared dominant taxa of Calanus glacialis, Pseudocalanus spp., barnacle larvae (nauplii and cypris), and Oikopleura vanhoffeni, while Pacific and neritic species were recognized as the dominant species in only the SCS. The inter-annual variations in the dominant species assemblages can be explained by the variability in oceanic circulation and the counteractions between copepods and barnacle larvae. Despite the numerical fluctuations, an increase in the average abundances in the Pacific-influenced region and the summer establishment of the C. glacialis population are proposed to be the most pronounced responses to ice decline. The NCS, which is governed by cold Arctic water, was characterized by low abundances and a constant dominant taxa assemblage. This area was also characterized by the presence of the high Arctic species Calanus hyperboreus and a lack of barnacle larvae. The total abundance of the NCS doubled from 2003 to 2008, while the community structure remained consistent. These results indicate that sea ice reduction has a positive effect on the zooplankton community, but heterogeneity is the main obstacle to the detection of the zooplankton community in the Western Arctic Ocean.
Similar content being viewed by others
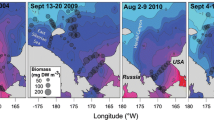
Explore related subjects
Discover the latest articles, news and stories from top researchers in related subjects.Avoid common mistakes on your manuscript.
Introduction
In response to climate forcing, dramatic ice retreat and far-reaching ecological consequences are expected in the Arctic Ocean, especially in productive shelf areas. According to a hypothetical scenario, the overall carbon and energy fluxes in coastal ecosystems will shift from a ‘sea-ice algae–benthos’ to ‘phytoplankton–zooplankton’ dominance, which is coincident with the change in the environment from a ‘cold/abundant ice’ to a ‘warm/limited ice’ mode (Piepenburg 2005). In the Chukchi Sea, where this hypothesis can likely be tested earlier than other marginal seas (Grebmeier et al. 2006), zooplankton consume only 5–15% of the total primary production under benthic dominance (Walsh et al. 1989). Thereafter, a switch in trophic structure means the multiplication of zooplankton production. Until now, warming trends have been established in studies on heat uptake, temperature, and ice coverage (Shimada et al. 2006; Stroeve et al. 2007; Comiso et al. 2008; Markus et al. 2009), but the demonstration of zooplankton responses lags far behind.
Despite the paucity of field observations, the detection of zooplankton trends is also impeded by the spatial heterogeneity of community composition. The zooplankton community structure in the Chukchi Sea is known to vary geographically with water mass configuration (Ashjian et al. 2003; Lane et al. 2008; Hopcroft et al. 2010). The zooplankton composition in the southern Chukchi Sea has an obvious Pacific feature (Hopcroft et al. 2010; Matsuno et al. 2011). As the Pacific waters are diluted by cold waters on the way to the Canada Basin, the proportions of both neritic and oceanic Arctic species increase. At the same time, both physical characteristics and zooplankton composition also differ among the Pacific waters that originate from the coastal or shelf regions of the Bering Sea (Hopcroft et al. 2010; Ershova et al. 2015). Furthermore, the zooplankton community in the Chukchi Sea is also characterized by high meroplankton abundances, which are similar to or exceed holoplankton abundances (Hopcroft et al. 2010). In addition to the different distribution patterns in the Arctic Ocean (Clough et al. 1997), meroplankton can potentially compete with copepods, as distinguished in temperate waters (Kirby et al. 2008).
Correspondingly, various zooplankton changes have been recorded in the western Arctic Ocean. The mean abundance remained consistent while the biomass increased in the Canada Basin between 2004 and 2008 (Hunt et al. 2014) when a drastic decrease in the area of sea ice was observed. In the Chukchi Sea, the abundance and biomass of zooplankton increased from 1991/1992 to 2007/2008, which was combined with a northern shift in the geographic distribution (Matsuno et al. 2011). In another study in the western Chukchi Sea, the biomass and abundance of zooplankton doubled in the summers of 2009–2012 compared with the summer of 2004 (Ershova et al. 2015).
Thus, we propose that the inter-annual variations of zooplankton in the responses to recent ice declines have spatial heterogeneity, which is determined by the zooplankton community structure. To examine the response of the zooplankton community and the potential spatial heterogeneity, we combined zooplankton samples from four summer cruises (2003–2012), covering a large area of the Chukchi Sea. The geographical distribution of the zooplankton communities was defined by the composition and abundance of each taxon, and the inter-annual variation in each community was analyzed in terms of dominant species, taxonomic groups, and total abundance. The specific objectives of this study are to (1) define the geographical distribution of the zooplankton communities in the Chukchi Sea, (2) illustrate the differences in the inter-annual changes among the various geographical communities, and (3) determine the change in zooplankton with ice retreat in terms of total abundance and community structure.
Materials and methods
Field observations were carried out during the Chinese Arctic Expedition in the summers of 2003, 2008, 2010, and 2012. A large area, including the slope area adjacent to the Canada Basin, was covered in 2003 and 2008, but the stations in 2010 and 2012 were limited to south of 72°N (Fig. 1). Zooplankton samples were collected from 29 July to 17 August in 2003 and 2008. During the other 2 years, field observations were conducted during July 18–26; however, station R02 was sampled on 7 July in 2010. To confirm the boundaries of the communities, four stations in the central region were resampled during a week in 2003, and the station numbers were addressed with a suffix of ‘-A’.
Sampling sites in the Chukchi Sea in 2003, 2008, 2012, and 2012. The shadowed area represents the floating ice coverage recorded by visual observations during field sampling, and the colored lines represent the ice edges on different dates (the blue, pink, green, yellow, and red lines represent the position of the ice edge on 30 June, 10 July, 20 July, 30 July, and 10 August, respectively). (Color figure online)
At each station, temperature and salinity in the water column were measured with a Seabird CTD (911 Plus). The in situ ice condition was estimated by visual observation and was expressed as the percentage of ice-covered area to the total area in sight. Sea ice extent, which is defined as the area covered at concentrations greater than 15%, was measured every 10 days from 30 June to 10 August with satellite data from National Snow and Ice Data Center (http://nsidc.org/data, Fig. 1). Zooplankton were collected with a North Pacific net (mouth opening: 0.5 m2; mesh size: 330 μm) that was towed vertically at a speed of 0.5 m s−1. The full water column (from 2 m above the bottom to the surface) was sampled at stations shallower than 200 m, and only the upper 200 m layer was sampled at deep stations. Immediately after capture, the zooplankton samples were preserved in buffered 5% formalin solution for species identification.
To determine the community composition and abundance, the formalin-preserved samples were processed under a dissecting microscope (Nikon, SMZ645) in the laboratory. All copepods, cnidarians, and chaetognaths except Harpacticoida were identified to the species or genus level. Prosome lengths and morphological characteristics were used to distinguish Calanus species (e.g., Hirche et al. 1994). Calanus marshallae was not distinguished from Calanus glacialis following the previous report that considered them a species-complex (Frost 1974). In the case of Pseudocalanus species, they were classified as Pseudocalanus spp. because of the difficulty in species-level identifications of copepodites. For other congeneric species, the identification of Metridia species (Metridia longa and Metridia pacifica) followed Brodsky (1967), and the identification of Microcalanus species (Microcalanus pusillus and Microcalanus pygmaeus) followed Brodsky et al. (1983). The copepodite stages of C. glacialis and Calanus hyperboreus were largely separated by prosome length. Barnacle larvae, which occurred at a high abundance, were identified separately as cypris and nauplii, but other meroplankton were grouped at the macrotaxa level. Other holoplanktonic crustaceans, with only immature individuals captured, were grouped to the family or order level.
To simplify the community structure, we categorized all taxa into 8 ecological groups according to their size-spectra, feeding habit, and trophic function. These taxa are copepods, cnidarians, chaetognaths, tunicates, barnacle larvae, meroplankton, other crustaceans, and others. Because of the high abundance, barnacle larvae were separated from meroplankton. The holoplanktonic species were divided at the phylum level, except for copepods, which were distinguished from other crustaceans. Furthermore, the occasionally present taxa, including fish eggs and juveniles, floating silkworms, and pteropods, were combined in the others group.
A dominance index (Y) was applied to identify the dominant species in each community using the equation:
where Yij is the dominance index of species i in community j, nij is the total number of individuals of species i in community j, and Nj is the total number of individuals of all zooplankton species in community j. fij is the occurrence frequency of species i, which is defined as
where Sij is the number of samples in group j where species i is present, and Sj is the number of samples in group j. Y value of ≥ 0.02 was selected as the cut-off point for a dominant taxon using this method.
The geographical pattern of the community was analyzed with the abundances of the taxonomic categories identified in our study. After log-transformation, the Bray–Curtis similarity index was calculated for all stations in each year. A Q-type cluster analysis was carried out using the complete linkages, and a qualitative separation of groups was established by the overall similarity. Because log-transformations will strongly increase the relative influences of rare zooplankton groups, we selected the taxonomic categories that contributed at least 1% to any sample (e.g., Hopcroft et al. 2010). The SIMPER program (similarity percentage analysis) was used to assess the percent contribution of the zooplankton taxa to the community-clusters and similarities between groups. All cluster analyses were processed with PRIMER 6.0 software, and the geographical distribution maps were prepared by Surfer 10.0, Ocean Date View 4.5.
Results
Environmental conditions
Only floating ice was observed by visual measurement (Fig. 1). The highest ice coverage was recorded in 2003, with floating ice present at all stations north of 71°N. The percentage of coverage was greater than 50% at half of the stations, and the highest coverage of 80% was observed at stations R14 and C06. Floating ice was recorded in similar geographical areas in 2008, but the percentage of coverage was less than 30%. As all stations were located in coastal regions, floating ice was absent in 2010. Sea ice was present in only the most northern transects in 2012. The percentage of coverage was 80% at station R05 and less than 20% at the other stations.
Temperature and its geographical variability were lowest in the north of Chukchi Sea, where a cold water mass (< 0 °C) was observed (Fig. 2). A thermal front was present between 69 and 72°N, where the temperature increased from 0 to 7 °C in the surface layer. In the south and central shallow areas, temperature varied with the distance to the coastline. In 2003, 2008, and 2012, high temperatures of 15°C were recorded at the station near Cape Lisburne, whereas the opposite trend was observed in 2010. The temperature was high at central station CC2. Surface salinity was also lowest in the north, and salinities lower than 28 were observed only in 2008. The salinities in shallow areas varied between 30.2 and 32.8, with the highest value observed near the Bering Strait.
Species composition and community division
We recorded 42 species and 16 higher taxa that could not be identified to the species level over the study period (Table 1). With 20 species and four unspecified taxa (Pseudocalanus spp., Oncaea spp., Harpacticoida and nauplii), copepods were the most diverse and widespread group, but the copepod abundance was lower than that of barnacle larvae on average in all investigation years. The total abundance of other taxa was far less than the abundances of copepods and barnacle larvae.
Three geographical communities were identified from the hierarchical cluster of zooplankton species composition and relative abundance, corresponding to the north (NCS), central (CCS), and south (SCS) Chukchi Sea (Figs. 3, 4). The NCS covered in only 2003 and 2008 was divided from the other two communities with similarity values less than 20%. Among the 4 years investigated, the CCS and SCS communities shared similarities that varied between 40 and 50%. Along the longitudinal transect of 169°W, the boundary between the NCS and CCS was located at station R12 (72.5°N) in 2003, as it was grouped with the NCS by the first sample but the CCS by the second sample (R12A). In 2008, R13 (73.0°N) was identified as the northernmost station of the CCS, but the abundance values were similar to those from the NCS stations and were only 10−1 of the other CCS stations. In the eastern part near the Beaufort Sea, two shelf stations (C10A and C17) were also included in the NCS in 2008. The geographical range of the shallow water communities varied from year to year. The SCS community covered a large area in 2003 and 2008 but was overwhelmingly limited to the south of Cape Lisburne in 2010 and 2012. Station C04 in the central part was also included with the SCS in 2012. The analysis of similarity (ANOSIM) showed significant differences among the communities each year (2003: p = 0.001; 2008: p = 0.001; 2010: p = 0.014; 2012: p = 0.002).
Geographic distribution of the three zooplankton communities determined by hierarchical clustering of log-transformed abundance and total zooplankton abundance at each station (red open circle: south Chukchi Sea community; blue open circle: central Chukchi Sea community; black open circle: north Chukchi Sea community). (Color figure online)
According to the physical conditions, the NCS and CCS communities were characterized by surface freshening and low temperatures. In the NCS, the surface salinity varied from 28.5 to 29.8 in 2003 and from 25.7 to 30.7 in 2008, but in the bottom layer, it ranged from 32.6–32.7 to 31.5–33.2 in 2003 and 2008, respectively. The surface salinity was governed by cold Arctic waters, and floating ice was commonly observed. The average temperatures were − 1.3 and − 1.0 °C in 2003 and 2008, respectively. Surface freshening was also evident, especially in 2008 (29.3). Generally, colder and fresher water was present in the CCS compared to the SCS (Fig. 5a). Both the average temperature and salinity in the CCS were lower than those in the SCS each year, but the differences in salinity in 2008 (31.9 and 31.8) and temperature in 2012 (3.1 and 2.5 °C) were small.
Inter-annual variations of the column-averaged temperature and salinity (a) and abundance of each taxonomic group (b). The circles and lines show the trends of the average total abundance in the entire Pacific-influenced region, which were calculated from all stations included in the SCS (south Chukchi Sea) and CCS (central Chukchi Sea) communities
The NCS was characterized by low zooplankton abundances, with averages of 118.9 and 182.5 ind. m−3 in 2003 and 2008, respectively (Fig. 5b). In 2003, the abundance was lowest at 40.9 ind. m−3 at the southernmost R12 station, and highest (235.9 ind. m−3) in the second sample at R14. The lowest abundance in 2008 was observed at the northernmost B11 station (50.5 ind. m−3), and the highest abundance of 435.2 ind. m−3 was present at station S12 near the Beaufort Sea. On average, much higher abundances were recorded in the CCS and SCS, with annual averages between 570.4 and 2415.3 ind. m−3. In these Pacific-influenced communities, the lowest abundances were observed in the central area in 2003 (236.6 ind. m−3 at station C21) and 2012 (407.8 ind. m−3 at station C05). Except for the northernmost station R13, the total abundance was lowest at 233.5 ind. m−3 at C35 near Cape Lisburne in 2008. The lowest abundance of 283.2 ind. m−3 in 2010 was recorded at C09 near the slope region of the Beaufort Sea.
Community structure and variability
On the proportion of functional groups, the NCS can be distinguished by the dominance of copepods and lack of barnacle larvae (Fig. 5b). According to the SIMPER (similarity percentages-species contributions) analysis, copepods and barnacle larvae were most important to the division of communities. The abundance of copepods was 101.2 and 168.0 ind. m−3 on average, and its proportion at each station varied between 82.0 and 96.1% in 2003 and 90.3 and 94.6% in 2008. Second in abundance, barnacle larvae accounted for proportions of only 3.2 and 1.9% in 2003 and 2008, respectively. Similarly, the total abundances increased from 2003 to 2008, and this increase was mostly induced by the increase in copepods, among which a numerical increase was observed in the widespread species Pseudocalanus spp., O. similis and C. glacialis, but increases were absent in the large Arctic copepod C. hyperboreus and unidentified copepod nauplii.
Copepods and barnacle larvae co-dominated in the CCS and SCS communities, but no consistent pattern was observed in their relative contributions to total abundance (Fig. 5b). In 2012, copepods prevailed in the CCS, with abundances of 294.2–1547.9 ind. m−3 and proportions of 62.3–96.3%, whereas barnacle larvae dominated in the SCS. The abundance of barnacle larvae varied between 727.4 and 2645.1 ind. m−3, accounting for 56.6–79.8% of the total abundance. Conversely, in 2010, barnacle larvae were scarce in the SCS and showed large spatial differences in the CCS. The abundance of barnacle larvae reached 8206.9 ind. m−3 at station C06 and was only 23.6 ind. m−3 at station C09.
On annual averages, these two groups accounted for an overwhelming majority (94.8–97.0%) in the CCS and SCS, but the other groups contributed much higher proportions in the SCS than in the CCS. In 2008, other meroplankton (mostly Gastropoda larvae) and cnidarians accounted for proportions of 21.2 and 10.9% of the total abundances. Comparatively high proportions were observed in cnidarians (4.3%) and tunicates (4.3%) in 2003, and chaetognaths (4.5%) and tunicates (5.3%) in 2010. In 2003 and 2008, barnacle larvae presented higher proportions than copepods in both communities, but they were scarce in the SCS in 2010 and CCS in 2012. Correspondingly, copepods constituted a vast majority of 80.5 and 78.4% of the total abundances.
In the CCS and SCS communities, the average abundances fluctuated during 2003–2012 in each community but increased overall (Fig. 4b). From 2003 to 2008, the abundances in the CCS and SCS also increased from 852.4 and 1410.1 ind. m−3 to 928.5 and 1738.2 ind. m−3, but the abundances of copepods decreased from 321.5 and 579.8 ind. m−3 to 184.4 and 224.3 ind. m−3, respectively. The numerical increase in the CCS was mainly induced by barnacle larvae (758.6–1461.9 ind. m−3), and that in the SCS was induced by cnidarians and other meroplankton. The abundance in the SCS was lowest in 2010 and highest in 2012, and that in the CCS exhibited the opposite trend. The lowest abundance in these communities was induced by the scarcity of barnacle larvae, which decreased to 45.7 and 178.0 ind. m−3. Barnacle larvae were extremely abundant in the CCS in 2010, especially at station C06 where the highest record of 8529.1 ind. m−3 was reached. On average, the abundance in these two communities increased from 1049.8 to 1749.5 ind. m−3.
Dominant species
In total, 13 taxa were recorded in various communities with dominance indexes greater than 0.02 (Table 2). Among these dominant taxa, only C. hyperboreus was recognized as an Arctic species, and its distribution was limited in the NCS community. In the northern part of the Chukchi Sea, C. hyperboreus was present in only deep waters, whereas in the eastern part adjacent to the Beaufort Sea, it spread into the shelf waters.
Among the four widespread dominant taxa, Pseudocalanus spp. and O. vanhoffeni were less abundant in the NCS than the other communities, but Oithona similis was most abundant (51.0 ind. m−3) in the NCS in 2008. All dominant species of the CCS were included in the dominant taxa of the SCS (Table 2), and their distributions varied geographically (Fig. 6). The abundance of C. glacialis exhibited the most dramatic inter-annual variation. The abundance of C. glacialis was 549.5 ind. m−3 in the CCS in 2003 but only 35.1 and 28.6 ind. m−3 in the other areas, and in 2012, comparatively high abundances of 460.1 and 684.4 ind. m−3 were recorded in both communities. Barnacle nauplii and cypris dominated in the shallow water communities during all cruises, but these groups were hardly detected in the NCS community. On average, cypris was more abundant than nauplii in all investigation years except 2010.
Comparatively, the dominant taxa that were recognized exclusively in the SCS community were present in dense abundances at only a few stations (Fig. 7). Acartia longiremis was dominant in 2003 and 2010, and the species was concentrated near the Cape Lisburne. High abundances of Macrura larvae were observed in the same area in 2008. E. bungii was sampled mostly in the central part of the south Chukchi Sea. O. longissima was absent in 2010 but was present at a dense abundance (454.6 ind. m−3) at one station in 2008. A high abundance of decapod larvae was observed at only the southernmost station in 2008. Unspecified copepod nauplii were more abundant in shallow waters, but they were recognized as dominant taxa only in the NCS community, owing to the lower total abundance.
In the NCS, dominant taxa were identified in same ranks in the two cruises, except O. vanhoffeni had a dominance index less than 0.02 in 2008. These dominant taxa accounted for 80.5 and 84.8% of the total abundance. Five dominant taxa were included in the CCS in 2003 and 2012, accounting for 87.8 and 93.3% of the total abundance, respectively, but only three of these taxa were recorded in 2008 and 2010, accounting for proportions of 95.1 and 93.0%, respectively. Barnacle nauplii and cypris were the most important dominant taxa of this community from 2003 to 2010, contributing 53.1, 84.1 and 89.8% to the total abundance, whereas C. glacialis was most abundant (684.4 ind. m−3) in 2012. The dominant taxa in the SCS contributed 83.9, 90.8, 90.7, and 93.1% of the total abundances in the four cruises. Barnacle cypris was most abundant in 2003, 2008, and 2012, whereas Pseudocalanus spp. was most abundant in 2010.
Among the dominant species, a significant increase from 2003 to 2012 was observed in only C. glacialis. In the SCS, the abundance of this species was less than 100.0 ind. m−3 from 2003 to 2010, but it increased to 460.1 ind. m−3 in 2012. This species was also scarce in the CCS in 2008 and 2010, but a comparatively high abundance of 274.7 ind. m−3 was recorded in 2003. In 2012, the abundance of this species reached the highest of 684.4 ind. m−3.
Discussion
The geographical separation of zooplankton communities in our study makes it feasible to compare the inter-annual variabilities among species assemblages in similar habitats. Clarifying the advection-related temporal fluctuations also makes the increasing trends of total abundance that are demonstrated in the north shelf and Pacific-influenced regions more reliable. Here, we analyze the variations in the abundances of taxonomic groups and dominant species in relation to environmental change to show the zooplankton assemblages in various habitats and assess how the zooplankton community will change with ice decline. To distinguish the potential effects of warming, the influences by direct transportation were evaluated in the context of observed physical and biological change.
Causes of spatial heterogeneity
In previous studies, various zooplankton communities had been identified in the Chukchi Sea in relation to the physical characteristics of the water masses or the trophic structures of the coastal ecosystems (Lane et al. 2008; Hopcroft et al. 2010). The distribution of zooplankton communities along longitudinal gradients was recorded when the entire shelf region of the Chukchi Sea was considered (Matsuno et al. 2011). From the samples from the east–west transects in the south Chukchi Sea, various communities can be distinguished based on the impacts from Pacific waters with different origins (Hopcroft et al. 2010). In limited regions with well-defined pelagic–benthic coupling patterns, the zooplankton communities may be distinguished based on competition with planktonic larvae or settled adults (Day et al. 2013). Owing to the large coverage area and the lack of longitudinal gradients, the zooplankton communities in our study were geographically divided into latitudinal gradients. Our study covered the eastern part of the Chukchi Sea, and the far-north stations in 2003 and 2008 reached the slope area between the Chukchi Sea and the Canada Basin. Thus, the geographical differences among the communities mostly resulted from the location, which resulted in various Pacific influences.
In the NCS, the low temperatures and salinities indicated governance by Arctic winter and melt waters and the absence of nutrient-rich Pacific waters. Accordingly, the zooplankton abundance in this community was one order of magnitude lower than that in the Pacific-influenced regions. At the same time, the species composition in the NCS showed high similarity with that in the south Beaufort Sea, sharing the same dominant Arctic species except for Limacina helicina (Hunt et al. 2013). In addition to the previous reports that the Arctic assemblage appears to be similar to that in the northwestern Chukchi shelf northward of Wrangell Island where melt and winter water predominate (Ershova et al. 2015), our results also suggest that the total zooplankton abundance in this region is not comparable with that in the CCS and SCS.
The replicated sampling makes it possible to locate the boundary areas between 72 and 73°N, which are coincident with the shelf break where the Bering Sea water turns eastward (Pickart et al. 2013). At the border station (e.g., R12 in 2003), a zooplankton community that was characteristic of the NCS was substituted by a community that was structured of the CCS in 10 days, and the total abundance increased by one order of magnitude. Accompanied by the abundance increases in barnacle larvae and C. glacialis, warm water intrusion was observed in the surface and subsurface layers. This finding indicated that mixing of water masses with various origins could exert a significant influence on the abundance and composition of zooplankton communities in the boundary area with temporal fluctuations.
Comparatively, the Pacific-influenced SCS and CCS communities shared similarly high total zooplankton abundances and most of the dominant species (Table 2). However, these communities can still be distinguished through both physical characteristics and species diversity in all sample years except in 2012. From 2003 to 2010, the average temperature varied between 3.2 and 4.6 °C in the CCS, but it varied between 0.5 and 0.9 °C in the SCS. In addition to the dominant species that were shared by both communities, seven taxa (A. longiremis, E. bungii, O. longissima, Gastropoda larvae, Macrura larvae, Sagitta elegans, and O. similis) were dominant species in only the SCS, and these taxa originated from coastal or Pacific waters. In previous studies, A. longiremis and E. bungii were concentrated in coastal and Anadyr waters, respectively (Springer et al. 1989). O. longissima and S. elegans were widely distributed in the coastal Arctic and sub-Arctic waters. Bivalvia and Macrura larvae have been recorded near the Bering Strait (Hopcroft et al. 2010). O. similis is a cosmopolitan species that is dominant in the SCS but scarce in the CCS, which indicates that this species is transported by Pacific inflows.
Another reason for spatial heterogeneity is the mass occurrence of benthic larvae. In previous reports, these larvae tended to be concentrated in coastal waters, as their distributions were determined by locations of adults as well as currents (Schlüter and Rachor 2001). In our study, this pattern was true for Macrura larvae, which were mostly concentrated in the SCS. However, high abundances of barnacle larvae were also observed in the central area of the Chukchi Sea. In the Kara Sea, mature females were not observed, which suggested that these larvae originated from the coasts or rocky bottoms (Fetzer and Arntz 2008). The Chukchi barnacle, Balanus crenatus, can also settle on hard surfaces of other marine invertebrates (Slattery and Oliver 1987). Thereafter, adult barnacles are also expected in areas with soft bottoms, where they can benefit from high food availability and low predation risks (Barnes and Bagenal 1951).
Causes of inter-annual variation
In the NCS community where the dominant species were consistent, inter-annual changes were mainly detected in abundance, whereas in the Pacific-influenced communities, inter-annual changes were recorded in both structure and abundance. The abundance fluctuations in the CCS and SCS were mostly governed by the barnacle larvae fluctuations, which differed by one order of magnitude from year to year. The inter-annual variability in the dominant species of the SCS was characterized by the occasional presence of Pacific species, but that in the CCS was identified as non-dominance of C. glacialis and O. vanhoffeni in the 2 years with barnacle larval blooms.
As the determinate factor of spatial heterogeneity, physical transportation may also exert critical influences on the inter-annual changes in community structure and abundance. In the SCS, community structure was much different in 2010 and was characterized by the lowest total abundance and lowest contribution by barnacle larvae. Correspondingly, E. bungii was most abundant in this community. In a previous report, a higher abundance was also observed in early August of the same year (Ershova et al. 2015). Compared to other species that are recognized as Bering Oceanic (Hopcroft et al. 2010), such as C. glacialis, recruitment of E. bungii has not been reported in the Chukchi Sea, and thus, it has been indicated as a better indicator of Anadyr water intrusion. Simultaneously, the absence of warm water near Cape Lisburne in 2010 indicated shrinkage of Alaskan Coastal Water. According to a study in the northeast Chukchi Sea, cold oceanographic conditions can likely slow the growth and development of zooplankton (Questel et al. 2013). In this study, a developmental lag possibly occurred in barnacle larvae, as the highest abundances and prevalences of nauplii were observed at the coastal stations. In the years with fewer Bering Sea species, the abundances of coastal species increased correspondingly, especially in 2008. In previous reports, low abundances of O. longissima were recorded, but this species was recognized as dominant in our study in 2008.
Variability of the community structure in the CCS was observed in 2012, which manifested as the dominance of large copepods and decreased abundances of barnacle larvae. In 2012, the water temperature was less variable in the investigation area. Species from the Bering Sea were also scarce or absent. Floating ice was recorded at most stations in the CCS in 2012, while it was absent in other years. Both laboratory and field studies indicated that the release time of barnacle larvae is strongly affected by the concentration of phytoplankton (Clare et al. 1984; Clare and Walker 1986). On the other hand, ice retreat is a key factor that stimulates the onset of phytoplankton blooms (Wang et al. 2005; Arrigo et al. 2012), and the major factor that affects ice retreat is considered to be the inflow of warm Pacific summer water (Shimada et al. 2006; Woodgate et al. 2010). This result indicated that coastal species such as barnacle larvae are strongly affected by the inflow of Bering Sea water. However, although the annual transport volume of Bering Sea water was highest in 2010 (Woodgate et al. 2015), the total zooplankton abundance was lowest in the SCS community. Whereas, the highest abundance was observed in the CCS community in this year, and barnacle nauplii contributed most to this high abundance.
Potential inter-species interactions, notably between barnacle larvae and copepods, are another reason for the inter-annual fluctuations in total abundance. Barnacle larvae feed on a wide range of food organisms, including phytoplankton (Turner et al. 2001; Vargas et al. 2006; Gaonkar and Anil 2010), and these larvae can be important competitors to copepods in both temperate and polar regions. In the Kara Sea, planktonic larvae of Balanus sp. were present as early as February (Fetzer and Arntz 2008) when feeding is not active in most copepod species. In temperate waters, climate-induced effects were observed on the meroplankton, which were coincident with the increased phytoplankton and declining holoplankton abundances (Kirby et al. 2008). Under normal phenological conditions, these species might respond to the spring blooms more rapidly than the advected populations (Schlüter and Rachor 2001; Willis et al. 2006). In our study, a positive correlation was observed in the abundances of these species in the SCS, but the correlation was negative in the CCS (Fig. 8). This result suggested that competition was less evident in shallow waters with lower standing zooplankton stocks and higher nutrition supplies.
Correlation between the abundance of barnacle larvae (AB) and those of copepods (AC) and Calanus glacialis (ACg). Positive and negative correlations were found in the SCS (south Chukchi Sea) and CCS (central Chukchi Sea) communities, respectively, and the negative correlation is acceptable in the CCS (Pearson correlation, 2-tail, p = 0.014 between AB and AC, p = 0.014 between AB and ACg)
Reliability of the increasing trends
Even though the NCS community was investigated in only 2003 and 2008, the increased zooplankton abundances showed strong coincidence with the rapid thinning and declining of ice during this period (Kwok and Untersteiner 2011). In this study, the water temperature increased, and the salinity decreased from 2003 to 2008. According to the visual estimation, the ice coverage decreased by 20–30%. The consistent community structure showed that the total abundance and species origins were comparable, which made the year to year increase more reliable. Despite the fact that the species compositions were similar to those in the Canada Basin, a numerical increase that was induced by small copepods was observed in this community, instead of the biomass increase that was contributed by large copepods (Hunt et al. 2014). It has been proposed that dependence on autochthonous basin production by short-lived species results in decreased secondary production under low chlorophyll a biomass conditions in the Canada Basin. However, comparatively high chlorophyll a concentrations were recorded in the NCS in our study, and high nutrient concentrations that have also been reported (Zhang et al. 2015) in this area also indicate that the primary production increased with ice thinning. These small species, with sustained reproductive periods and short life cycles, are therefore expected to be highly adaptable to changing conditions (Ashjian et al. 2003; Lischka and Hagen 2005).
With significant inter-annual fluctuations, the increasing trends in the total zooplankton abundance were observed in only the Pacific-influenced shelf region, rather than in each community. Although the differences in the temporal and spatial coverages of the field sampling may reduce the comparability of the community structure, we still believe that the increasing trend is reliable. First, the establishment of an increasing trend in each community was prevented by the extremely low abundances in 2010 and 2012, both of which were induced by the scarcity of barnacle larvae. Suffering both direct impacts from environmental anomalies and indirect influences from benthic adults, the abundance of barnacle larvae showed higher inter-annual variability than copepods, and it is more difficult to track these fluctuations (Clough et al. 1997). The low abundances of barnacle larvae in the SCS in 2010 may be correlated with the strengthening of Bering oceanic water, which is indicated by the comparatively high E. bungii abundance, and the decrease of the Alaska Coastal Current, which is judged by the lack of warm water near Cape Lisburne. Although there were no clues to why the abundances of barnacle larvae in the CCS were low in 2012, the similarly low abundances in Macrura larvae in 2010 and 2012 indicated that these low abundances might arise from benthic adult anomalies rather than direct environmental changes. The highest water temperature and dominance of cypris in the CCS in 2012 suggested the possibility of advanced settlement.
Second, the extremely high abundance of C. glacialis in July of 2012 when the summer ice coverage was minimum is most likely a result of ice decline. Consistently, a significant increase in the abundance of C. glacialis was also recorded in September of the same year (Ershova et al. 2015; Pinchuk and Eisner 2017). The original scarcity of C. glacialis on the Chukchi shelf may be a result of the ice coverage and the < 100 m depth, which do not satisfy the overwintering requirements (Hirche 1991; Conover and Siferd 1993; Ashjian et al. 2003). Still, it is uncertain if C. glacialis can reproduce successfully in the Chukchi Sea. Advection from the Bering Sea shelf was proposed for the copepodite stages within the offshore section of the Southern Chukchi Sea and the eastern side of the Herald Canyon (Baier and Napp 2003; Ershova et al. 2015). However, integration of the abundance and population structure in July and September can ensure that the massive population that developed from C1 & C2 to C5 was dominant in the Chukchi Sea during this period. Based on the higher lipid contents in the locally recruited individuals, the summer conditions in the Chukchi Sea are more favorable than those in the North Pacific Ocean (Mohan et al. 2016). This result suggests that with sufficient supply of overwintered individuals, they can successfully recruit in the Chukchi Sea.
On the other hand, local reproduction cannot be denied. First, the abundances recorded in 2012 were even higher than those recorded in most previous reports and the synchronous results in the north Bering Sea (Durbin and Casas 2013; Eisner et al. 2013; Pinchuk and Eisner 2017), and equivalent abundances were observed in only Kongsfjorden, Svalbard in June 2006 (Daase et al. 2013). Second, the timing of the presence of early stages in our study showed better coincidence with the timings in other Arctic shelf waters than those in the Bering Sea. Egg production in the Northern Bering Sea peaked before ice melt and almost ceased in early April (Daase et al. 2013; Durbin and Casas 2013). Based on a duration of 44 days from egg to nauplii stage N6 (Jung and Nielsen 2015), the occurrence of C1 was expected no later than May, following the disappearance of nauplii in April in the field observations (Durbin and Casas 2013). The transport time of the Alaska Coastal Current from the Bering Strait to Pt. Barrow is estimated to be approximately 4 months, which is based on a current velocity of 10 cm s−1 (Woodgate et al. 2005). We thus suggest that the early stages in the CCS cannot be transported from the north Bering Sea.
Another potential reason for the massive occurrence of C. glacialis is their competitive ability against barnacle larvae. This copepod can spawn as early as March/April and as late as August/September, depending on the sea ice conditions that largely determine the onset of the algal growth season (Hirche 1989; Søreide et al. 2008). Although barnacle larvae are present in the water column as early as February, dense occurrences were observed mainly in July and August (Fetzer and Arntz 2008). At their release, separate habitats have been suggested for the oceanic copepod C. glacialis. In our study, there is a significant correlation between C. glacialis and barnacle larvae in the CCS (Fig. 8). At the same time, according to our results, C. glacialis may have established a large population consisting of copepodites when they encountered the competition by dense barnacle larvae. Within the Alaska Coastal Water and north of 74°N, the population of C. glacialis mainly consisted of C5 copepodites in all sample years except 2012, while the population mainly consisted of C1–C3 copepodites in the area adjacent to the Canadian Basin. C1–C3 copepodites dominated in the south and central Chukchi Sea in 2003 and 2010, while C4–C5 copepodites dominated in 2008. The composition of population stages in 2012 was different from the compositions in other years. C5 copepodites and adults were scarce or absent, and the populations mainly consisted of C1–C4 copepodites (82.7–99.5%) in all stations.
Conclusions
Our results can be used to delimit the boundary between the Pacific-influenced and north shelf communities in the Chukchi Sea, which differ in total zooplankton abundance by one order of magnitude. In the northern shelf community where the dominant species assemblages are consistent, the response to ice decline can be detected in the total abundances and contributions of various taxa. Owing to the significant inter-annual fluctuations in structure and abundances of the communities, numerical increases in response to ice decline were observed in only the Pacific-influenced region, and the interaction between copepods and barnacle larvae is proposed as an important reason for the variabilities in these communities. The extremely high abundance of C. glacialis is the most interesting response phenomena on the species level. Along with previous reports, our results prove that at least early copepodite development was accomplished in the shallow Chukchi Sea with high recruitment success.
References
Arrigo KR, Perovich DK, Pickart RS et al (2012) Massive phytoplankton blooms under Arctic sea ice. Science 336(6087):1408
Ashjian CJ, Campbell RG, Welch HE, Butler M, Van Keuren D (2003) Annual cycle in abundance, distribution, and size in relation to hydrography of important copepod species in the western Arctic Ocean. Deep Sea Res Part I 50(10):1235–1261
Baier CT, Napp JM (2003) Climate-induced variability in Calanus marshallae populations. J Plankton Res 25(7):771–782
Barnes H, Bagenal TB (1951) Observations on Nephrops norvegicus (L.) and on an epizoic population of Balanus crenatus Brug. J Mar Biol Assoc UK 30(2):369–380
Brodsky KA (1967) Calanoida of the far-eastern seas and polar basin of the USSR. Israel Program Scientific Translation, Jerusalem
Brodsky KA, Vyshkvartzeva NV, Kos MS, Markhaseva EL (1983) Copepoda Calanoida of the seas of the USSR and adjastent seas. Opredeliteli po faune SSSR 35:1–358
Clare AS, Walker G (1986) Further studies on the control of the hatching process in Balanus balanoides (L.). J Exp Mar Biol Ecol 97(3):295–304
Clare AS, Walker G, Holland DL, Crisp DJ (1984) Nature of the barnacle hatching substance and the role of dopamine in the egg hatching process. In: Engles W (ed) Advances in invertebrate reproduction, vol 3. Elsevier Science Publishers B.V, Amsterdam, p 569
Clough LM, Ambrose WG, Cochran JK (1997) Infaunal density, biomass and bioturbation in the sediments of the Arctic Ocean. Deep Sea Res Part II 44(8):1683–1704
Comiso JC, Parkinson CL, Gersten R, Stock L (2008) Accelerated decline in the Arctic sea ice cover. Geophys Res Lett. https://doi.org/10.1029/2007GL031972
Conover RJ, Siferd TD (1993) Dark-season survival strategies of coastal zone zooplankton in the Canadian Arctic. Arctic 46(4):303–311
Daase M, Falk-Petersen S, Varpe Ø et al (2013) Timing of reproductive events in the marine copepod Calanus glacialis: a pan-Arctic perspective. Can J Fish Aquat Sci 70(6):871–884
Day RH, Weingartner TJ, Hopcroft RR et al (2013) The offshore northeastern Chukchi Sea, Alaska: a complex high-latitude ecosystem. Cont Shelf Res 67:147–165
Durbin EG, Casas MC (2013) Early reproduction by Calanus glacialis in the Northern Bering Sea: the role of ice algae as revealed by molecular analysis. J Plankton Res 36(2):523–541
Eisner L, Hillgruber N, Martinson E, Maselko J (2013) Pelagic fish and zooplankton species assemblages in relation to water mass characteristics in the northern Bering and southeast Chukchi seas. Polar Biol 36(1):87–113
Ershova EA, Hopcroft RR, Kosobokova KN (2015) Inter-annual variability of summer mesozooplankton communities of the western Chukchi Sea: 2004–2012. Polar Biol 38(9):1461–1481
Fetzer I, Arntz WE (2008) Reproductive strategies of benthic invertebrates in the Kara Sea (Russian Arctic): adaptation of reproduction modes to cold water. Mar Ecol Prog Ser 356:189–202
Frost BW (1974) Calanus marshallae, a new species of calanoid copepod closely allied to the sibling species C. finmarchicus and C. glacialis. Mar Biol 26(1):77–99
Gaonkar CA, Anil AC (2010) What do barnacle larvae feed on? Implications in biofouling ecology. J Mar Biol Assoc UK 90(6):1241–1247
Grebmeier JM, Overland JE, Moore SE et al (2006) A major ecosystem shift in the northern Bering Sea. Science 311(5766):1461–1464
Hirche HJ (1989) Egg production of the Arctic copepod Calanus glacialis: laboratory experiments. Mar Biol 103(3):311–318
Hirche HJ (1991) Distribution of dominant calanoid copepod species in the Greenland Sea during late fall. Polar Biol 11(6):351–362
Hirche HJ, Hagen W, Mumm N, Richter C (1994) The Northeast Water Polynya, Greenland Sea. III. Meso- and macrozooplankton distribution and production of dominant herbivorous copepods during spring. Polar Biol 14:491–503
Hopcroft RR, Kosobokova KN, Pinchuk AI (2010) Zooplankton community patterns in the Chukchi Sea during summer 2004. Deep Sea Res Part II 57(1):27–39
Hunt GL, Blanchard AL, Boveng P et al (2013) The Barents and Chukchi Seas: comparison of two Arctic shelf ecosystems. J Mar Syst 109:43–68
Hunt BPV, Nelson RJ, Williams B et al (2014) Zooplankton community structure and dynamics in the Arctic Canada Basin during a period of intense environmental change (2004–2009). J Geophys Res Ocean 119(4):2518–2538
Jung MS, Nielsen TG (2015) Early development of Calanus glacialis and C. finmarchicus. Limnol Oceanogr 60(3):934–946
Kirby RR, Beaugrand G, Lindley JA (2008) Climate-induced effects on the meroplankton and the benthic-pelagic ecology of the North Sea. Limnol Oceanogr 53(5):1805–1815
Kwok R, Untersteiner N (2011) The thinning of Arctic sea ice. Phys Today 64(4):36–41
Lane PV, Lliná L, Smith SL, Pilz D (2008) Zooplankton distribution in the western Arctic during summer 2002: hydrographic habitats and implications for food chain dynamics. J Mar Syst 70(1):97–133
Lischka S, Hagen W (2005) Life histories of the copepods Pseudocalanus minutus, P. acuspes (Calanoida) and Oithona similis (Cyclopoida) in the Arctic Kongsfjorden (Svalbard). Polar Biol 28(12):910–921
Markus T, Stroeve JC, Miller J (2009) Recent changes in Arctic sea ice melt onset, freezeup, and melt season length. J Geophys Res Ocean. https://doi.org/10.1029/2007GL031972
Matsuno K, Yamaguchi A, Hirawake T, Imai I (2011) Year-to-year changes of the mesozooplankton community in the Chukchi Sea during summers of 1991, 1992 and 2007, 2008. Polar Biol 34(9):1349–1360
Mohan SD, Connelly TL, Harris CM, Dunton KH, McClelland JW (2016) Seasonal trophic linkages in Arctic marine invertebrates assessed via fatty acids and compound-specific stable isotopes. Ecosphere. https://doi.org/10.1002/ecs2.1429
Pickart RS, Schulze LM, Moore GWK, Charette MA, Arrigo KR, van Dijken G, Danielson SL (2013) Long-term trends of upwelling and impacts on primary productivity in the Alaskan Beaufort Sea. Deep Sea Res Part I 79:106–121
Piepenburg D (2005) Recent research on Arctic benthos: common notions need to be revised. Polar Biol 28(10):733–755
Pinchuk AI, Eisner LB (2017) Spatial heterogeneity in zooplankton summer distribution in the eastern Chukchi Sea in 2012–2013 as a result of large-scale interactions of water masses. Deep Sea Res Part II 135:27–39
Questel JM, Clarke C, Hopcroft RR (2013) Seasonal and interannual variation in the planktonic communities of the northeastern Chukchi Sea during the summer and early fall. Cont Shelf Res 67:23–41
Schlüter M, Rachor E (2001) Meroplankton distribution in the central Barents Sea in relation to local oceanographic patterns. Polar Biol 24(8):582
Shimada K, Kamoshida T, Itoh M et al (2006) Pacific Ocean inflow: influence on catastrophic reduction of sea ice cover in the Arctic Ocean. Geophys Res Lett. https://doi.org/10.1029/2005GL025624
Slattery PN, Oliver JS (1987) Barnacle settlement on Pleustes Panopla Tuberculata (Amphipoda) in the Chukchi Sea. J Crustac Biol 7(2):358–363
Søreide JE, Falk-Petersen S, Hegseth EN, Hop H, Carroll ML, Hobson KA, Blachowiak-Samolyk K (2008) Seasonal feeding strategies of Calanus in the high-Arctic Svalbard region. Deep Sea Res Part II 55(20):2225–2244
Springer AM, McRoy CP, Turco KR (1989) The paradox of pelagic food webs in the northern Bering Sea—II. Zooplankton communities. Cont Shelf Res 9(4):359–386
Stroeve J, Holland MM, Meier W, Scambos T, Serreze M (2007) Arctic sea ice decline: faster than forecast. Geophys Res Lett. https://doi.org/10.1029/2007GL029703
Turner JT, Levinsen H, Nielsen TG, Hansen BW (2001) Zooplankton feeding ecology: grazing on phytoplankton and predation on protozoans by copepod and barnacle nauplii in Disko Bay, West Greenland. Mar Ecol Prog Ser 221:209–219
Vargas CA, Narváez DA, Piñones A, Navarrete SA, Lagos NA (2006) River plume dynamic influences transport of barnacle larvae in the inner shelf off central Chile. J Mar Biol Assoc UK 86(5):1057–1065
Walsh JJ, McRoy CP, Coachman LK (1989) Carbon and nitrogen cycling within the Bering/Chukchi Seas: source regions for organic matter effecting AOU demands of the Arctic Ocean. Prog Oceanogr 22(4):277–359
Wang J, Cota GF, Comiso JC (2005) Phytoplankton in the Beaufort and Chukchi Seas: distribution, dynamics, and environmental forcing. Deep Sea Res Part II 52(24):3355–3368
Willis K, Cottier F, Kwasniewski S, Wold A, Falk-Petersen S (2006) The influence of advection on zooplankton community composition in an Arctic fjord (Kongsfjorden, Svalbard). J Mar Syst 61(1):39–54
Woodgate RA, Aagaard K, Weingartner TJ (2005) A year in the physical oceanography of the Chukchi Sea: moored measurements from autumn 1990–1991. Deep Sea Res Part II 52(24):3116–3149
Woodgate RA, Weingartner T, Lindsay R (2010) The 2007 Bering Strait oceanic heat flux and anomalous Arctic sea-ice retreat. Geophys Res Lett. https://doi.org/10.1029/2009GL041621
Woodgate RA, Stafford KM, Prahl FG (2015) A synthesis of year-round interdisciplinary mooring measurements in the Bering Strait (1990–2014) and the RUSALCA years (2004–2011). Oceanography 28(3):46–67
Zhang J, Ashjian C, Campbell R, Spitz YH, Steele M, Hill V (2015) The influence of sea ice and snow cover and nutrient availability on the formation of massive under-ice phytoplankton blooms in the Chukchi Sea. Deep Sea Res Part I 118:122–135
Acknowledgements
We thank Zhencheng Tao, Yongshan Zhang, Yongqiang Wang, Shaoqing Wang as well as the captain and crew of the R/V ‘XUELONG’ for their help with zooplankton sampling. Dr. Guang Yang offered helpful advice regarding data analysis, and Dr. Xiaoyu Wang at the Ocean University of China provided hydrographic data. This research is supported by National Natural Science Foundation of China (Nos. 41706217, 41406148) and Chinese Polar Environment Comprehensive Investigation and Assessment Programmes (No. CHINARE-2017-03-05).
Author information
Authors and Affiliations
Corresponding author
Ethics declarations
Conflicts of interest
The authors declare that they have no conflict of interest.
Rights and permissions
About this article
Cite this article
Xu, Z., Zhang, G. & Sun, S. Inter-annual variation of the summer zooplankton community in the Chukchi Sea: spatial heterogeneity during a decade of rapid ice decline. Polar Biol 41, 1827–1843 (2018). https://doi.org/10.1007/s00300-018-2324-3
Received:
Revised:
Accepted:
Published:
Issue Date:
DOI: https://doi.org/10.1007/s00300-018-2324-3