Abstract
Haloferax mediterranei, a poly(3-hydroxybutyrate-co-3-hydroxyvalerate) (PHBV) producing haloarchaeon, possesses four PHA synthase encoding genes, phaC, phaC1, phaC2, and phaC3. In the wild-type strain, except phaC, the other three genes are cryptic and not transcribed under PHA-accumulating conditions. The PhaC protein together with PhaE subunit forms the active PHA synthase and catalyzes PHBV polymerization. Previously, it was observed that the deletion of a gene named pps-like significantly enhanced PHBV accumulation probably resulted from the upregulation of pha cluster genes (phaR-phaP-phaE-phaC). The present study demonstrated the influence of pps-like gene deletion on the cryptic phaC genes. As revealed by qRT-PCR, the expression level of the three cryptic genes was upregulated in the ΔEPSΔpps-like geneΔphaC mutant. Sequential knockout of the cryptic phaC genes and fermentation experiments showed that PhaC1 followed by PhaC3 had the ability to synthesize PHBV in ΔEPSΔpps-like geneΔphaC mutant. Both PhaC1 and PhaC3 could complex with PhaE to form functionally active PHA synthase. However, the expression of phaC2 did not lead to PHBV synthesis. Moreover, PhaC, PhaC1, and PhaC3 exhibited distinct substrate specificity as the 3HV content in PHBV copolymers was different. The EMSA result showed that PPS-like protein might be a negative regulator of phaC1 gene by binding to its promoter region. Taken together, PhaC1 had the most pronounced effect on PHBV synthesis in ΔEPSΔpps-like geneΔphaC mutant and deletion of pps-like gene released the negative effect from phaC1 expression and thereby restored PHBV accumulating ability in ΔphaC mutant.
Key points
• Cryptic phaC genes were activated by pps-like gene deletion.
• PPS-like protein probably regulated phaC1 expression by binding to its promoter.
• Both PhaC1 and PhaC3 formed active PHA synthase with PhaE.
Similar content being viewed by others
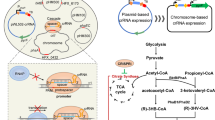
Avoid common mistakes on your manuscript.
Introduction
Nature has diverse ecosystem encompassing soda lakes, hot springs, deep oceans, deep sea hydrothermal vents, deserts, polar regions, etc. that exhibit extremities in one or more physico-chemical parameters. Microbiota inhabiting such environments includes varieties of extremophiles, such as halophiles, acidophiles, alkaliphiles, thermophiles, psychrophiles, piezophiles, xerotolerant, and radiotolerant (Orellana et al. 2018). Haloarchaea are the salt loving extremophiles belonging to Archaea domain. They are predominantly distributed in hypersaline environments such as soda lakes, natural brines, the Dead Sea, solar salterns, and rock salts (Fendrihan et al. 2006). Haloarchaea as an important microbial resource presents several advantages from an industrial point of view. For example, haloarchaeal enzymes are potential candidates for industrial applications under harsh conditions, where normal enzymes tend to be inactive or degrade. This is because haloarchaeal proteins are most likely to be salt-tolerant due to their adaptation to high salinity, resulting in high acidic amino acid content (Siroosi et al. 2014). Most haloarchaea are red and orange pigmented due to their ability to synthesize carotenoids (Zuo et al. 2018). Most importantly, haloarchaea are considered one of the most promising producers of polyhydroxyalkanoates (PHA) (Bhattacharyya et al. 2015). Until now, almost 17 haloarchaeal genera are known to produce PHA, including Halopiger, Natrialba, Halobiforma, Haloarcula, Haloferax, Halococcus, Halogranum, Halorubrum, Natrinema, and Natronorubrum (Hezayen et al. 2002; Legat et al. 2010; Zhao et al. 2015; Mitra et al. 2020).
PHA is a family of biodegradable and biocompatible polyester of hydroxyalkanoates synthesized by microbes as intracellular carbon and energy reserves under unbalanced conditions of nutrient limitation with excess carbon source (Han et al. 2007). Because of its good thermoplastic and mechanical properties, PHA is considered a potential alternative to the petroleum-based synthetic plastics. The characteristic properties of PHA are dependent on their monomer composition. For instance, poly(3-hydroxybutyrate) (PHB), the homopolymer of 3-hydroxybutyrate (3HB), is stiff and brittle in nature. It exhibits high degree of crystallization, early thermal degradation, and poor mechanical properties (Hong et al. 2013). Incorporation of 3-hydroxyvalerate (3HV) monomer in the PHB chain enhances their flexibility and mechanical properties (Han et al. 2015). Until now, more than 150 different types of monomers have been identified in PHA. Extensive research work has showed that PHA holds high promise as a biomaterial for developing medical implants for tissue engineering and wound healing (Han et al. 2017; Xue et al. 2018). Halophilic production of PHA is advantageous over non-halophiles because halophiles require less stringent sterile conditions as high salt concentrations prevent microbial contamination (Zhao et al. 2013). Moreover, haloarchaeal cells undergo lysis simply in tap water due to their high intracellular osmotic pressure (Shih et al. 2015). This eases the downstream processing. As a result, these factors contribute to reducing the overall PHA production cost.
Haloferax mediterranei is a model haloarchaea for studying archaeal physiology and metabolism. This strain accumulates poly(3-hydroxybutyrate-co-3-hydroxyvalerate) (PHBV) from various cheap unrelated carbon sources without using 3HV precursors (Hou et al. 2014). It efficiently utilizes several agro-industrial wastes, including vinasse, cheese whey, olive mill wastewater, and rice-based ethanol stillage, as low-cost substrates for PHBV production (Alsafadi and Al-Mashaqbeh 2017; Bhattacharyya et al. 2012; Bhattacharyya et al. 2014; Pais et al. 2016). Thus, it is the most prominent natural PHA producer among haloarchaea. The complete genome sequence of H. mediterranei was published in 2012 (Han et al. 2012). Subsequently, the key genes involved in PHBV metabolism and regulation have been identified and characterized in our lab (Cai et al. 2012; Cai et al. 2015; Han et al. 2013; Hou et al. 2013; Liu et al. 2015; Liu et al. 2016; Lu et al. 2008). The active PHA synthase in H. mediterranei is constituted of two subunits, PhaC and PhaE (Lu et al. 2008). Additionally, its genome sequence revealed three cryptic phaC genes, phaC1, phaC2, and phaC3 (Han et al. 2010). Except phaC2, phaC1 and phaC3 encoded functional proteins which formed active PHA synthases with PhaE subunit and led to the synthesis of PHBV in Haloarcula hispanica PHB-1 (PHA synthase gene deletion mutant). These findings have further paved the way for developing engineered H. mediterranei strain. For example, the knockout mutant of exopolysaccharide (EPS) biosynthesis gene cluster, ΔEPS, showed a 20% higher PHBV production than the wild-type stain (Zhao et al. 2013). EPS is synthesized by the wild-type H. mediterranei and excreted into the medium. Its accumulation hinders PHA synthesis by enhancing the culture viscosity and consuming carbon source. The culture of ΔEPS exhibited a reduced viscosity and thus increased the dissolved oxygen content in the medium and decreased the foaming propensity. Furthermore, more carbon source was channeled towards PHBV synthesis in ΔEPS compared with the wild-type strain (Zhao et al. 2013).
In our recent research, we serendipitously discovered two mutants H. mediterranei ΔEPSΔpps and ΔEPSΔpps-like gene with further improved PHBV accumulation ability while characterizing the key enzymes involved in the interconversion of pyruvate and phosphoenolpyruvate (PEP) (Chen et al. 2019). PEP synthetase (PPS) catalyzed pyruvate to PEP. Its deletion strain ΔEPSΔpps led to a 35.9% increase in PHA production which might be resulted from channeling more pyruvate to PHA synthesis. Notably, a novel protein that showed high homology with PPS, named PPS-like, was identified. However, unlike PPS, PPS-like protein did not participate in the conversion from pyruvate to PEP. Unexpectedly, its deletion increased PHBV production by 70.46%. Our RNA-seq data showed that several genes involved in the PHBV monomer supplying pathway (bktB, phaB1, phaB2, and phaJ) and PHBV biosynthesis (phaR, phaP, phaE, and phaC) were upregulated in ΔEPSΔpps-like gene. This finding is speculated to result in a significantly increased PHBV accumulation. However, the definite mechanism behind the enhanced PHBV production remained to be determined.
The present paper is a continuation of our previous research work and attempts to determine the impact of pps-like gene deletion on the cryptic PHA synthase genes of H. mediterranei (Chen et al. 2019). We designed and developed several PHA synthase mutant strains based on H. mediterranei ΔEPS Δpps-like gene and checked its corresponding effects on PHBV synthesis. Overall, this study is an endeavor to elucidate the reasons behind improved PHBV synthesis upon pps-like gene deletion in H. mediterranei.
Materials and methods
Strains and growth conditions
All the bacterial and haloarchaeal strains used in this study are summarized in Table 1. The two Escherichia coli strains, JM109 and JM110, were used for constructing plasmids and eliminating DNA methylation of plasmids, respectively. They were grown in Luria-Bertani medium at 37 °C. When needed, 100 μg/mL ampicillin was added to the medium. H. mediterranei CGMCC 1.2087 (wild type strain) was deposited in the China General Microbiological Culture Collection Center (CGMCC). The derived strains of H. mediterranei used in this study were pyrF deletion mutants, and thus 50-μg/mL uracil was added to their culture media. They were cultivated in AS-168 medium (Sambrook et al. 1989) at 37 °C and used as seed cultures after entering late exponential phase. The seed culture was then inoculated into PHA production medium (Zhao et al. 2013) containing 10 g/L glucose with a 5% (vol/vol) inoculum for PHA synthesis. The fermentation was carried out at 37 °C until the glucose in the media was completely consumed.
Plasmid construction and genetic manipulation of H. mediterranei
The plasmids and primers used for gene knockout are listed in Table 1 and Table 2, respectively. The upstream and downstream fragments of the three phaC genes were amplified by using relevant primer pairs NF/NR and CF/CR from the genomic DNA of H. mediterranei via PCR, respectively. The three knockout plasmids, pHFX-phaC, pHFX-phaC1, and pHFX-phaC3, were constructed by inserting the two fragments of the target gene into the suicide plasmid pHFX (Liu et al. 2011). The plasmids were first constructed in E. coli JM109 and were confirmed by sequencing. Then, the plasmids extracted from E. coli JM110 were transformed into H. mediterranei by the polyethylene glycol-mediated method (Cline et al. 1989). The correct gene knockout mutants were screened by PCR verification as previously described (Liu et al. 2011).
RNA isolation and qRT-PCR
H. mediterranei cells were grown in PHA production medium in tubes to exponential phase and stationary phase and were subsequently harvested for RNA isolation. Total RNA extraction was carried out with the TRIzol® Reagent (Invitrogen, USA) as instructed by the manufacturer. cDNA was generated from the DNA-free RNA samples by using random hexamers and the MLV Reverse Transcriptase (Promega, USA). The fluorogenic quantitative PCR was performed in a ViiA™ 7 Real-Time PCR System (Applied Biosystems, USA) as described previously (Chen et al. 2019). Each sample was replicated in triplicates and normalized using 7S rRNA gene as an endogenous control. The fold change of gene expression was analyzed according to the method developed by Schmittgen et al. (2008). The primers used are listed in Table 2.
Measurements of cell growth and determination of residual glucose concentration
Diphenylamine colorimetric method was used to measure the growth of H. mediterranei mutants by using Beckman Coulter DU800 spectrophotometer (Jersey City, NJ, USA), as described previously (Hou et al. 2015). The cell growth curve was plotted by using OD595 per mL culture against time. The residue glucose concentration in the culture was monitored by using SBA-40C biosensor analyzer (Shandong, China).
Gas chromatography
Harvested cells of H. mediterranei were lyophilized overnight by using TF-FD-12S freeze dryer (Shanghai, China). About 50-mg lyophilized cells were used for methanolysis reaction in 2-mL chloroform and 2-mL methanol containing 3% (vol/vol) sulfuric acid at 100 °C for 4 h. After stratification using distilled water, the constituents in the organic phase were analyzed by GC-6820 (Agilent, USA) as previously described (Han et al. 2007). In the GC analysis, benzoic acid was used as the internal standard for quantitative calculation of 3HB and 3HV. The relative mass correction factors for 3HB and 3HV were obtained from reference material PHBV and used for the quantitative analysis of monomer compositions. PHBV content was calculated as follows: (mass of PHBV/original lyophilized cell mass) × 100%. The 3HV molar content in PHA was calculated as the ratio of 3HV mole / (3HV mole + 3HB mole) (as mol%).
Transmission electron microscopy
The H. mediterranei strains of ΔEPSΔpps-like gene, ΔEPSΔpps-like geneΔphaCΔphaC1, ΔEPSΔpps-like geneΔphaCΔphaC3, and ΔEPSΔpps-like geneΔphaCΔphaC1ΔphaC3 were grown in PHA production medium for 168 h and subsequently harvested for TEM analysis. TEM sample preparation was performed as previously described (Cai et al. 2012). TEM images of PHA granules were acquired on JEM-1400 transmission electron microscope (JEOL, Japan).
Electrophoretic mobility shift assay
The double-stranded DNA fragment (176 bp) of phaC1 promoter was amplified by PCR using the primer pair of phaC1-Pro-F/phaC1-Pro-R (Table 2). The purified PCR product as the probe for EMSA was labeled with biotin by using Chemiluminescent Biotin-Labeled Nucleic Acid Detection Kit (Beyotime, China) as the manufacturer’s instruction. PPS-like protein was expressed and purified from Haloferax volcanii H1424 as previously described (Chen et al. 2019). The EMSA was performed using Chemiluminescent EMSA Kit (Beyotime, China) with minor modifications. Briefly, PPS-like protein (0–160 pmol) were incubated with 1 fmol DNA probe in a 20 μL reaction mixture contained 1 × EMSA buffer, 1 μg/μL poly dI-dC, 20 mM Tris-HCl (pH 8.0), 2 M NaCl at 25 °C for 30 min. Then, the reaction samples were immediately loaded on a non-denaturing 8% polyacrylamide gel with 1 μL 40% sucrose solution and 0.2 μL bromophenol blue. The electrophoresis was run in 0.5 × Tris-borate-EDTA buffer at 250 V for 25 min. Subsequently, the DNA-protein complex and free probes were transferred onto a Biodyne nylon membrane (Pall, USA) by using Trans-Blot (Bio-Rad, USA). The transfer was run in 0.5 × Tris-borate-EDTA buffer at 4 °C for 30 min at electricity of 380 mA. The membrane with probes was cross-linked using Gene Linker UV Chamber (Bio-Rad, American) at C-3 gear. The biotin-labeled probes were detected by Chemiluminescent Nucleic Acid Detection Module Kit (Thermo Fisher Scientific, USA) and photographed using Tanon 5200 Multi Chemiluminescent Imaging System (Tanon, China).
Sequence analysis
An online webserver was used for predicting DNA-binding proteins (http://crdd.osdd.net/raghava/dnabinder/index.html). The parameter of SVM (support vector machine) score greater than zero indicated that the predicted protein is a DNA-binding protein.
Statistical analysis
All data were analyzed using GraphPad Prism software and presented as mean ± standard deviation (SD) of the three independent replicates. Statistical significance between groups were performed using Student’s t test at three significance levels (*p < 0.05, **p < 0.01, and ***p < 0.01).
Results
pps-like gene deletion upregulates the transcription of cryptic phaCs
In H. mediterranei, the active PHA synthase encoding genes, phaE and phaC, form a PHA gene cluster with phaR and phaP on the megaplasmid pHM300 (Fig. 1a). In addition, phaC1 and phaC2/phaC3 are located on its chromosome and the megaplasmid pHM500, respectively. Our previous work found that phaC1, phaC2, and phaC3 were not transcribed in the wild-type H. mediterranei strain (Han et al. 2010). Our recent published RNA-seq data showed that the three cryptic phaC genes were upregulated by 5-, 2-, and 3-fold, respectively, in H. mediterranei ΔEPSΔpps-like gene compared with ΔEPS (Chen et al. 2019). However, the number of fragments per kilobase of transcript per million mapped reads (FPKM) of phaC1, phaC2, and phaC3 was 27, 21, and 187, respectively, which was much lower than the FPKM (1250) of phaC gene. In order to precisely compare the expression level of the three phaC genes in ΔEPSΔpps-like gene and ΔEPS, we carried out qRT-PCR with the RNA samples from both exponential phase and stationary phase cultures by using the specific primers (Fig. 1a and Table 2).
Expression of three cryptic phaC genes in Haloferax mediterranei mutants. a Genetic organization of four phaC genes in H. mediterranei. hp hypothetical protein, DBD DNA binding domain. The location of primers used for qRT-PCR is indicated by arrows. qRT-PCR analysis of three cryptic phaC genes in pps-like gene or phaC gene knockouts at exponential phase (b) and stationary phase (c). All the data are represented as mean ± standard deviations from three independent experiments. **p < 0.01, ***p < 0.01
The qRT-PCR results were basically consistent with the RNA-seq transcription tendency (Fig. 1 b and c). The transcription level of phaC1 was upregulated by 3- and 22-fold at exponential and stationary phase, respectively, in ΔEPSΔpps-like gene compared with ΔEPS. For phaC2 and phaC3, no significant change was observed at exponential phase, whereas the expression level at stationary phase was 5-fold and 3-fold higher, respectively, in the ΔEPSΔpps-like gene. Our RNA-seq found that the pps-like gene deletion increased the functional PHA synthase expression level in H. mediterranei. Overexpression of PHA synthase could influence the expression level of other PHA synthesis genes, including other phaCs, phaF, and phaI (Kim et al. 2006; Quelas et al. 2013). In order to exclude the effect of phaC overexpression on the three cryptic phaC genes’ expression, we knockout the phaC gene in ΔEPS and ΔEPSΔpps-like gene. The expression level of phaC1, phaC2, and phaC3 in ΔEPSΔphaC was similar to ΔEPS at two growth phases (Fig. 1 b and c). It indicated that the transcription level of the three phaC genes was negligible in ΔEPSΔphaC as in ΔEPS. However, deletion of pps-like gene in ΔEPSΔphaC increased the expression of phaC1, phaC2, and phaC3 more obviously compared with deletion of pps-like gene in ΔEPS. The transcription level of phaC1 was almost 10- and 34-fold higher at exponential and stationary phase in ΔEPSΔpps-like geneΔphaC, respectively. The expression of phaC2 showed almost 3- and 6-fold increase at exponential and stationary phase in ΔEPSΔpps-like geneΔphaC, respectively. In the case of phaC3, its expression underwent almost 6-fold increase at stationary phase in ΔEPSΔpps-like geneΔphaC. In total, deletion of the pps-like gene activated the expression of the cryptic phaC genes in H. mediterranei, which supported our previous findings (Chen et al. 2019). Moreover, deletion of both phaC and pps-like genes led to a more prominent upregulation of the expression level of phaC1 than phaC2 and phaC3 genes. This indicated that phaC deletion had a positive effect on cryptic phaC gene expression when pps-like gene was deleted.
pps-like and phaC knockout strain accumulates PHBV
In our subsequent study, we aimed to investigate whether the expressed cryptic PhaC proteins could form a functional PHA synthase with PhaE protein in Δpps-like gene strain. We tested the PHA accumulation ability of four mutant strains (ΔEPS, ΔEPSΔphaC, ΔEPSΔpps-like gene, ΔEPSΔpps-like geneΔphaC) by culturing them in PHA production media for 132 h. During the fermentation process, culture samples were taken every 12 h to measure cell growth and glucose consumption (Fig. 2a). Obviously, ΔEPSΔphaC and ΔEPSΔpps-like geneΔphaC grew slowly compared with ΔEPS and ΔEPSΔpps-like gene, respectively. Among the four tested strains, the growth rate decreased in the following order: ΔEPSΔpps-like gene>ΔEPS>ΔEPSΔpps-like geneΔphaC>ΔEPSΔphaC. The residual glucose concentration in the culture supernate showed an opposite trend with respect to the cell growth rate. These results showed that phaC deletion slowed down glucose consumption and simultaneously had a negative effect on cell growth in H. mediterranei. However, pps-like gene deletion increased glucose consumption and accelerated cell growth in ΔEPS and ΔEPSΔphaC.
Effects of pps-like or phaC knockout on cell growth, glucose consumption, and PHBV synthesis in H. mediterranei. a Time course of cell growth and glucose consumption. Green curves, glucose consumption; red curves, cell growth; closed circles, ΔEPS; open circles, ΔEPSΔphaC; closed triangles, ΔEPSΔpps-like gene; open triangles, ΔEPSΔpps-like geneΔphaC. b Optical images of cell cultures in PHA fermentation medium at 132 h. c GC analysis of PHBV accumulation in ΔEPS, ΔEPSΔphaC, ΔEPSΔpps-like geneΔphaC, and ΔEPSΔpps-like gene
The samples at 132 h were used for PHA analysis. With the increase in the amount of intracellular PHA, the turbidity of H. mediterranei culture became higher. As shown in Fig. 2b, the cultures of ΔEPS and ΔEPSΔpps-like gene showed greater turbidity compared with ΔEPSΔpps-like geneΔphaC and ΔEPSΔphaC. Notably, the culture of ΔEPSΔphaC was almost transparent orange color, indicating no PHA deposited in the cells. The PHA accumulation results determined by GC analysis are shown in Table 3 and Fig. 2c. ΔEPS and ΔEPSΔpps-like gene could accumulate 48% and 54% (wt) PHBV, respectively. The deletion of phaC in ΔEPS completely abolished its PHA accumulation ability, whereas its deletion in ΔEPSΔpps-like gene still resulted in a PHBV accumulation of 5% (wt). It implied that PhaC1 and/or PhaC3 could form functional PHA synthase with the PhaE subunit and catalyzed PHBV synthesis in the pps-like gene deletion mutant.
Both PhaC1 and PhaC3 form functional PHA synthase with PhaE subunit in pps-like deletion mutant
We next wanted to know which of the three cryptic PhaCs polymerized with PhaE to accumulate PHBV in ΔEPSΔpps-like geneΔphaC. Thus, phaC1, phaC3, or both were further knockout in ΔEPSΔpps-like geneΔphaC. The parent stain (ΔEPSΔpps-like geneΔphaC) and obtained mutants (ΔEPSΔpps-like geneΔphaCΔphaC1, ΔEPSΔpps-like geneΔphaCΔphaC3, and ΔEPSΔpps-like geneΔphaCΔphaC1ΔphaC3) were then used for PHA fermentation and GC analysis. The fermentation process lasted for 228 h until the glucose in all cultures was consumed completely. The slow glucose consumption might be related to a small amount of or even no PHA synthesis (Table 4). Deletion of the phaC1 gene reduced the PHBV content from 4 to 0.43% (wt). On the other hand, the deletion of the phaC3 gene slightly reduced the PHBV content to 3.44% (wt). This suggested that PhaC1 had a pronounced effect on PHBV synthesis in the ΔEPSΔpps-like geneΔphaC strain, compared with PhaC3. However, the PHA yield in ΔEPSΔpps-like geneΔphaCΔphaC1 and ΔEPSΔpps-like geneΔphaCΔphaC3 mutants was very low compared with ΔEPSΔpps-like gene mutant. It also implied that the expression level of phaC1 and phaC3 caused by pps-like gene deletion mutant was quite low compared with the phaC gene in its original strain. The knockout of both phaC1 and phaC3 genes in ΔEPSΔpps-like geneΔphaC completely abolished its PHA synthesis. Thus, PhaC2 did not participate in PHBV synthesis. The 3HV content was significantly enhanced in the phaC1 or phaC3 deletion mutants compared with the ΔEPSΔpps-like strain. The expression of phaC1 and phaC3 genes resulted into the 3HV content of 48.63 and 35.11 mol%, respectively, whereas phaC gene expression led to a 3HV content of 10.55 mol%. This might be due to the different substrate specificities of PhaC1, PhaC3, and PhaC. The fermentation results were further verified by visualizing the PHA granules by TEM imaging (Fig. 3). Few PHA granules were observed in the strain ΔEPSΔpps-like geneΔphaCΔphaC1. The number of PHA granules was more and appeared bigger in size in ΔEPSΔpps-like geneΔphaCΔphaC3. No PHA granule was observed in ΔEPSΔpps-like geneΔphaCΔphaC1ΔphaC3. Despite the deletion of phaC gene, PhaC1 and PhaC3 formed PHA granules after the pps-like gene knockout. Thus, PhaC1 and PhaC3 formed an active PHA synthase complex with PhaE subunit in the Δpps-like gene mutant. However, PhaC2 did not participate in PHA granule formation. This indicated that PhaC2 was incapable of forming functional PHA synthase due to its truncated C terminus. This result was consistent with our previous report that PhaC2 could not form functional PHA synthase with PhaE protein in H. hispanica (Han et al. 2010). Overall, in agreement with the previous two results and the fermentation results, both PhaC1 and PhaC3 could complex with PhaE subunit to form active PHA synthase and accumulate PHBV in ΔEPSΔpps-like geneΔphaC. Especially, PhaC1 demonstrated a significant effect on PHBV synthesis.
TEM images of pps-like and phaCs deletion H. mediterranei mutants grown in PHA fermentation medium for 168 h. a ΔEPSΔpps-like gene; b ΔEPSΔpps-like geneΔphaCΔphaC1; c ΔEPSΔpps-like geneΔphaCΔphaC1ΔphaC3; d ΔEPSΔpps-like geneΔphaCΔphaC3. PHA granules observed in (b) and (c) are indicated by yellow arrows. Scar bars are 0.5 μm
PPS-like protein binds to the phaC1 promoter in vitro
The detailed mechanism behind the activation of the three cryptic phaC genes in pps-like gene deleted H. mediterranei remained to be clarified. PPS-like protein has no PPS enzyme activity although it shows high homology with PPS family proteins (Chen et al. 2019). Then, we tentatively speculated that PPS-like protein might regulate its target genes by binding their promoters and performed a prediction by using an online webserver (http://crdd.osdd.net/raghava/dnabinder/index.html). The SVM score of PPS-like protein is 0.89 and thus probably possessed DNA sequence binding ability. We chose phaC1 gene as the target gene to test the DNA-binding capability of PPS-like protein due to the follow reasons. Our qRT-PCR and fermentation results revealed that among the three cryptic phaC genes, phaC1 was upregulated most prominently and played the most important role in PHA polymerization in pps-like gene and phaC gene deletion mutants. In addition, phaC1 and pps-like gene are closely linked and separated by only one small gene with unknown function on the chromosome (Fig. 1a). This indicated that the interaction between PPS-like and the promoter sequence of phaC1 has a high probability. The 176 bp promoter sequence of phaC1 embracing its core elements, BRE and TATA box, was amplified (Fig. 4a and Table 2) to test whether the phaC1 promoter could be recognized by PPS-like protein overexpressed and purified from Haloferax volcanii. Their interaction was determined by electrophoretic mobility shift assay (EMSA) (Fig. 4b). As we predicted, PPS-like protein could efficiently bind to the phaC1 promoter sequence deduced from a clear binding band observed by EMSA analysis (Fig. 4b). At a fixed DNA concentration of 1 fmol, the increasing concentration of PPS-like protein resulted in the formation of more protein-DNA complex. PPS-like could bind to phaC1 promoter sequence at the protein concentration of 50 pmol. As the protein concentration increased to 160 pmol, the formed PPS-like DNA complex was significantly intensified (Fig. 4b). This result might help explain the upregulation of phaC1 gene expression after pps-like gene deletion, as PPS-like protein repressed phaC1 expression by binding to its promoter sequence.
EMSA analysis of the interaction between PPS-like protein and the double-stranded DNA fragment of phaC1 promoter. a Nucleotide sequences of phaC1 promoter used for EMSA. The length of the DNA probe was 176 bp. The predicted BRE and TATA box were shaded and double underlined, respectively. The start code of phaC1 was boxed. The primers for PCR were in italic. b EMSA of PPS-like protein and phaC1 promoter. The amount of DNA probe (1 fmol) and proteins (0–160 pmol) in each lane was indicated. The DNA protein complex was indicated by arrows. FP free probes
Discussion
The present study showed that the deletion of pps-like gene in H. mediterranei activated the expression of three cryptic phaC genes, especially phaC1, although their absolute transcription was low. PPS-like protein probably negatively regulated the expression level of phaC1 by binding to its promoter region. It was also found that PhaC1 and PhaC3 could form functional PHA synthases with PhaE and accumulated PHBV in ΔEPSΔpps-like geneΔphaC. However, the expression of phaC2 alone completely abolished the PHBV synthesis capability. The 3HV content in the PHBV synthesized by the phaC mutants were significantly higher compared with the ΔEPSΔpps-like strain. PhaC1 contributed to the highest 3HV mol%, followed by PhaC3 and finally PhaC.
H. mediterranei wild-type strain has four phaC encoding genes. Only the phaC gene in the pha cluster is expressed, and the other three phaC genes are cryptic under PHA-accumulating condition. The presence of multiple PHA synthase genes is a common phenomenon among PHA-accumulating microorganisms. For example, Pseudomonas species usually possess at least two PHA synthase encoding genes. Pseudomonas mendocina genome encoded two PHA synthase genes (Hein et al. 2002). Both the PHA synthase genes were functional and conferred PHA synthesis when expressed individually in PHA-negative mutants. However, PhaC1 was the major PHA synthase and accumulated higher PHA content with fatty acid as a sole carbon source, while PhaC2 accumulated a higher concentration of PHA using gluconate under storage conditions. Pseudomonas oleovorans and Pseudomonas putida strains were reported to contain even more than two PHA synthase genes that influenced the PHA monomer composition (Matsusaki et al. 1998). Ralstonia eutropha has two PHA synthase encoding genes, phaC1 and phaC2 (Peplinski et al. 2010). phaC1 gene was constitutively expressed and encoded the major PHA synthase enzyme. phaC2 was not transcribed under all conditions except during storage conditions using gluconate as sole carbon source. Moreover, deletion of phaC1 gene inactivated phaC2 gene. The PHA gene locus in Burkholderia sp. included two PHA synthase genes, each of them encoding functional enzyme, probably with distinct substrate specificity (de Andrade Rodrigues et al. 2000; Hang et al. 2002). The genome of Neptunomonas concharum JCM17730 contained two class I PHA synthases, PhaC1 and PhaC2, and one class III PHA synthase, PhaEC (Pu et al. 2020). Heterologous expression of the individual PHA synthase genes in E. coli showed that the three phaC genes exhibited different catalytic activities at different cultivation temperatures (Pu et al. 2020). PhaC1 presented the highest PHA synthesis ability, followed by PhaEC and PhaC2. Several Halomonas species have been reported to encode two different phaC genes. The two PhaC proteins from Halomonas sp. TD01 showed 78% and 73% identity with the two PhaCs from Halomonas elongata DSM2581, respectively (Cai et al. 2011). The heterologous expression of phaC1He from H. elongate DSM 2581 accumulated PHA in E. coli (Ilham et al. 2014). However, phaC2He expression in E. coli did not synthesize PHA. Furthermore, coexpression of phaC1 and phaC2 genes from Halomonas sp. O-1 resulted in an effect similar to phaC1 expression alone in E. coli (Ilham et al. 2014). This indicated that Halomonas phaC2 was non-functional. A total of 5 phaC genes were annotated in the genome of Bradyrhizobium japonicus UDSA 110 (Quelas et al. 2013).
It has been observed that PHA synthase gene expression influenced the expression level of other PHA biosynthesis genes, positively or negatively. For instance, phaC2 overexpression in P. putida elevated the transcription level of its two PHA granule-associated genes, phaF and phaI (Kim et al. 2006). B. japonicum USDA 110 genome had five paralogs of PHA synthase genes belonging to different classes (Quelas et al. 2013). PhaC1 was similar to rhizobial PHA synthase and belonged to class I type. PhaC2 was unclassified and related to PHA synthase from betaproteobacteria. PhaC3, PhaC4, and PhaC5 belonged to class I, class III, and class IV, respectively. Among them, phaC1 and phaC2 were significantly expressed, but phaC3, phaC4, and phaC5 expression level was very low. phaC1 was the main PHA synthase as its deletion mutant could not synthesize PHA. Interestingly, ΔphaC2 mutant increased phaC3 transcript and synthesized more PHA than wild type, suggesting that phaC2 negatively regulated PHA synthesis. Similarly, in the case of Rhodospirillum rubrum possessing three phaC genes, PhaC2 was the major PHA synthase. However, deletion of phaC1 gene elevated the PHA production level, indicating that PhaC1 exerted inhibitory effect on PHA synthesis (Jin and Nikolau 2012). In this study performed in H. mediterranei, prior to the deletion of the cryptic genes in ΔEPSΔpps-like gene mutant, phaC gene was knockout. This strategy helped to determine the sole effect of the cryptic genes on PHBV synthesis upon pps-like gene deletion. Our results showed that besides the regulation of phaC1 imposed by PPS-like protein, it was probable that overexpression of phaEC gene caused by the deletion of pps-like gene in H. mediterranei had influenced the expression of phaC1, phaC2, and phaC3 genes (Fig. 1 b and c). Moreover, the fact that the three PhaC proteins, PhaC, PhaC1, and PhaC3 led to incorporation of different molar fractions of 3HV showed their distinct substrate specificity. Thus, engineering of these genes can possibly produce different PHBV materials with tailor-made 3HV content.
The expression of phaC is often regulated by DNA binding regulatory proteins. In P. oleovorans, PhaF negatively regulated the expression of phaC1 by binding to the promoter of phaC1 (Prieto et al. 1999). Additionally, Pseudomonas sp. encodes a PhaD protein which is a TetR-like transcriptional regulator. PhaD could bind the promoter of phaC1, which directed the transcription of phaC1ZC2D operon (de Eugenio et al. 2010). In B. japonicum USDA 110, expression of phaC5 and phaC3 was elevated when phaR gene, encoding a DNA binding PHA granule associated protein, was deleted (Nishihata et al. 2018). In Azotobacter vinelandii, PhbR protein is a transcriptional activator of phbBAC operon (Peralta-Gil et al. 2002). However, in the case of PPS-like mediated regulation, we had two important findings. First, pps-like gene knockout led to upregulated phaEC expression and enhanced PHA accumulation with no disorder in granule formation. Second, PPS-like protein repressed phaC1 expression by binding to its promoter region, and hence its deletion upregulated phaC1 gene expression. This type of regulation phenomenon by PPS-like protein is identified in PHA accumulating microorganisms for the first time. However, the specific binding sites of phaC1 promoter by PPS-like protein need to be further determined in our future study.
PPS belongs to the PEP-utilizing family of protein. The function of PPS is quite diverse among various microorganisms. It is normally involved in the gluconeogenic direction of carbohydrate metabolism and catalyzes the conversion of pyruvate to PEP (Niersbach et al. 1992). However, there are reports that PPS has a bidirectional activity and participates in the glycolytic direction, i.e., conversion of PEP to pyruvate in microorganisms like Pyrococcus furiosus (Sakuraba et al. 2001). In Thermococcus kodakarensis, PPS enzyme played a vital role in the conversion of PEP to pyruvate as the strain failed to grow on maltooligosaccharides upon the deletion of pps gene (Imanaka et al. 2006). Pyruvate phosphate dikinase (PPDK) has a similar structure and function with PPS and also belongs to PEP-utilizing family. In thermophilic archaeon Thermoproteus tenax, PPDK showed a bidirectional activity in PEP/pyruvate interconversion with preference for PEP conversion to pyruvate (Tjaden et al. 2006). In Microbispora rosea, PPDK is involved in the gluconeogenic pathway, catalyzing the formation of PEP from pyruvate (Eisaki et al. 1999). PPDK is an important rate-limiting enzyme in C4 photosynthetic pathway in plants. It is involved in diverse processes in plants, including abiotic stress tolerance, early seedling growth, seed development, conversion of NADH to NADPH, maintenance of pH and replenishing citric acid cycle intermediates for amino acid synthesis, nitrogen assimilation and fatty acid synthesis (Yadav et al. 2020). A very close structural homolog to PPS and PPDK protein is rifampin phosphotransferases (RPH). It is an antibiotic resistance protein, widespread among pathogenic bacteria that confer resistance to rifamycin by phosphorylation (Qi et al. 2016). Similarly, multiple sequence alignment and phylogenetic tree analysis showed that PPS-like protein was closely related to both PPS and PPDK proteins (Chen et al. 2019). However, it neither possessed PPS nor PPDK function. Rather, our present study revealed its regulatory function on phaC1 gene. Thus, even though all these proteins showed high homology, they have evolved diverse functions. Taken together, molecular characterization of PPS-like protein and its involvement in PHBV synthesis enhancement has provided a new insight on both the PHA biosynthesis in haloarchaea and the diversity of PPS family protein functions.
References
Alsafadi D, Al-Mashaqbeh O (2017) A one-stage cultivation process for the production of poly-3-(hydroxybutyrate-co-hydroxyvalerate) from olive mill wastewater by Haloferax mediterranei. New Biotechnol 34:47–53. https://doi.org/10.1016/j.nbt.2016.05.003
Bhattacharyya A, Pramanik A, Maji SK, Haldar S, Mukhopadhyay UK, Mukherjee J (2012) Utilization of vinasse for production of poly-3-(hydroxybutyrate-co-hydroxyvalerate) by Haloferax mediterranei. AMB Express 2(1):34. https://doi.org/10.1186/2191-0855-2-34
Bhattacharyya A, Saha J, Haldar S, Bhowmic A, Mukhopadhyay UK, Mukherjee J (2014) Production of poly-3-(hydroxybutyrate-co-hydroxyvalerate) by Haloferax mediterranei using rice-based ethanol stillage with simultaneous recovery and re-use of medium salts. Extremophiles 18(2):463–470. https://doi.org/10.1007/s11274-015-1823-4
Bhattacharyya A, Jana K, Haldar S, Bhowmic A, Mukhopadhyay UK, De S, Mukherjee J (2015) Integration of poly-3-(hydroxybutyrate-co-hydroxyvalerate) production by Haloferax mediterranei through utilization of stillage from rice-based ethanol manufacture in India and its techno-economic analysis. World J Microbiol Biotechnol 31(5):717–727. https://doi.org/10.1007/s11274-015-1823-4
Cai L, Tan D, Aibaidula G, Dong XR, Chen JC, Tian WD, Chen GQ (2011) Comparative genomics study of polyhydroxyalkanoates (PHA) and ectoine relevant genes from Halomonas sp. TD01 revealed extensive horizontal gene transfer events and co-evolutionary relationships. Microb Cell Factories 10:88. https://doi.org/10.1186/1475-2859-10-88
Cai S, Cai L, Liu H, Liu X, Han J, Zhou J, Xiang H (2012) Identification of the haloarchaeal phasin (PhaP) that functions in polyhydroxyalkanoate accumulation and granule formation in Haloferax mediterranei. Appl Environ Microbiol 78(6):1946–1952. https://doi.org/10.1128/AEM.07114-11
Cai S, Cai L, Zhao D, Liu G, Han J, Zhou J, Xiang H (2015) A novel DNA-binding protein, PhaR, plays a central role in the regulation of polyhydroxyalkanoate accumulation and granule formation in the haloarchaeon Haloferax mediterranei. Appl Environ Microbiol 81(1):373–385. https://doi.org/10.1128/AEM.02878-14
Chen J, Mitra R, Zhang S, Zuo Z, Lin L, Zhao D, Xiang H, Han J (2019) Unusual phosphoenolpyruvate (PEP) synthetase-like protein crucial to enhancement of polyhydroxyalkanoate accumulation in Haloferax mediterranei revealed by dissection of PEP-pyruvate interconversion mechanism. Appl Environ Microbiol 85(19). https://doi.org/10.1128/AEM.00984-19
Cline SW, Lam WL, Charlebois RL, Schalkwyk LC, Doolittle WF (1989) Transformation methods for halophilic archaebacteria. Can J Microbiol 35(1):148–152. https://doi.org/10.1139/m89-022
de Andrade Rodrigues MF, Vicente EJ, Steinbuchel A (2000) Studies on polyhydroxyalkanoate (PHA) accumulation in a PHA synthase I-negative mutant of Burkholderia cepacia generated by homogenotization. FEMS Microbiol Lett 193(1):179–185. https://doi.org/10.1111/j.1574-6968.2000.tb09421.x
de Eugenio LI, Galan B, Escapa IF, Maestro B, Sanz JM, Garcia JL, Prieto MA (2010) The PhaD regulator controls the simultaneous expression of the pha genes involved in polyhydroxyalkanoate metabolism and turnover in Pseudomonas putida KT2442. Environ Microbiol 12(6):1591–1603. https://doi.org/10.1111/j.1462-2920.2010.02199.x
Eisaki N, Tatsumi H, Murakami S, Horiuchi T (1999) Pyruvate phosphate dikinase from a thermophilic actinomyces Microbispora rosea subsp. aerata: purification, characterization and molecular cloning of the gene. Biochim Biophys Acta 1431(2):363–373. https://doi.org/10.1016/s0167-4838(99)00057-6
Fendrihan S, Legat A, Pfaffenhuemer M, Gruber C, Weidler G, Gerbl F, Stan-Lotter H (2006) Extremely halophilic archaea and the issue of long-term microbial survival. Rev Environ Sci Biotechnol 5(2–3):203–218. https://doi.org/10.1007/s11157-006-0007-y
Han J, Lu Q, Zhou L, Zhou J, Xiang H (2007) Molecular characterization of the phaECHm genes, required for biosynthesis of poly(3-hydroxybutyrate) in the extremely halophilic archaeon Haloarcula marismortui. Appl Environ Microbiol 73(19):6058–6065. https://doi.org/10.1128/AEM.00953-07
Han J, Li M, Hou J, Wu L, Zhou J, Xiang H (2010) Comparison of four phaC genes from Haloferax mediterranei and their function in different PHBV copolymer biosyntheses in Haloarcula hispanica. Saline Syst 6:9. https://doi.org/10.1186/1746-1448-6-9
Han J, Zhang F, Hou J, Liu XQ, Li M, Liu HL, Cai L, Zhang B, Chen YP, Zhou J, Hu SN, Xiang H (2012) Complete genome sequence of the metabolically versatile halophilic archaeon Haloferax mediterranei, a poly(3-hydroxybutyrate-co-3-hydroxyvalerate) producer. J Bacteriol 194(16):4463–4464. https://doi.org/10.1128/JB.00880-12
Han J, Hou J, Zhang F, Ai G, Li M, Cai S, Liu H, Wang L, Wang Z, Zhang S, Cai L, Zhao D, Zhou J, Xiang H (2013) Multiple propionyl coenzyme A-supplying pathways for production of the bioplastic poly(3-hydroxybutyrate-co-3-hydroxyvalerate) in Haloferax mediterranei. Appl Environ Microbiol 79(9):2922–2931. https://doi.org/10.1128/AEM.03915-12
Han J, Wu LP, Hou J, Zhao DH, Xiang H (2015) Biosynthesis, characterization, and hemostasis potential of tailor-made poly(3-hydroxybutyrate-co-3-hydroxyvalerate) produced by Haloferax mediterranei. Biomacromolecules 16(2):578–588. https://doi.org/10.1021/bm5016267
Han J, Wu LP, Liu XB, Hou J, Zhao LL, Chen JY, Zhao DH, Xiang H (2017) Biodegradation and biocompatibility of haloarchaea-produced poly(3-hydroxybutyrate-co-3-hydroxyvalerate) copolymers. Biomaterials 139:172–186. https://doi.org/10.1016/j.biomaterials.2017.06.006
Hang X, Zhang G, Wang G, Zhao X, Chen GQ (2002) PCR cloning of polyhydroxyalkanoate biosynthesis genes from Burkholderia caryophylli and their functional expression in recombinant Escherichia coli. FEMS Microbiol Lett 210(1):49–54. https://doi.org/10.1111/j.1574-6968.2002.tb11158.x
Hein S, Paletta JR, Steinbuchel A (2002) Cloning, characterization and comparison of the Pseudomonas mendocina polyhydroxyalkanoate synthases PhaC1 and PhaC2. Appl Microbiol Biotechnol 58(2):229–236. https://doi.org/10.1007/s00253-001-0863-x
Hezayen FF, Steinbüchel A, Rehm BHA (2002) Biochemical and enzymological properties of the polyhydroxybutyrate synthase from the extremely halophilic archaeon strain 56. Arch Biochem Biophys 403(2):284–291. https://doi.org/10.1016/S0003-9861(02)00234-5
Hong SG, Hsu HW, Ye MT (2013) Thermal properties and applications of low molecular weight polyhydroxybutyrate. J Therm Anal Calorim 111(2):1243–1250. https://doi.org/10.1007/s10973-012-2503-3
Hou J, Feng B, Han J, Liu H, Zhao D, Zhou J, Xiang H (2013) Haloarchaeal-type beta-ketothiolases involved in poly(3-hydroxybutyrate-co-3-hydroxyvalerate) synthesis in Haloferax mediterranei. Appl Environ Microbiol 79(17):5104–5111. https://doi.org/10.1128/AEM.01370-13
Hou J, Han J, Cai L, Zhou J, Lu Y, Jin C, Liu JF, Xiang H (2014) Characterization of genes for chitin catabolism in Haloferax mediterranei. Appl Microbiol Biotechnol 98(3):1185–1194. https://doi.org/10.1007/s00253-013-4969-8
Hou J, Xiang H, Han J (2015) Propionyl coenzyme A (propionyl-CoA) carboxylase in Haloferax mediterranei: indispensability for propionyl-CoA assimilation and impacts on global metabolism. Appl Environ Microbiol 81(2):794–804. https://doi.org/10.1128/AEM.03167-14
Ilham M, Nakanomori S, Kihara T, Hokamura A, Matsusaki H, Tsuge T, Mizuno K (2014) Characterization of polyhydroxyalkanoate synthases from Halomonas sp. O-1 and Halomonas elongata DSM2581: site-directed mutagenesis and recombinant expression. Polym Degrad Stab 109:416–423. https://doi.org/10.1016/j.polymdegradstab.2014.04.024
Imanaka H, Yamatsu A, Fukui T, Atomi H, Imanaka T (2006) Phosphoenolpyruvate synthase plays an essential role for glycolysis in the modified Embden-Meyerhof pathway in Thermococcus kodakarensis. Mol Microbiol 61(4):898–909. https://doi.org/10.1111/j.1365-2958.2006.05287.x
Jin HN, Nikolau BJ (2012) Role of genetic redundancy in polyhydroxyalkanoate (PHA) polymerases in PHA biosynthesis in Rhodospirillum rubrum. J Bacteriol 194(20):5522–5529. https://doi.org/10.1128/JB.01111-12
Kim TK, Jung YM, Vo MT, Shioya S, Lee YH (2006) Metabolic engineering and characterization of phaC1 and phaC2 genes from Pseudomonas putida KCTC1639 for overproduction of medium-chain-length polyhydroxyalkanoate. Biotechnol Prog 22(6):1541–1546. https://doi.org/10.1021/bp0601746
Legat A, Gruber C, Zangger K, Wanner G, Stan-Lotter H (2010) Identification of polyhydroxyalkanoates in Halococcus and other haloarchaeal species. Appl Microbiol Biotechnol 87(3):1119–1127
Liu H, Han J, Liu X, Zhou J, Xiang H (2011) Development of pyrF-based gene knockout systems for genome-wide manipulation of the archaea Haloferax mediterranei and Haloarcula hispanica. J Genet Genomics 38(6):261–269. https://doi.org/10.1016/j.jgg.2011.05.003
Liu G, Hou J, Cai S, Zhao D, Cai L, Han J, Zhou J, Xiang H (2015) A patatin-like protein associated with the polyhydroxyalkanoate (PHA) granules of Haloferax mediterranei acts as an efficient depolymerase in the degradation of native PHA. Appl Environ Microbiol 81(9):3029–3038. https://doi.org/10.1128/AEM.04269-14
Liu GM, Cai SF, Hou J, Zhao DH, Han J, Zhou J, Xiang H (2016) Enoyl-CoA hydratase mediates polyhydroxyalkanoate mobilization in Haloferax mediterranei. Sci Rep-Uk 6:24015. https://doi.org/10.1038/srep24015
Lu QH, Han J, Zhou LG, Zhou J, Xiang H (2008) Genetic and biochemical characterization of the poly(3-hydroxybutyrate-co-3-hydroxyvalerate) synthase in Haloferax mediterranei. J Bacteriol 190(12):4173–4180. https://doi.org/10.1128/JB.00134-08
Matsusaki H, Manji S, Taguchi K, Kato M, Fukui T, Doi Y (1998) Cloning and molecular analysis of the poly(3-hydroxybutyrate) and poly(3-hydroxybutyrate-co-3-hydroxyalkanoate) biosynthesis genes in Pseudomonas sp. strain 61-3. J Bacteriol 180(24):6459–6467. https://doi.org/10.1128/JB.180.24.6459-6467.1998
Mitra R, Xu T, Xiang H, Han J (2020) Current developments on polyhydroxyalkanoates synthesis by using halophiles as a promising cell factory. Microb Cell Factories 19(1):86. https://doi.org/10.1186/s12934-020-01342-z
Niersbach M, Kreuzaler F, Geerse RH, Postma PW, Hirsch HJ (1992) Cloning and nucleotide sequence of the Escherichia coli K-12 ppsA gene, encoding PEP synthase. Mol Gen Genet 231(2):332–336. https://doi.org/10.1007/BF00279808
Nishihata S, Kondo T, Tanaka K, Ishikawa S, Takenaka S, Kang CM, Yoshida K (2018) Bradyrhizobium diazoefficiens USDA110 PhaR functions for pleiotropic regulation of cellular processes besides PHB accumulation. BMC Microbiol 18(1):156. https://doi.org/10.1186/s12866-018-1317-2
Orellana R, Macaya C, Bravo G, Dorochesi F, Cumsille A, Valencia R, Rojas C, Seeger M (2018) Living at the frontiers of life: extremophiles in Chile and their potential for bioremediation. Front Microbiol 9:2309. https://doi.org/10.3389/fmicb.2018.02309
Pais J, Serafim LS, Freitas F, Reis MAM (2016) Conversion of cheese whey into poly(3-hydroxybutyrate-co-3-hydroxyvalerate) by Haloferax mediterranei. New Biotechnol 33(1):224–230. https://doi.org/10.1016/j.nbt.2015.06.001
Peplinski K, Ehrenreich A, Doring C, Bomeke M, Reinecke F, Hutmacher C, Steinbuchel A (2010) Genome-wide transcriptome analyses of the 'Knallgas' bacterium Ralstonia eutropha H16 with regard to polyhydroxyalkanoate metabolism. Microbiology 156:2136–2152. https://doi.org/10.1099/mic.0.038380-0
Peralta-Gil M, Segura D, Guzman J, Servin-Gonzalez L, Espin G (2002) Expression of the Azotobacter vinelandii poly-beta-hydroxybutyrate biosynthetic phbBAC operon is driven by two overlapping promoters and is dependent on the transcriptional activator PhbR. J Bacteriol 184(20):5672–5677. https://doi.org/10.1128/jb.184.20.5672-5677.2002
Prieto MA, Buhler B, Jung K, Witholt B, Kessler B (1999) PhaF, a polyhydroxyalkanoate-granule-associated protein of Pseudomonas oleovorans GPo1 involved in the regulatory expression system for pha genes. J Bacteriol 181(3):858–868. https://doi.org/10.1128/JB.181.3.858-868.1999
Pu N, Wang MR, Li ZJ (2020) Characterization of polyhydroxyalkanoate synthases from the marine bacterium Neptunomonas concharum JCM17730. J Biotechnol 319:69–73. https://doi.org/10.1016/j.jbiotec.2020.06.002
Qi X, Lin W, Ma M, Wang C, He Y, He N, Gao J, Zhou H, Xiao Y, Wang Y, Zhang P (2016) Structural basis of rifampin inactivation by rifampin phosphotransferase. Proc Natl Acad Sci U S A 113(14):3803–3808. https://doi.org/10.1073/pnas.1523614113
Quelas JI, Mongiardini EJ, Perez-Gimenez J, Parisi G, Lodeiro AR (2013) Analysis of two polyhydroxyalkanoate synthases in Bradyrhizobium japonicum USDA 110. J Bacteriol 195(14):3145–3155. https://doi.org/10.1128/JB.02203-12
Sakuraba H, Utsumi E, Schreier HJ, Ohshima T (2001) Transcriptional regulation of phosphoenolpyruvate synthase by maltose in the hyperthermophilic archaeon, Pyrococcus furiosus. J Biosci Bioeng 92(2):108–113. https://doi.org/10.1263/jbb.92.108
Sambrook J, Fritsch EF, Maniatis T (1989) Molecular cloning: a laboratory manual, 2nd edn. Cold Spring Harbor Laboratory Press, Cold Spring Harbor
Schmittgen TD, Livak KJ (2008) Analyzing real-time PCR data by the comparative C(T) method. Nat Protoc 3(6):1101–1108. https://doi.org/10.1038/nprot.2008.73
Shih CJ, Chen SC, Weng CY, Lai MC, Yang YL (2015) Rapid identification of haloarchaea and methanoarchaea using the matrix assisted laser desorption/ionization time-of-flight mass spectrometry. Sci Rep-UK 5:16326. https://doi.org/10.1038/srep16326
Siroosi M, Amoozegar MA, Khajeh K, Fazeli M, Rezaei MH (2014) Purification and characterization of a novel extracellular halophilic and organic solvent-tolerant amylopullulanase from the haloarchaeon, Halorubrum sp. strain Ha25. Extremophiles 18(1):25–33. https://doi.org/10.1007/s00792-013-0589-6
Tjaden B, Plagens A, Dorr C, Siebers B, Hensel R (2006) Phosphoenolpyruvate synthetase and pyruvate, phosphate dikinase of Thermoproteus tenax: key pieces in the puzzle of archaeal carbohydrate metabolism. Mol Microbiol 60(2):287–298. https://doi.org/10.1111/j.1365-2958.2006.05098.x
Xue Q, Liu XB, Lao YH, Wu LP, Wang D, Zuo ZQ, Chen JY, Hou J, Bei YY, Wu XF, Leong KW, Xiang H, Han J (2018) Anti-infective biomaterials with surface-decorated tachyplesin I. Biomaterials 178:351–362. https://doi.org/10.1016/j.biomaterials.2018.05.008
Yadav S, Rathore MS, Mishra A (2020) The pyruvate-phosphate dikinase (C4-SmPPDK) gene from Suaeda monoica enhances photosynthesis, carbon assimilation, and abiotic stress tolerance in a C3 plant under elevated CO2 conditions. Front Plant Sci 11:345. https://doi.org/10.3389/fpls.2020.00345
Zhao D, Cai L, Wu J, Li M, Liu H, Han J, Zhou J, Xiang H (2013) Improving polyhydroxyalkanoate production by knocking out the genes involved in exopolysaccharide biosynthesis in Haloferax mediterranei. Appl Microbiol Biotechnol 97(7):3027–3036. https://doi.org/10.1007/s00253-012-4415-3
Zhao Y-X, Rao Z-M, Xue Y-F, Gong P, Ji Y-Z, Ma Y-H (2015) Poly(3-hydroxybutyrate-co-3-hydroxyvalerate) production by Haloarchaeon Halogranum amylolyticum. Appl Microbiol Biotechnol 99(18):7639–7649. https://doi.org/10.1007/s00253-015-6609-y
Zuo ZQ, Xue Q, Zhou J, Zhao DH, Han J, Xiang H (2018) Engineering Haloferax mediterranei as an efficient platform for high level production of lycopene. Front Microbiol 9:2893. https://doi.org/10.3389/fmicb.2018.02893
Acknowledgments
The authors thank Jingnan Liang (Public Technology Service Center of Institute of Microbiology, CAS) in TEM observation.
Funding
This work was financially supported by grants from funding from National Key R&D Program of China (No. 2020YFA0906800), and the National Natural Science Foundation of China (No. 31970031 and No. 91751201).
Author information
Authors and Affiliations
Contributions
JH, HX, and JC designed the experiments. JC performed the experiments. JC and RM analyzed the data. JH, RM, and HX wrote the manuscript. All of the authors read and approved the final version of the manuscript.
Corresponding authors
Ethics declarations
This article does not contain any studies with human participants or animals performed by any of the authors.
Conflict of interest
The authors declare that they have no conflict of interest.
Additional information
Publisher’s note
Springer Nature remains neutral with regard to jurisdictional claims in published maps and institutional affiliations.
Rights and permissions
About this article
Cite this article
Chen, J., Mitra, R., Xiang, H. et al. Deletion of the pps-like gene activates the cryptic phaC genes in Haloferax mediterranei. Appl Microbiol Biotechnol 104, 9759–9771 (2020). https://doi.org/10.1007/s00253-020-10898-0
Received:
Revised:
Accepted:
Published:
Issue Date:
DOI: https://doi.org/10.1007/s00253-020-10898-0