Abstract
Objectives
Long-term cannabis use has been associated with the appearance of psychotic symptoms and schizophrenia-like cognitive impairments; however these studies may be confounded by concomitant use of tobacco by cannabis users. We aimed to determine if previously observed cannabis-associated deficits in sensory gating would be seen in cannabis users with no history of tobacco use, as evidenced by changes in the P50, N100, and P200 event-related potentials. A secondary objective of this study was to examine the effects of acute nicotine administration on cannabis users with no tobacco use history.
Methods
Three components (P50, N100, P200) of the mid-latency auditory-evoked response (MLAER) were elicited by a paired-stimulus paradigm in 43 healthy, non-tobacco smoking male volunteers between the ages of 18–30. Cannabis users (CU, n = 20) were administered nicotine (6 mg) and placebo gum within a randomized, double-blind design. Non-cannabis users (NU, n = 23) did not receive nicotine.
Results
Between-group sensory gating effects were only observed for the N100, with CUs exhibiting a smaller N100 to S1 of the paired stimulus paradigm, in addition to reduced dN100 (indicating poorer gating). Results revealed no significant sensory gating differences with acute administration of nicotine compared to placebo cannabis conditions.
Conclusions
These findings suggest a relationship between gating impairment and cannabis use; however, acute nicotine administration nicotine does not appear to impact sensory gating function.
Similar content being viewed by others
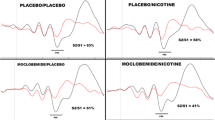
Avoid common mistakes on your manuscript.
Cannabis, schizophrenia, and sensory gating
Cannabis is the collective term of any consumable product or extract from the Cannabis sativa plant with its major psychoactive constituent being Δ-9-tetrahydrocannabinol (THC; Sundram 2006). Consumption of THC, an exogenous cannabinoid, influences the CNS through the endocannabinoid receptors, including CB1, and the changes it induces in the nervous system may ultimately lead to dependence (Fernandez-Espejo et al. 2009).
Overall, both acute and chronic THC have been shown to induce memory impairments similar to those seen in schizophrenia (SZ) including working memory, episodic memory encoding, impaired retrieval, and attentional deficits (Skosnik et al. 2001; Fletcher and Honey 2006); it has been hypothesized that deficits in these higher-order cognitive functions in SZ are in part related to an inability to inhibit irrelevant sensory input, leading to an overload of information reaching consciousness (Patterson et al. 2008). There is also evidence suggesting that there are alterations in the endogenous cannabinoid (eCB) system in SZ (see reviews; Cohen et al. 2008; Leweke et al. 2004).
The cognitive deficits associated with SZ (and, potentially, chronic cannabis use) can be assessed using non-invasive event-related potentials (ERPs), which are derived from scalp-recorded electroencephalographic (EEG) recordings and reflect the brain’s stereotypical responses to sensory stimuli. Using the high temporal resolution of ERPs, information processing can be investigated across the earliest stages of stimulus input through to the transition to higher cognitive operations (Light et al. 2010), several of which are notably impaired in SZ patients (Braff and Light 2004). These objective, brain-based measures have been related to clinical symptoms (Fisher et al. 2008, 2012), neurocognitive deficits (Javitt et al. 2000), and real-world functioning (Light and Braff 2005). Furthermore, ERPs have been used to index psychopharmacological change in cognitive processes (Knott et al. 2009; de la Salle et al. 2019).
P50 ERP suppression is an index of sensory gating that is important in pre-attentional information processing. Sensory gating involves the capacity to filter out irrelevant sensory input from consciousness (Boutros and Belger 1999), presumably to minimize information overload, and to allocate limited resources to more relevant, meaningful stimuli (Evans and Drobes 2009) and, overall, to protect higher order cognitive functions (Venables 1964). P50 suppression is assessed by measuring ERP responses, typically at a central midline electrode (CZ, the site of maximum amplitude), to repeated pairs of brief auditory clicks (Patterson et al. 2008). The P50 wave is identified as the most positive ERP peak occurring 40–80 ms after the first (S1) “conditioning” click and the second (S2) “test” click (Turetsky et al. 2007). Across multiple studies in healthy populations it has been found that there is normally a 70–80% decrease of the S2 P50 wave relative to S1, thought to be due in part to the activation of inhibitory neural circuitry by the first conditioning stimulus (Braff and Light 2004).
While the pre-attentive P50 is the most studied marker of sensory gating, a growing literature has reported that early and later attentive phases of sensory gating-related information processing can be captured by the N100 and P200 components of the mid-latency auditory-evoked response (MLAER; Lijffijt et al. 2009). Indeed, the N100 and P200 are increasingly utilized as neurophysiological markers of sensory gating due to their superior reliability (Anokhin et al. 2007; Boutros et al. 2018; Rentzsch et al. 2008; Shen et al. 2020; Thoma et al. 2020). While these three components are related, they are not dependent and appear to index different neural processes (Boutros et al. 2004; Sklar and Nixon 2014). The P50 appears to reflect pre-attentional inhibitory filter mechanisms, while N100 gating has been suggested to index filter mechanisms (Wan et al. 2008) involved in attention triggering and P200 gating might index filter mechanisms involved in the attentional allocation and early conscious awareness of a stimulus (Lijffijt et al. 2009). Furthermore, it has been suggested that N100 and P200 gating might reflect neural mechanisms that protect cognitive function through interactions with working memory processes to enhance target discrimination (Lijffijt et al. 2009).
A series of replication studies have shown P50 suppression to be reduced in chronic cannabis users who were medically and psychiatrically healthy and did not abuse any illicit substances other than cannabis. The greatest degree of reduction in P50 suppression was observed with the greatest exposure to cannabis (Patrick et al. 1999; Patrick and Struve 2000; Rentzsch et al. 2007; Edwards et al. 2009; Broyd et al. 2013). To date, the effects of cannabis on N100- and P200-indexed sensory gating have yet to be characterized.
The cholinergic system, tobacco use, and sensory gating
Smoking has been shown to improve sensory gating in overnight abstinent smokers with SZ (Adler et al. 1993), and nicotine has been shown to transiently normalize sensory gating among non-smoking relatives of individuals with SZ who all exhibit the deficit (Adler et al. 1992). In minimally deprived smokers, acute smoking has been shown to not affect P50 gating (Croft et al. 2004; Wan et al. 2006, 2007), but nicotine administration was found to enhance gating in healthy non-smokers (Knott et al. 2010a, b) and particularly it was found to enhance gating in individuals with reduced gating efficiency (de la Salle et al. 2013; Knott et al. 2010a, b, 2013). Clinical data has suggested that nicotine improves certain cognitive and sensory abnormalities associated with SZ, such as deficits in sensory gating (Ripoll et al. 2004).
Recent studies have suggested that the α7 subunit-containing nicotinic acetylcholine receptor (α7-nAChR) plays a role in mediating both the response to the first stimulus (S1) and inhibiting response to the subsequent stimulus (S2), perhaps via divergent circuits within the hippocampus (Leiser et al. 2009). α7-nAChRs have been reported to directly influence cellular processes like neurotransmitter release and synaptic plasticity (Fucile 2004) and, thus, is primed to affect higher-order processes that likely underlie cognition (Leiser et al. 2009). Additionally, the endogenous cannabinoid anandamide has been shown to modulate α7-nAChRs (Van Der Stelt and Di Marzo 2005), suggesting that eCB and nicotine systems may interact to modulate sensory gating (Solowij and Michie 2007).
Interactions between the cholinergic and cannabinoid systems
Nicotine and cannabis are frequently used in combination, particularly among adolescents and young adults, and may have synergistic or antagonistic effects (Viveros et al. 2006). While the shared route of administration is likely one of the underlying mechanisms contributing to combined tobacco and cannabis use (Agrawal et al. 2012), including concurrent administration in the same cigarette (or “mulling”; Banbury et al. 2013), it is possible that these two substances are commonly administered together due to synergistic effects (Ream et al. 2008). It has been suggested that comorbid use of cannabis and tobacco may attenuate the cognitive and functional impairments induced by cannabis use alone (Banbury et al. 2013; Rabin and George 2015; de la Salle et al. 2019). Additionally, it has been suggested that cannabis intoxication may potentiate reward sensitivity to nicotine (Rabin and George 2015). Perhaps relatedly, concomitant tobacco and marijuana use has been reported to contribute to cannabis dependence (Ream et al. 2008) and adolescents who consume both cannabis and tobacco appear to experience greater cognitive disruption during smoking cessation than non-users of cannabis. Nicotine may partially mask cannabis-induced deficits in verbal learning, verbal memory formation, and working memory, thereby suggesting that tobacco use among cannabis users may be a form of self-medication in order to avoid impairment of cognitive function which may arise during nicotine withdrawal (Jacobsen et al. 2007).
Summary, study objectives, and hypotheses
While long-term cannabis use has been shown to induce sensory gating impairments similar to those seen in schizophrenia, acute nicotine displays pro-gating effects. It is therefore possible that tobacco use may negate the decrements in sensory gating impairment associated with chronic cannabis use and that this may underlie the combined administration commonly seen. However, given how common combined use is and the fact that both nicotine and cannabis use appears to alter measures of sensory gating, it has been suggested that tobacco use may be a confounding variable in patient and non-patient studies of cannabis use (Croft et al. 2004). To better understand the gating effects of long-term cannabis use independent of tobacco use, the primary objective of this study was to compare P50-indexed sensory gating in chronic cannabis users with no history of cigarette smoking and non-smoking controls. In order to help elucidate the role of nicotinic and cannabinoid receptor interactions on sensory gating, a secondary objective of this study was to investigate the effect of acute nicotine administration on P50, N100, and P200 gating in cannabis users with no history of tobacco use. It was hypothesized that (a) cannabis users would have reduced sensory gating measures compared to non-users and (b) administration of acute nicotine would restore sensory gating measures in cannabis users to the approximate level of non-users.
Materials and methods
Participants
A total of forty-three, right-handed (as assessed by the Edinburgh Handedness Inventory; Oldfield 1971), male volunteers were recruited from the local community. Twenty of these participants were cannabis users (CU) who, for study inclusion, had to report cannabis use prior to 17 years of age, be smoking at least 1 joint per month since the beginning of use and smoking at least 1 joint per week one month prior to study participation. The twenty-three non-cannabis users (NU) had consumed no more than the equivalent of 10 joints in their lifetime and none in the past year. On the basis of a urine toxicology screen, NUs tested negative for THC and all participants tested negative for amphetamines, barbiturates, benzodiazepines, cocaine, ethanol, methadone, opiates, and oxycodone. All participants were required to be non-cigarette smokers, having consumed no more than 100 cigarettes in their lifetime and none in the past year. Non-smoking status was confirmed by analysis of expired air carbon monoxide level (CO), which was required to be below 3 parts per million (ppm). Volunteers were interviewed with regards to their general medical health and their mental health was assessed using the Structural Clinical Interview for DSM-IV Non-Patient Edition (SCID-NP; First et al. 1995). For study inclusion, participants had to report no neurological disorder, prior head injury, hearing impairment, or major medical illness. Participants were excluded if they reported past psychiatric diagnosis, history of neuroleptic or antidepressant use, or treatment for substance abuse of any kind (with the exception of cannabis use for the CU group) as assessed by the SCID-NP, or first degree family member with a psychiatric diagnosis as assessed by the Family Interview for Genetic Studies (FIGS; Maxwell 1992). Written informed consent was obtained prior to testing. The study was cleared by the Research Ethics Boards of the Royal Ottawa Health Care Group and the University of Ottawa and the study was conducted according to the principles of the Declaration of Helsinki.
Design
The effects of nicotine in CU participants were assessed within a randomized, placebo-controlled, counter-balanced, double-blind crossover design requiring them to attend two test sessions, 2–5 days apart. Half of the CUs were randomly selected to receive nicotine during the first session and placebo in the second session and the remaining half received the treatment in the reverse order. NUs were only required to attend one morning session that did not involve nicotine or placebo administration.
Procedure
Participants attended testing sessions in the morning between 8:00 a.m. and 12:30 p.m., with each session lasting approximately 2 h. The test session followed overnight abstinence from drugs, alcohol and medications and 2 h abstinence from caffeine. CUs were instructed to abstain from cannabis consumption 10 days prior to the first scheduled testing date and up until completion of their second session. Although this period of abstinence did not allow for CUs to be completely free of THC (half-life = 20–30 h; Grotenhermen 2003), it did reduce any acute THC effects. Upon arrival to the laboratory, abstinence from cannabis and other drug use was confirmed by verbal report. Subsequent verification was confirmed by urinalysis. The urine tests for all non-users were negative at their test session. All of the 5 users who tested negative during their initial study screening tested negative for both of their test sessions. Of the 15 users who tested THC positive during their initial study screen, 6 were positive in both test sessions, 4 were negative in each test session, and 3 were positive in their first session but negative in their second session.
Following confirmation of abstinence, participants underwent nicotine administration and EEG electrode attachment, and were then presented with an auditory P50 gating paradigm. Following neurophysiological assessment, CU participants completed the Marijuana Withdrawal Checklist (MWC; Budney et al. 1999) and the Checklist of Nicotine-Related Symptoms (CNRS; Harkrider and Hedrick 2005). Vital signs were assessed before and after nicotine administration.
Nicotine
Nicotine (6 mg) was administered orally as two pieces (2-mg Nicorette and 4-mg Nicorette Plus; GlaxoSmithKline) of cinnamon-flavoured polacrix gum. The placebo consisted of 2 pieces of commercially available cinnamon flavoured gum, which matched the active nicotine gum pieces in size, texture, and color. Participants were blindfolded and required to wear a nose plug throughout administration to reduce any sensory differences between placebo and nicotine gums. Complying with manufacturer guidelines, participants were instructed to bite the gum twice per minute and ‘park’ the gum between teeth and cheek between bites for a total time period of 25 min. Immediately following nicotine absorption and prior to nose plug removal, participants removed the nicotine or placebo gum and chewed a commercially available mint flavored “wash-out” gum for approximately 2 min to mask any residual difference in flavour between the nicotine and placebo gum. On the basis of previous pharmacokinetic studies, blood nicotine level was expected to peak between 16–26 ng/ml after 25 min of chewing (elimination half-life of approximately 2 h), which is comparable to the 15–30-ng/ml level typically seen with smoking of a single cigarette with medium nicotine yield (Hukkanen et al. 2005).
ERP acquisition and computation
The paired-stimulus paradigm was presented to participants while they viewed a silent, neutral video. Thirty-two paired clicks (S1–S2) with an inter-click interval of 500 ms and interpair interval of 8 s (Zouridakis and Boutros 1992) were presented binaurally through headphones. The 100-μs clicks were presented with an intensity level of 80 dB (SPL). ERPs were recorded with Ag+/Ag+ Cl− electrodes at 8 scalp sites: Fz, F3, F4, Cz, C3, C4, Pz, and Oz. A mid-forehead site served as ground and an electrode on the nose served as a reference. Electrodes placed at sites above and below the right eye were used to record vertical electro-oculographic activity (VEOG) and electrodes at the outer corners of both eyes were used to record horizontal electro-oculographic activity (HEOG). EEG recordings were carried out using a Brain Vision V-8 Amp® (Brain Products GmbH, Munich DE) amplifier and Brain Vision Recorder® (Brain Products GmbH, Munich DE) software. Electrical activity was sampled at 500 Hz, with bandpass filters set at 0.1–100.0 Hz. Electrical impedance was kept below 5 kΩ. Off-line analysis was performed using Brain Vision Analyzer® (Brain Products GmbH, Munich DE) software.
The paired stimulus paradigms elicited three components of the MLAER: the P50, N100, and P200. In order to analyze the P50, electrical epochs of 150 ms duration (including 50 ms pre-stimulus) were digitally filtered using low and high filters of 10 Hz and 50 Hz, respectively, to increase the signal-to-noise ratio. The N100 and P200 were analyzed within epochs of 400 ms duration (including 50 ms pre-stimulus) using a frequency filter ranging from 0.1-30 Hz. All epochs were ocular corrected, (Gratton et al. 1983) and baseline corrected (relative to the 50 ms pre-stimulus segment), and only epochs with voltages below 50 μV were used for final ERP averaging. Taken from the CZ scalp site, the site of maximal amplitude, P50 amplitudes were measured as the amplitude of the most positive peak from 50 to 110 ms relative to baseline due to a 30-ms delay in our stimulus. Due to the potential ambiguity of the P50, additional constraints were added; the P50 needed to be observable in at least one other central electrode (C3 or C4) and S2 P50 activity needed to peak within 10 ms of the observed peak for S1 P50 (Nagamoto et al. 1991). The N100 and P200 were defined as the largest negative deflection between 80 and 150 ms and the largest positive deflection between 150 and 250 ms, respectively (Gooding et al. 2013). For each component, sensory gating was calculated two ways following the rationale of Broyd et al. (2013). In order to facilitate comparisons with previous cannabis research (Patrick et al. 1999; Rentzsch et al. 2007; Edwards et al. 2009), we calculated ratio (S2/S1) measures of sensory gating for each component (i.e., rP50, rN100, rP200). However, difference measures of sensory gating (S1–S2) have been suggested to be a more reliable index of sensory gating (Smith et al. 1994; Turetsky et al. 2009) and have been increasingly used (Rentzsch et al. 2007; Edwards et al. 2009; Broyd et al. 2013). As such, we also calculated a difference measure of sensory gating for each component (i.e., dP50, dN100, dP200).
Subjective ratings
The Marijuana Withdrawal Checklist (Budney et al. 1999) is a 22-item scale that measures symptoms of marijuana withdrawal after a period of abstinence. Cannabis using participants were asked to indicate which symptoms (including craving, irritability and restlessness) were experienced during their period of marijuana abstinence and rate the severity of each symptom on a four point scale (0 = none, 1 = mild, 2 = moderate, 3 = severe). There is also an open-ended “other” section to capture any additional symptoms that were not listed. A total withdrawal discomfort score (WDS) was created by summing the severity ratings.
The Checklist of Nicotine-Related Symptoms (CNRS; adapted from Harkrider and Hedrick 2005) was employed to measure the severity of nicotine-related adverse symptoms following administration of drug and placebo. Participants were instructed to indicate the severity of their symptoms (including heart-pounding, headache, dizziness, and nausea) on a 5-point scale (0 = no symptoms, 4 = extreme symptoms).
Vital signs
Heart rate (HR) (beats per minute (bpm)) and systolic (SBP) and diastolic (DBP) blood pressure (milliliters per milligram of mercury (mm/mgHg)) were measured while participants were resting in an upright position.
Statistical analyses
Analysis of data was conducted using Statistical Package for the Social Sciences (SPSS; IBM Corp., Armonk, NY). Independent samples t tests were used to compare group means for the demographic data.
Between-group effects
Separate repeated measures analyse of variance (ANOVA) were conducted for group comparisons of P50 amplitudes, N100 amplitudes, and P200 amplitudes between cannabis users and non-users. Each ANOVA contained a between-group factor (NU and CU (placebo only)) and a within-group factor (S1 and S2). Ratio and difference score measures of sensory gating were compared with two-tailed independent samples t tests.
Within-group effects
Further repeated measure ANOVAs containing a drug factor (placebo and nicotine) and stimulus factor (S1 and S2) were conducted in the CUs only. Separate repeated measure ANOVAs with drug as the sole within-subject factor were conducted for ratio and difference score measures of sensory gating. Two-tailed paired-samples t tests were conducted to determine inter-session differences in systolic and diastolic blood pressure, heart rate, and MWS and CNRS scores.
Results
There were no significant differences in age between CU and NU. Participant demographics are presented in Table 1.
Mean values and standard error (SE) are presented
Between-group effects
P50 amplitude
Cannabis users under the placebo condition were compared to non-using controls. As in numerous earlier studies with healthy volunteers (Braff and Light 2004), S2 P50 amplitude in the study sample evidenced an average amplitude suppression of 75.5% relative to S1 P50.
There was a significant main effect of stimulus F(1, 35) = 25.54, p < .001. Follow-up comparisons showed that stimulus 1 (S1; M = 1.22, μV, SE ± .16 ) elicited a larger P50 amplitude, p < 0.001, than stimulus 2 (S2; M = .48 μV, SE ± 0.10). This pattern of larger S1 amplitudes (relative to S2) was observed in both the NU (p = 0.001) and CU (p = 0.002) group. No between-group differences were observed.
N100–P200 amplitudes
There was a significant effect of stimulus type for both N100, F(1, 41) = 67.73, p < .001, and P200, F(1,41) = 116.10, p < .001, in both cases due to larger amplitudes for S1 relative to S2. There was also a significant stimulus-by-group interaction for N100 only, F(1, 41) = 4.23, p = .046; followed up, this revealed N100 S1 was significantly larger (p = .037) in NUs (M = −7.40 μV, SE ± 0.62) than in CUs (M = −5.42 μV, SE ± 0.67), as shown in Fig. 1.
Gating measures
Independent samples t tests revealed no significant group differences for both dP50 and rP50, between CUs and NUs. Conversely, there was a significant group difference for dN100, t(40) = 2.42, p = .020, due to a larger S1–S2 difference in NUs (M = −3.76, SE ± 0.18) relative to CUs (M = 1.84, SE ± 0.51), as shown in Fig. 2.
Drug effects
P50 amplitude
As observed above with the analysis of placebo responses, a significant main effect of stimulus, F(1, 20) = 12.09, p = .002, was observed with S1 (M = 1.14 μV, SE ± 0.22) exhibiting a significantly larger P50 amplitude than S2 (M = 0.46 μV, SE ± 0.10). No main effect of drug was present and no drug by stimulus interaction.
N100–P200 amplitudes
Beyond the expected significant main effect of stimulus type (S1 > S2, p < .001 for both N100 and P200), there were no significant main or interaction effects for analyses comparing nicotine and placebo condition in CUs.
Gating measures
There were no significant main effects or interaction effects present for nicotine and placebo cannabis conditions as measured by dP50 and rP50. Paired samples t tests revealed that there were no significant differences for either rP50 and dP50 between nicotine and placebo cannabis conditions.
In addition to Spearman’s rho correlations being performed to assess gating measures and or cannabis use variables of interest (age of onset, weekly use, recent use, and years of use), we saw a moderate positive correlation between the current age and the rP50 amplitude indicating that as age increased so did rP50.
Subjective ratings
Marijuana withdrawal checklist
No significant withdrawal differences were observed between placebo and nicotine in cannabis users.
Checklist of nicotine-related symptoms
In general, a significant drug effect in CUs F(1, 15), p < 0.008, showed higher symptom scores after receiving nicotine (M = 1.74, SE ± 0.19) compared with after receiving placebo (M = 1.06, SE ± 0.06).
Vital signs
There were no significant group, drug, or drug × group effects observed with heart rate, systolic blood pressure, or diastolic blood pressure.
Discussion
This study was done to investigate sensory gating (as indexed by the P50, N100, and P200) in long-term CU compared to NU, both of which reported no history of tobacco use. We were also interested in the effect of acute nicotine administration in cannabis users on sensory gating ability. We hypothesized that CUs would have reduced P50 sensory gating; this was not supported, however CUs did exhibit reduced N100-indexed sensory gating and smaller N100 amplitudes to S1. Notably, this study is the first to characterize N100 and P200 sensory gating in cannabis users and the first to report N100-related deficits. We hypothesized that administration of acute nicotine would restore the P50 amplitude and gating in CUs to the approximate level of NUs was not supported.
Although not evidenced in the gating indices, our findings were consistent with previous research (Braff and Light 2004) showing S2 P50 amplitude suppression relative to S1 P50 within both the NU and CU groups. The lack of P50-indexed differences between groups, either for S1 and S2 amplitudes or sensory gating measures differs from earlier investigations in cannabis users (Patrick et al. 1999; Patrick and Struve 2000; Rentzsch et al. 2007; Edwards et al. 2009). The deviation in our findings may be explained by differences in our study sample relative to previous studies. The cannabis users in a previous study by Patrick et al. (1999) for example, had a much greater cumulative duration of THC exposure (13.5 years) and mean consumption rate (13.2 joints per week) than the CUs in the present study (3.49 g, or approximately 7 joints, per week). In a similar study, when cannabis users were divided into long- and short-term users by way of median split, sensory gating deficits were only present in long term, but not short term (mean duration of regular use: 6.1 years) users (Broyd et al. 2013). Importantly, this latter study also attempted to control for the potential confounding effects of nicotine use. Taken together, these studies suggest that mild-to-moderate short-term cannabis use is not associated with pre-attentive sensory gating deficits, at least when controlling for concurrent nicotine use. This is further supported by recent work showing a differential response of the pre-attentive, automatic mismatch negativity (MMN) waveform between short-term and long-term tobacco-naïve cannabis users, where deficits were only observed in long-term users (Impey et al. 2015).
This is consistent with previous findings that cannabis-induced changes in cognition are associated with higher levels of circulating THC (Hunault et al. 2009), but reverse with abstinence (Rabin et al. 2017; Melissa et al. 2018). Furthermore, users in our sample are moderate cannabis users when compared to other studies, which may be why no significant gating differences were observed (Patrick and Struve 2000; Edwards et al. 2009; Broyd et al. 2013). Another significant difference between our study and past research is that our participants were acutely administered nicotine. Previous reports indicate that nicotine may be used to help mask the undesirable effects of cannabis such as impairments in cognitive functioning and the sedative effects (de la Salle et al 2019; Fucile 2004; Harkrider and Hedrick 2005; Viveros et al. 2006). This may explain why there was no significant difference in sensory gating in our cannabis using participants, as the nicotine that was acutely administered may have combated the sensory gating impairments common in cannabis users. Finally, it could have been a combination of decreased cannabis consumption in our sample paired with the acute administration of nicotine which may have made for an optimal gating capability for our participants.
Contrary to the P50, group differences in N100-indexed sensory gating (dN100) were observed, despite the relatively short duration of cannabis use in our sample. These findings suggest that cannabis use may have a great effect on later attention triggering aspects of the stimulus filter process, rather than early sensory inhibition processes. This pattern, combined with reports of pre-attentional (P50) deficits in cannabis users with a longer duration of use, suggests that deficits may be limited to later, more complex processes, which are more susceptible insult relative to earlier, pre-attentive and automatic processes (Fisher et al. 2010). Also, of interest is that the observed N100 gating deficits appear to have resulted mostly from a decrease in S1 rather than from an increase in S2, indicating a disturbance in attention capture processes in a manner typically reported in schizophrenia patients (Blumenfeld and Clementz 2001; Clementz and Blumenfeld 2001; Hu et al. 2012). This is notable given the purported similarities in cognitive deficits seen between cannabis use and schizophrenia (Skosnik et al. 2001; Fletcher and Honey 2006) and suggests overlap in in some of the underlying neural mechanisms, such as those associated with early sensory gating processes.
Limitations
There are several limitations to this study including relatively small sample sizes and the use of a relatively young population. Furthermore, although there were heavy long-term users in the CU group, it is possible that more robust results would be observed in a population with an overall longer history of use and/or greater current use. The relatively light use among our CUs may be an artefact of our selection criteria. Due to the requirement to abstain from cannabis for 10 days, this may have deterred heavier users from participating. Future studies may want to waive this requirement in order to obtain a more representative sample of CUs, albeit at the cost of potential introducing increased circulating THC levels as a potential confound. Additionally, we only recorded the amount of cannabis reportedly consumed by each participant and did not account for varying amounts of THC that may be present depending on the source, as different strains of marijuana are known to vary in their respective THC content and THC/Cannabidiol ratio (Burgdorf et al. 2011). This study also utilized a single dose of nicotine that was absorbed buccally, which differs greatly from the way in which nicotine is absorbed from a cigarette. Different routes of administration may impact the degree to which different nAChR subunit types are activated and/or desensitized, as might different doses of nicotine. While we did administer a relatively small dosage of nicotine, it is also possible that the administration of nicotine to nicotine naïve individuals caused adverse side effects that could impact our findings. Indeed, there was an increase in nicotine-related symptoms following administration of nicotine (relative to placebo); however, the average strength of effects in both conditions was in the mild-to-moderate range. In addition to this, we only collected data from our control participants once while cannabis users were brought into the lab twice for testing, therefore half of the data reported for the cannabis users under the placebo condition is representative of their second testing session, and therefore could lead to practice effects. Finally, this study only included male participants; given the suggestion that cannabis may differentially affect males and females (Ketcherside et al. 2016; Cooper and Craft 2018), future work should include sex as a biological variable.
Conclusions
In summary, this study examined sensory gating in cannabis users while controlling for nicotine exposure, which has been a common concomitant confounding variable in previous studies. This study is the first to investigate the acute effect of nicotine on sensory gating in cannabis users, while also being the first (to our knowledge) to characterize later MLAEP markers of sensory gating (i.e., N100 and P200) in this group. Our findings show no P50-indexed sensory gating impairments in otherwise healthy volunteers and no overall impact of nicotine on gating contrary to previous work. Given the relatively low cannabis consumption rates in our sample, this may suggest that early sensory gating deficits only emerge with increased cannabis use, or that pre-existing sensory gating deficits may drive greater cannabis use, and that low-to-moderate cannabis use is only associated with later (i.e., N100) markers of sensory gating.
Overall, this suggests that further research is needed to clarify the relationship between cannabis use and acute nicotine administration and how this may act as a potential modulator of the gating disruptions incurred by long-term and recent cannabis use.
References
Adler LE, Hoffer LJ, Griffith J, Waldo MC, Freedman R (1992) Normalization by nicotine of deficient auditory sensory gating in the relatives of schizophrenics. Biol Psychiatry 32:607–616. https://doi.org/10.1016/0006-3223(92)90073-9
Adler LE, Hoffer LD, Wiser A, Freedman R (1993) Normalization of auditory physiology by cigarette smoking in schizophrenic patients. Am J Psychiatry 150:1856–1861. https://doi.org/10.1176/ajp.150.12.1856
Agrawal A, Budney AJ, Lynskey MT (2012) The co-occurring use and misuse of cannabis and tobacco: A review. Addiction 107:1221–1233
Anokhin AP, Vedeniapin AB, Heath AC, Korzyukov O, Boutros NN (2007) Genetic and environmental influences on sensory gating of mid-latency auditory evoked responses: a twin study. Schizophr Res 89:312–319. https://doi.org/10.1016/j.schres.2006.08.009
Banbury A, Zask A, Carter SM, van Beurden E, Tokley R, Passey M, Copeland J (2013) Smoking mull: a grounded theory model on the dynamics of combined tobacco and cannabis use among adult men. Heal Promot J Aust 24:143–150. https://doi.org/10.1071/HE13037
Blumenfeld LD, Clementz BA (2001) Response to the first stimulus determines reduced auditory evoked response suppression in schizophrenia: single trials analysis using MEG. Clin Neurophysiol 112:1650–1659. https://doi.org/10.1016/S1388-2457(01)00604-6
Boutros NN, Belger A (1999) Midlatency evoked potentials attenuation and augmentation reflect different aspects of sensory gating. Biol Psychiatry 45:917–922. https://doi.org/10.1016/S0006-3223(98)00253-4
Boutros NN, Korzyukov O, Jansen B, Feingold A, Bell M (2004) Sensory gating deficits during the mid-latency phase of information processing in medicated schizophrenia patients. Psychiatry Res 126:203–215. https://doi.org/10.1016/j.psychres.2004.01.007
Boutros N, Bowyer SM, Ford Hospital H (2018) Evoked potentials investigations of deficit versus nondeficit schizophrenia: EEG-MEG preliminary data predicting on-road driver performance from laboratory tests view project cognitive demand view project. Artic Clin EEG Neurosci Off J EEG Clin Neurosci Soc 50:75–87. https://doi.org/10.1177/1550059418797868
Braff DL, Light GA (2004) Preattentional and attentional cognitive deficits as targets for treating schizophrenia. Psychopharmacology 174:75–85. https://doi.org/10.1007/s00213-004-1848-0
Broyd SJ, Marie GL, Croft RJ et al (2013) Chronic effects of cannabis on sensory gating. Int J Psychophysiol 89:381–389. https://doi.org/10.1016/j.ijpsycho.2013.04.015
Budney AJ, Novy PL, Hughes JR (1999) Marijuana withdrawal among adults seeking treatment for marijuana dependence. Addiction 94:1311–1322. https://doi.org/10.1046/j.1360-0443.1999.94913114.x
Burgdorf JR, Kilmer B, Pacula RL (2011) Heterogeneity in the composition of marijuana seized in California. Drug Alcohol Depend 117:59–61. https://doi.org/10.1016/j.drugalcdep.2010.11.031
Clementz BA, Blumenfeld LD (2001) Multichannel electroencephalographic assessment of auditory evoked response suppression in schizophrenia. Exp Brain Res 139:377–390. https://doi.org/10.1007/s002210100744
Cohen M, Solowij N, Carr V (2008) Cannabis, cannabinoids and schizophrenia: integration of the evidence. Aust N Z J Psychiatry 42:357–368. https://doi.org/10.1080/00048670801961156
Cooper ZD, Craft RM (2018) Sex-dependent effects of cannabis and cannabinoids: a translational perspective. Neuropsychopharmacology 43:34–51
Croft RJ, Dimoska A, Gonsalvez CJ, Clarke AR (2004) Suppression of P50 evoked potential component, schizotypal beliefs and smoking. Psychiatry Res 128:53–62. https://doi.org/10.1016/j.psychres.2004.05.009
de la Salle S, Smith D, Choueiry J, Impey D, Philippe T, Dort H, Millar A, Albert P, Knott V (2013) Effects of COMT genotype on sensory gating and its modulation by nicotine: differences in low and high P50 suppressors. Neuroscience 241:147–156. https://doi.org/10.1016/j.neuroscience.2013.03.029
de la Salle S, Inyang L, Impey D, Smith D, Choueiry J, Nelson R, Heera J, Baddeley A, Ilivitsky V, Knott V (2019) Acute separate and combined effects of cannabinoid and nicotinic receptor agonists on MMN-indexed auditory deviance detection in healthy humans. Pharmacol Biochem Behav 184:172739. https://doi.org/10.1016/j.pbb.2019.172739
Edwards CR, Skosnik PD, Steinmetz AB et al (2009) Sensory gating impairments in heavy cannabis users are associated with altered neural oscillations. Behav Neurosci 123:894–904. https://doi.org/10.1038/jid.2014.371
Evans DE, Drobes DJ (2009) Nicotine self-medication of cognitive-attentional processing. Addict Biol 14:32–42. https://doi.org/10.1111/j.1369-1600.2008.00130.x
Fernandez-Espejo E, Viveros M-P, Núñez L, Ellenbroek BA, Rodriguez de Fonseca F (2009) Role of cannabis and endocannabinoids in the genesis of schizophrenia atypical psychotic symptoms in Lafora’s disease: a case report view project Cannabis and Human Effects View project. Springer 206:531–549. https://doi.org/10.1007/s00213-009-1612-6
First M, Spitzer R, Williams J, Gibbon M (1995) Structured clinical interview for DSM-IV-non-patient edition (SCID-NP, Version 1.0). Am Psychiatr Washington, DC
Fisher DJ, Labelle A, Knott VJ (2008) Auditory hallucinations and the mismatch negativity: processing speech and non-speech sounds in schizophrenia. Int J Psychophysiol 70:3–15. https://doi.org/10.1016/j.ijpsycho.2008.04.001
Fisher DJ, Labelle A, Knott VJ (2010) Auditory hallucinations and the P3a: attention-switching to speech in schizophrenia. Biol Psychol 85:417–423. https://doi.org/10.1016/j.biopsycho.2010.09.003
Fisher DJ, Labelle A, Knott VJ (2012) Alterations of mismatch negativity (MMN) in schizophrenia patients with auditory hallucinations experiencing acute exacerbation of illness. Schizophr Res 139:237–245. https://doi.org/10.1016/j.schres.2012.06.004
Fletcher PC, Honey GD (2006) Schizophrenia, ketamine and cannabis: evidence of overlapping memory deficits. Trends Cogn Sci 10:167–174. https://doi.org/10.1016/j.tics.2006.02.008
Fucile S (2004) Ca2+ permeability of nicotinic acetylcholine receptors. Cell Calcium 35:1–8
Gooding DC, Gjini K, Burroughs SA, Boutros NN (2013) The association between psychosis proneness and sensory gating in cocaine-dependent patients and healthy controls. Psychiatry Res 210:1092–1100. https://doi.org/10.1016/j.psychres.2013.08.049
Gratton G, Coles MGH, Donchin E (1983) A new method for off-line removal of ocular artifact. Electroencephalogr Clin Neurophysiol 55:468–484. https://doi.org/10.1016/0013-4694(83)90135-9
Grotenhermen F (2003) Pharmacokinetics and pharmacodynamics of cannabinoids, vol 42. Springer, Berlin, pp 327–360. https://doi.org/10.2165/00003088-200342040-00003
Harkrider AW, Hedrick MS (2005) Acute effect of nicotine on auditory gating in smokers and non-smokers. Hear Res 202:114–128. https://doi.org/10.1016/j.heares.2004.11.009
Hu L, Boutros NN, Jansen BH (2012) Sensory gating-out and gating-in in normal and schizophrenic participants. journals.sagepub.com 43:23–31. https://doi.org/10.1177/1550059411429524
Hukkanen J, Iii PJ, Benowitz NL (2005) Metabolism and disposition kinetics of nicotine. ASPET. 57:79–115. https://doi.org/10.1124/pr.57.1.3
Hunault CC, Mensinga TT, Böcker KBE, Schipper CMA, Kruidenier M, Leenders MEC, de Vries I, Meulenbelt J (2009) Cognitive and psychomotor effects in males after smoking a combination of tobacco and cannabis containing up to 69 mg delta-9-tetrahydrocannabinol (THC). Psychopharmacology Springer 204:85–94. https://doi.org/10.1007/s00213-008-1440-0
Impey D, El-Marj N, Parks A et al (2015) Mismatch negativity in tobacco-naïve cannabis users and its alteration with acute nicotine administration. Pharmacol Biochem Behav 136:73–81. https://doi.org/10.1016/j.pbb.2015.07.002
Jacobsen LK, Pugh KR, Constable RT, Westerveld M, Mencl WE (2007) Functional correlates of verbal memory deficits emerging during nicotine withdrawal in abstinent adolescent cannabis users. Biol Psychiatry 61:31–40. https://doi.org/10.1016/j.biopsych.2006.02.014
Javitt DC, Shelley AM, Silipo G, Lieberman JA (2000) Deficits in auditory and visual context-dependen processing in schizophrenia: defining the pattern. Arch Gen Psychiatry 57:1131–1137. https://doi.org/10.1001/archpsyc.57.12.1131
Ketcherside A, Baine J, Filbey F (2016) Sex effects of marijuana on brain structure and function. Curr Addict Reports 3:323–331. https://doi.org/10.1007/s40429-016-0114-y
Knott V, Millar A, Fisher D (2009) Sensory gating and source analysis of the auditory P50 in low and high suppressors. Neuroimage 44:992–1000. https://doi.org/10.1016/j.neuroimage.2008.10.002
Knott V, Millar A, Fisher D, Albert P (2010a) Effects of nicotine on the amplitude and gating of the auditory P50 and its influence by dopamine D2 receptor gene polymorphism. Neuroscience 166:145–156. https://doi.org/10.1016/j.neuroscience.2009.11.053
Knott VJ, Fisher DJ, Millar AM (2010b) Differential effects of nicotine on P50 amplitude, its gating, and their neural sources in low and high suppressors. Neuroscience 170:816–826. https://doi.org/10.1016/j.neuroscience.2010.07.012
Knott VJ, De La Salle S, Smith D et al (2013) Baseline dependency of nicotine’s sensory gating actions: similarities and differences in low, medium and high P50 suppressors. J Psychopharmacol 27:790–800. https://doi.org/10.1177/0269881113490449
Leiser SC, Bowlby MR, Comery TA, Dunlop J (2009) A cog in cognition: how the α7 nicotinic acetylcholine receptor is geared towards improving cognitive deficits. Pharmacol Ther 122:302–311
Leweke FM, Gerth CW, Klosterkötter J (2004) Cannabis-associated psychosis: current status of research. CNS Drugs 18:895–910
Light GA, Braff DL (2005) Mismatch negativity deficits are associated with poor functioning in schizophrenia patients. Arch Gen Psychiatry 62:127–136. https://doi.org/10.1001/archpsyc.62.2.127
Light GA, Williams LE, Minow F, et al (2010) Electroencephalography (EEG) and event-related potentials (ERPs) with human participants. Curr. Protoc. Neurosci.
Lijffijt M, Lane SD, Meier SL, Boutros NN, Burroughs S, Steinberg JL, Gerard Moeller F, Swann AC (2009) P50, N100, and P200 sensory gating: relationships with behavioral inhibition, attention, and working memory. Psychophysiology 46:1059–1068. https://doi.org/10.1111/j.1469-8986.2009.00845.x
Maxwell E (1992) Family interview for genetic studies (FIGS). Fam Interview Genet Stud 1–7
Melissa R, Gilman J, Schoenfeld D et al (2018) One month of cannabis abstinence in adolescents and young adults is associated with improved memory. J Clin Psychiatry 79. https://doi.org/10.4088/JCP.17m11977
Nagamoto HT, Adler LE, Waldo MC, Griffith J, Freedman R (1991) Gating of auditory response in schizophrenics and normal controls. Effects of recording site and stimulation interval on the P50 wave. Schizophr Res 4:31–40. https://doi.org/10.1016/0920-9964(91)90007-E
Oldfield RC (1971) The assessment and analysis of handedness: the Edinburgh inventory. Neuropsychologia 9:97–113. https://doi.org/10.1016/0028-3932(71)90067-4
Patrick G, Struve FA (2000) Reduction of auditory P50 gating response in marijuana users: further supporting data. Clin EEG Neurosci 31:88–93. https://doi.org/10.1177/155005940003100207
Patrick G, Straumanis JJ, Struve FA, Fitz-Gerald MJ, Leavitt J, Manno JE (1999) Reduced P50 auditory gating response in psychiatrically normal chronic marihuana users: a pilot study. Biol Psychiatry 45:1307–1312. https://doi.org/10.1016/S0006-3223(98)00155-3
Patterson JV, Hetrick WP, Boutros NN, Jin Y, Sandman C, Stern H, Potkin S, Bunney WE Jr (2008) P50 sensory gating ratios in schizophrenics and controls: a review and data analysis. Psychiatry Res 158:226–247. https://doi.org/10.1016/j.psychres.2007.02.009
Rabin RA, George TP (2015) A review of co-morbid tobacco and cannabis use disorders: possible mechanisms to explain high rates of co-use. Am J Addict 24:105–116. https://doi.org/10.1111/ajad.12186
Rabin RA, Barr MS, Goodman MS, Herman Y, Zakzanis KK, Kish SJ, Kiang M, Remington G, George TP (2017) Effects of extended cannabis abstinence on cognitive outcomes in cannabis dependent patients with schizophrenia vs non-psychiatric controls. Neuropsychopharmacology 42:2259–2271. https://doi.org/10.1038/npp.2017.85
Ream GL, Benoit E, Johnson BD, Dunlap E (2008) Smoking tobacco along with marijuana increases symptoms of cannabis dependence. Drug Alcohol Depend 95:199–208. https://doi.org/10.1016/j.drugalcdep.2008.01.011
Rentzsch J, Penzhorn A, Kernbichler K et al (2007) Differential impact of heavy cannabis use on sensory gating in schizophrenic patients and otherwise healthy controls. Exp Neurol 205:241–249. https://doi.org/10.1016/j.expneurol.2007.02.004
Rentzsch J, De Castro AG, Neuhaus A et al (2008) Comparison of midlatency auditory sensory gating at short and long interstimulus intervals. Neuropsychobiology 58:11–18. https://doi.org/10.1159/000154475
Ripoll N, Bronnec M, Bourin M (2004) Nicotinic receptors and schizophrenia. Curr Med Res Opin 20:1057–1074
Shen CL, Chou TL, Lai WS, Hsieh MH, Liu CC, Liu CM, Hwu HG (2020) P50, N100, and P200 auditory sensory gating deficits in schizophrenia patients. Front Psychiatry 11:1–11. https://doi.org/10.3389/fpsyt.2020.00868
Sklar AL, Nixon SJ (2014) Disruption of sensory gating by moderate alcohol doses. Psychopharmacology 231:4393–4402. https://doi.org/10.1007/s00213-014-3591-5
Skosnik PD, Spatz-Glenn L, Park S (2001) Cannabis use is associated with schizotypy and attentional disinhibition. Schizophr Res 48:83–92. https://doi.org/10.1016/S0920-9964(00)00132-8
Smith DA, Boutors NN, Schwarzoph SB (1994) Reliability of P50 auditory event-related potential indices of sensory gating. Psychophysiology 31:495–502. https://doi.org/10.1111/j.1469-8986.1994.tb01053.x
Solowij N, Michie P (2007) Cannabis and cognitive dysfunction: parallels with endophenotypes of schizophrenia? J Psychiatry Neurosci 32:30–52
Sundram S (2006) Cannabis and neurodevelopment: Implications for psychiatric disorders. Hum Psychopharmacol 21:245–254. https://doi.org/10.1002/hup.762
Thoma L, Rentzsch J, Gaudlitz K, Tänzer N, Gallinat J, Kathmann N, Ströhle A, Plag J (2020) P50, N100, and P200 sensory gating in panic disorder. Clin EEG Neurosci 51:317–324. https://doi.org/10.1177/1550059419899324
Turetsky BI, Calkins ME, Light GA, Olincy A, Radant AD, Swerdlow NR (2007) Neurophysiological endophenotypes of schizophrenia: the viability of selected candidate measures. Schizophr Bull 33:69–94. https://doi.org/10.1093/schbul/sbl060
Turetsky BI, Bilker WB, Siegel SJ, Kohler CG, Gur RE (2009) Profile of auditory information-processing deficits in schizophrenia. Psychiatry Res 165:27–37. https://doi.org/10.1016/j.psychres.2008.04.013
Van Der Stelt M, Di Marzo V (2005) Anandamide as an intracellular messenger regulating ion channel activity. Prostaglandins Other Lipid Mediat 77:111–122. https://doi.org/10.1016/j.prostaglandins.2004.09.007
Venables PH (1964) Input dysfunction in schizophrenia. Progr Exp Personal Res 72:1–47
Viveros MP, Marco EM, File SE (2006) Nicotine and cannabinoids: parallels, contrasts and interactions. Neurosci Biobehav Rev 30:1161–1181
Wan L, Crawford HJ, Boutros N (2006) P50 sensory gating: impact of high vs. low schizotypal personality and smoking status. Int J Psychophysiol 60:1–9. https://doi.org/10.1016/j.ijpsycho.2005.03.024
Wan L, Crawford HJ, Boutros N (2007) Early and late auditory sensory gating: moderating influences from schizotypal personality, tobacco smoking status, and acute smoking. Psychiatry Res 151:11–20. https://doi.org/10.1016/j.psychres.2006.01.020
Wan L, Friedman BH, Boutros NN, Crawford HJ (2008) P50 sensory gating and attentional performance. Int J Psychophysiol 67:91–100. https://doi.org/10.1016/j.ijpsycho.2007.10.008
Zouridakis G, Boutros NN (1992) Stimulus parameter effects on the P50 evoked response. Biol Psychiatry 32:839–841. https://doi.org/10.1016/0006-3223(92)90088-h
Acknowledgements
This project was supported by a Natural Sciences and Engineering Research Council of Canada (NSERC) operating grant (VK), as well as a Frederick Banting and Charles Best Canada Graduate Scholarship (Doctoral) from the Canadian Institutes of Health Research (CIHR) awarded to DF.
Author information
Authors and Affiliations
Corresponding author
Ethics declarations
Conflict of interest
The authors declare no conflict of interest.
Additional information
Publisher’s note
Springer Nature remains neutral with regard to jurisdictional claims in published maps and institutional affiliations.
This article belongs to a Special Issue on Cannabis and Cannabinoids
Rights and permissions
About this article
Cite this article
Francis, A.M., Parks, A., Choueiry, J. et al. Sensory gating in tobacco-naïve cannabis users is unaffected by acute nicotine administration. Psychopharmacology 239, 1279–1288 (2022). https://doi.org/10.1007/s00213-021-05843-6
Received:
Accepted:
Published:
Issue Date:
DOI: https://doi.org/10.1007/s00213-021-05843-6