Abstract
Titanium dioxide nanoparticles (TiO2 NPs) are ubiquitous in the environment and enter the terrestrial food chain via plant uptake. However, plant uptake behaviors of TiO2 NPs remain elusive. Here, the uptake kinetics of TiO2 NPs by wheat (Triticum aestivum L.) seedlings and the effects on cation flux in roots were examined in a hydroponic system. Uptake rate of TiO2 NPs ranged from 119.0 to 604.2 mg kg− 1 h− 1 within 8 h exposure. NP uptake decreased by 83% and 47%, respectively, in the presence of sodium azide (NaN3) and carbonyl cyanide m-chlorophenylhydrazone (CCCP), indicating an energy-dependent uptake of TiO2 NPs. Moreover, accompanied with TiO2 NP uptake, net influx of Cd2+ decreased by 81%, while Na+ flux shifted from inflow to outflow at the meristematic zone of root. These findings provide valuable information for understanding plant uptake of TiO2 NPs.
Similar content being viewed by others
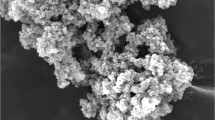
Explore related subjects
Discover the latest articles, news and stories from top researchers in related subjects.Avoid common mistakes on your manuscript.
Introduction
Titanium dioxide nanoparticles (TiO2 NPs) are widely used as an absorbent of ultraviolet light in cosmetics, as a pigment for paints, as a food additive, and as nano-pesticides and fertilizers in agriculture (Farooq et al. 2022). In parallel, they are produced naturally through volcanic eruptions or mineral weathering (Hochella et al. 2019). These NPs can end up in the terrestrial environment and inevitably interact with plants. Plant uptake of these NPs is the first step toward their trophic transfer and plays an important role in risk assessments of NPs (Ma et al. 2015).
Previous studies have demonstrated that TiO2 NPs can be internalized in a wide diversity of plants. For instance, TiO2 NPs at 2.8 nm could pass the root cell walls of Arabidopsis thaliana seedlings, and accumulate in cell vacuoles and nuclei (Kurepa et al. 2010). TiO2 NPs (27 nm) were detected mainly in the root dermis and cortex of cucumber (Cucumis sativus), and transported to the leaf trichomes (Servin et al. 2012). Transmission electron microscopy analysis showed the presence of TiO2 NPs in cell walls, endoderm, Casparian band, vascular cylinders and cell nuclei in roots of Nigella arvensis L. after 21 days exposure in hydroponics (Chahardoli et al. 2022). After internalization, these NPs were translocated to leaves, fruits and seeds without biotransformation (Belhaj Abdallah et al. 2020; Larue et al. 2012a; Larue et al. 2012b). There is currently very little information, however, on uptake kinetics of NPs, which provide basic information on particulate uptake of TiO2 NPs.
Accumulation of TiO2 NPs has been demonstrated to decrease nutrient/contaminant concentrations, such as Mg, Ca, and Cd in plants (Hu et al. 2020; Ji et al. 2017). This was attributed to the adsorption of nutrient/contaminant on TiO2 NPs in the exposure medium, and thereby, the decrease in their phytoavailability (Hu et al. 2020). Alternatively, it was proposed that TiO2 NPs blocked the cell wall pores of roots, and decreased cation uptake (Asli and Neumann 2009; Ren et al. 2011). There is currently little evidence, however, on cation flux at root after TiO2 NP exposure.
In this study, we quantified the uptake kinetics of TiO2 NPs and their effects on cation fluxes in hydroponic wheat (Triticum aestivum L.) seedlings. We employed non-invasive micro-test technique, an in situ method that monitored the real-time changes of cation fluxes in the roots, to provide insights into cation uptake in response to TiO2 NP exposure. Our study offers important insights into plant uptake of TiO2 NPs, which was an initial step of their entry into the terrestrial food web.
Materials and Methods
TiO2 NPs and Plant
Titanium dioxide nanoparticles (TiO2 NPs) without coating were obtained from XFNANO Ltd., Nanjing, China. After suspension in the exposure medium by ultrasonication, the morphology, size, number-weighted hydrodynamic diameters, zeta potential, and specific surface area of TiO2 NPs were characterized. More details on the characterization of NPs are provided in Supporting Information Text S1.
Wheat seeds (Triticum aestivum L.) were germinated on filter paper and moistened with deionized water in dark at 25 ℃ for 2 days. Then they were transplanted into Hoagland solution (Table S1) under a 16 h day/8 h night with a relative humidity of 80%. The nutrient solution was renewed every two days.
Uptake Experiments
TiO2 NP Uptake with or Without Inhibitors
Wheat seedlings at 15-d-old were exposed to a series of titanium concentrations (5, 10 and 30 mg L− 1) for 8 h. Exposure concentrations were within the concentrations that exist in nature (Kabata-Pendias and Mukherjee 2007). At each time point (1, 4, and 8 h), aliquot of the exposure medium was sampled to measure total Ti and dissolved Ti concentrations, and whole wheat seedlings were sampled for total Ti analysis.
To verify the uptake mechanism, three types of inhibitors were examined. Carbonyl cyanide m-chlorophenylhydrazone (CCCP) is a protonophore and an uncoupler of oxidative phosphorylation, which causes a dissipation of the proton motive force across the membranes; it was used as a metabolic inhibitor (Xu et al., 2007). Sodium azide (NaN3) blocked the protein and enzyme activity to inhibit cellular endocytosis process, and was used as an endocytic inhibitor (Li et al. 2016). Mercury binds to the sulfhydryl group of cys residues in the vicinity of the aqueous pore, thereby blocking most aquaporin and root water transport (Javot and Maurel, 2002). Uptake experiments were performed with 10 mg L− 1 TiO2 NPs in the presence or absence of CCCP or Hg(NO3)2. CCCP was dissolved in ethanol before adding into the exposure medium, with a final CCCP concentration of 20 µM and ethanol concentration of 0.1% (v/v). Simultaneously, a control group containing 0.1% ethanol without CCCP was included. Ethanol at 0.1% did not affect TiO2 NP uptake (p > 0.05). As for Hg(NO3)2, Hg concentration was 100 µM. Wheat seedlings were pre-exposed to NaN3 for 1 h, rinsed with deionized water thoroughly and then subjected to TiO2 NP exposure to avoid the aggregation of TiO2 NPs by NaN3 (Fig. S1). Moreover, the inhibition in endocytosis process by NaN3 last up to 12 h (Li et al. 2016), longer than the exposure duration of 8 h in this study. All experiments were performed with four replicate pots in each treatment, and five seedlings per pot.
The percentage of uptake inhibition (% inhibition) can be calculated as:
Where C0 is TiO2 concentration (mg kg− 1) in whole plants without inhibitors, and C1 is TiO2 concentration (mg kg− 1) in whole plants with inhibitors.
Net Ionic Fluxes at Roots
Net fluxes of Cd2+, Na+, Ca2+, and Mg2+ were conducted using non-invasive micro-test technique (NMT, NMT100 Series, Younger USA, LLC) according to the procedures in previous study (Li et al. 2022). Before net ionic fluxes measured, 15-d-old wheat seedlings with or without pre-exposure to 10 mg L− 1 TiO2 NPs for 1 h were rinsed with deionized water and equilibrated with measuring solution for 30 min. Measurements were conducted on root surface 500 μm from the root apex (i.e., the meristematic zone). Steady-state ionic flux was recorded for 4 min and each measurement was repeated 4 − 6 times.
Cell Death in Root
After exposure to 10 mg L− 1 TiO2 NPs for 8 h, the roots were washed with Milli-Q water thoroughly and assessed for cell death. Root tips (5 mm in length from the tip) were stained in 0.25% (w/v) Evans blue for 30 min, and then rinsed with Milli-Q water (Zhang et al. 2019). Subsamples were photographed using a fluorescence microscope (Nikon-80i, Nikon, Japan). Other samples were used to quantify Evans blue. Briefly, dyed roots were homogenized in solution containing 50% (v/v) methanol and 1% (w/v) sodium dodecyl sulfate, incubated at 50 ℃ for 15 min and centrifuged at 10,000 g for 15 min. The optical density of the supernatant was determined at 600 nm using a UV spectrometer (UV, Shimadzu UV-2700, Japan).
Sample Analysis
Total Ti concentrations in the exposure medium were digested with 65% HNO3 overnight at room temperature and measured by inductively coupled plasma mass spectrometry (ICP-MS, Agilent 8800x, USA). Dissolved Ti concentration was defined as those passed through 3 kDa ultrafiltration filter (Amicon Ultra-15, Millipore).
Seedlings were rinsed with deionized water for 10 min, 1 mM HNO3 for 10 min, and then deionized water to remove adsorbed TiO2 NPs (Hu et al. 2020). They were dried at 70 ℃ and digested in a mixture of HNO3 and H2O2 at a ratio of 3:1 (v:v) at room temperature overnight. They were then digested in a heating block at 280 ℃ for an additional 6 h. Samples were analyzed for total Ti concentrations using ICP-MS. Digestion blanks, certified reference material for Ti (laver leaf, GBW10020, Chinese Academy of Geophysical Sciences, China) and calibration verification solution were included for quality assurance and quality control. The recovery of Ti averaged 86.6% ± 19.3%. NP uptake was expressed as TiO2 concentration.
Statistical Analysis
One-way analysis of variance (ANOVA) with Tukey’s test was used to test the differences among treatments (p < 0.05). Analysis was performed using SPSS 16.0. Data are presented as Mean ± SD.
Results and Discussion
Characteristics of TiO2 NPs
TiO2 NPs were spherical in exposure medium, with an average TEM diameter of 35.7 ± 1.2 nm (Fig. 1a-b). Their number-weighted hydrodynamic diameters were 60.8 ± 7.3 nm and relatively stable during the 8 h experiment (Fig. 1c, p > 0.05). Zeta potential and specific surface area of TiO2 NPs were − 13.8 ± 1.3 mV (pH 6.0) and 155.4 m2 g− 1, respectively. Dissolution of TiO2 NPs was less than 0.27‰ (Fig. 1d).
Characterization of TiO2 NPs in the exposure medium (10 mg L− 1 NPs, pH = 6). Representative TEM image of TiO2 NPs (a); size distribution of TiO2 NPs collected from TEM images (> 300 particles) (b); number-weighted hydrodynamic diameters (c) and the dissolution of TiO2 NPs (d) with or without inhibitors. Data are given as Mean ± SD (n = 3). CCCP: carbonyl cyanide m-chlorophenylhydrazone
TiO2 NP Uptake
Accumulated TiO2 in plants increased linearly and significantly over time (Fig. 2a, p < 0.05). The uptake rates of TiO2 NPs ranged from 119.0 to 604.2 mg kg− 1 h− 1, which were larger than those of AgNPs (8 − 39.0 mg kg− 1 h− 1) or AuNPs (4.4 − 20.8 mg kg− 1 h− 1) by wheat (Triticum aestivum spp.) under similar experimental conditions (Zhang et al. 2019). Collectively, the much higher uptake rates of TiO2 NPs confirms greater bioavailability of TiO2 NPs than AgNPs/AuNPs. Given the negligible dissolution of TiO2 NPs in the exposure medium (less than 0.27‰), TiO2 NPs were taken up as intact particles over 8 h exposure. In support of our statement, particulate uptake of TiO2 NPs by wheat (Triticum aestivum spp.) was documented by Laure et al. (2012), where NPs less than 140 nm could accumulate in wheat roots, and by Belhaj Abdallah et al. (2020), where particles less than 50 nm being accumulated in the roots of wild Dittrichia viscosa (accounting for 89% of total particles). Yet, the uptake mechanism for TiO2 NPs remains elusive in plant roots.
The accumulation of TiO2 NPs in wheat over time (a). Different letters indicate significant differences in TiO2 accumulation over time at p < 0.05. Effects of inhibitors on the uptake of TiO2 NPs by wheat seedlings (b). Different letters represent statistical differences among treatments at p < 0.05. Data are given as Mean ± SD (n = 3 or 4)
Particular Uptake Mechanism
Uptake of TiO2 NPs with Inhibitors
The addition of CCCP or Hg(NO3)2 generally did not affect the hydrodynamic diameters of TiO2 NPs and dissolution (Fig. 1c-d, p > 0.05). All inhibitors tended to reduce TiO2 concentrations in plant (Fig. 2b). Upon 1 h exposure, TiO2 concentration decreased by 65 ± 11% (p < 0.05), 77 ± 5% (p < 0.05) and 47 ± 6% (p < 0.05) in the presence of Hg(NO3)2, NaN3, and CCCP, compared to the control; after 8 h exposure, TiO2 concentration decreased by 85 ± 3% (p < 0.05), 83 ± 2% (p < 0.05) and 47 ± 21% (p > 0.05).
The decrease in TiO2 NP uptake in the presence of NaN3 or CCCP collectively suggests that TiO2 NP uptake is an energy-dependent active process. NaN3 was reported to reduce the ATP levels for the functioning of the cellular active transport and destroy the transmembrane proton gradient (Li et al. 2016), and CCCP was shown to cause a dissipation of the proton motive force across the membranes (Xu et al., 2007). Together with the observations of TiO2 NPs (2.8 ± 1.4 nm) in nuclei or vacuoles of root in Ardidopsis thaliana using X-ray fluorescence microscopy (Kurepa et al. 2010) and of TiO2 NPs (14 − 655 nm) in endosomes, vacuoles or in cell nuclei of root in wheat (Triticum aestivum L.) (Larue et al. 2012a), TiO2 NPs are likely to be internalized through the symplastic pathway. That is, NPs cross the cell membrane from cell to cell through plasmodesmata via endocytic pathways (the non-endocytic pathway aquaporins was excluded as shown below). Indirect evidence on the endocytic pathway could also derive from the size-selective internalization and translocation of TiO2 NPs (dimensions less than 50 nm) in wild Dittrichia viscosa (Abdallah et al., 2020), because endocytic pathway is selective on particle size (Schwab et al., 2016). In fact, endocytosis uptake of TiO2 NPs has been demonstrated in human lung cell lines (Tedja et al., 2012). Different from animal cells, plant cells are surrounded with cell wall with a pore diameter of 5 − 20 nm (Schwab et al., 2016). Increasing evidence has shown the endocytic uptake of gold nanoparticles, mesoporous silica nanoparticles, single-walled carbon nanotubes and cerium oxide nanoparticles in vitro (e.g., isolated tobacco protoplasts) or in planta in literature (Cui et al. 2020; Onelli et al. 2008; Shen et al. 2010; Zhao et al. 2012).
The difference in NP uptake inhibition between NaN3 and CCCP indicates that TiO2 NP uptake is a complex process that involves multiple energy-dependent pathways, which deserves further investigation. Because NaN3 or CCCP did not inhibit NP uptake completely (Fig. 2b), our results did not exclude the possibility of apoplastic pathway.
The 85% decline in TiO2 NP uptake with Hg(NO3)2 indicated that the uptake was associated with transpiration. Mercury ions can block water transport and aquaporin channel, whose diameters are 0.2 − 0.25 nm (Sattelmacher and Horst 2007). The uptake of TiO2 NPs of 36 nm was thus unlikely via aquaporin channels in this study (Fig. 1a-b). Thus, the decrease in TiO2 NP uptake was related to the reduced water flow through the plant when Hg(II) blocked water transport. Similarly, Asli and Neumann (2009) showed that TiO2 NPs (30 nm) physically inhibited transpiration in maize (Zea mays L.) via physical inhibition of apoplastic flow.
Cell Death in Root
Evans blue staining was used to detect the cell death in wheat root. Exposure to TiO2 NPs did not induce root cell death (Fig. 3a-b). Plasma membrane integrity of TiO2 NP-exposed roots was comparable to the control, and so was the uptake of Evans blue stain. Thus, the uptake of TiO2 NPs through physical injuries in root was unlikely.
Effects of TiO2 Uptake on net Ionic Fluxes
Net ionic fluxes in the meristematic zone of wheat roots were further examined using the real-time non-invasive micro-test technique (NMT). Four types of ionic fluxes were investigated, including net Cd2+, Na+, Ca2+, and Mg2+ fluxes (Fig. 3c-d). These ionic channels include divalent and monovalent uptake systems, which are likely to play important roles in ion acquisition and ionic homeostasis. Unexposed roots had net fluxes of Cd2+, Na+, Ca2+ and Mg2+ of − 11.0 ± 1.0, − 3.6 ± 3.3, − 4.5 ± 4.8 and − 25.3 ± 22.3 pmol cm− 2 s− 1, respectively. However, exposed roots had net fluxes of Cd2+, Na+, Ca2+ and Mg2+ of − 2.1 ± 0.5, 24.7 ± 6.2, − 1.1 ± 1.8, and − 16.2 ± 16.8 pmol cm− 2 s− 1. A negative value represents net ions influx, while the positive value represents net ions outflow. Generally, unexposed roots had a net influx of these cations. TiO2 NP exposure decreased the influx of Cd2+, and tend to decline the influx of Mg2+ and Ca2+. However, Na+ flux shifted from inflow to outflow.
Fluorescence microscope images of wheat roots upon 8 h exposure to 10 mg L− 1 TiO2 NPs (a) and Evans blue uptake into wheat roots (% of the control) (b). No significant difference between treatments was observed. Effects of TiO2 NPs on net fluxes of Cd2+, Na+, Ca2+, and Mg2+ at the meristematic zone (c, d). The mean value of ion fluxes in wheat seedling roots, using the mean value of 4 independent measurements (mean ± SD; n = 4). Significant difference between treatments is denoted at **p < 0.01, *p < 0.05
Our results provided direct evidence of the decrease in Cd2+, Ca2+and Mg2+ influx in plants in the presence of TiO2 NPs. The lower influx of Cd2+, Ca2+and Mg2+ could not be explained by their adsorption on TiO2 NPs in the exposure medium because the roots were pre-exposed to TiO2 NPs and, consequently, to these metals in the absence of TiO2 NPs. Rather, it is highly possible that the uptake of TiO2 NPs may lead to the blocking of root pores and inhibit the apoplastic flow of water and micronutrients (Asli and Neumann 2009; Lin and Xing 2008). This was supported by the findings that TiO2 NP accumulation in maize (Zea mays L.) was accompanied by a reduction in the cell wall pore diameter (Asli and Neumann 2009). Alternatively, NP exposure may downregulate the genes and microRNAs (miRNAs) for metal transporter (Ruotolo et al. 2018; Dai et al. 2021), and thus reduce the cation influxes. Nevertheless, previous studies have shown that TiO2 NPs significantly decreased the contents of Mg in lettuce roots and shoots by 23 − 27% and 7 − 27%, respectively, in hydroponics, due to the adsorption of Mg2+ on TiO2 NPs in the exposure medium (Hu et al., 2020). Our results further suggest that the decline in Mg2+ influx rate was responsible.
TiO2 NP exposure also shifted the trend of Na+ flux, from inflow to outflow. Low concentration of TiO2 NPs could increase the tolerance to salinity by inducing miRNAs expression in tobacco (Nicotiana tabacum) seedlings (Frazier et al. 2014). The pre-exposure to TiO2 NPs may increase the salinity tolerance of wheat, resulting in an active efflux of Na+ from wheat root epidermal cells (Cuin et al. 2011), and led to a decrease in Na concentrations over a long term. Indeed, Hu et al. (2020) showed that TiO2 NP exposure in hydroponics decreased Na contents in lettuce root and leaves by 9.5% and by 7 − 15% after one week, respectively. Sodium is a functional nutrient regulating stomatal opening and closing. As a result, TiO2 NP (15 nm) exposure led to a decrease in stomatal conductance of Oryza sativa (Costa and Sharma 2015).
Overall, our results suggested that TiO2 NPs accumulate in wheat seedlings (Triticum aestivum L.) as intact particles within 8 h exposure. The uptake was unlikely through the physical injury in roots. NaN3 and CCCP inhibited TiO2 NP uptake, indicating energy-dependent uptake pathways. Accompanied with TiO2 NP uptake, metal-specific flux in the roots was observed, which reduced the influx of Cd2+ and Mg2+ significantly, but not significantly of Ca2+. Meanwhile, the pre-exposure to TiO2 NPs shift the Na+ flux from influx to efflux within plants. The findings from this study provide valuable information for understanding plant uptake of TiO2 NPs.
References
Asli S, Neumann PM (2009) Colloidal suspensions of clay or titanium dioxide nanoparticles can inhibit leaf growth and transpiration via physical effects on root water transport. Plant Cell Environ 32:577–584. doi:https://doi.org/10.1111/j.1365-3040.2009.01952.x
Belhaj Abdallah B, Andreu I, Chatti A, Landoulsi A, Gates BD (2020) Size fractionation of titania nanoparticles in wild Dittrichia viscosa grown in a native environment. Environ Sci Technol 54:8649–8657. doi:https://doi.org/10.1021/acs.est.9b07267
Chahardoli A, Sharifan H, Karimi N, Kakavand SN (2022) Uptake, translocation, phytotoxicity, and hormetic effects of titanium dioxide nanoparticles (TiO2 NPs) in Nigella arvensis L. Sci Total Environ 806. doi:https://doi.org/10.1016/j.scitotenv.2021.151222
Costa M, Sharma PK (2015) Influence of titanium dioxide nanoparticles on the photosynthetic and biochemical processes in oryza sativa. Int J Recent Sci Res 6:2445–2451
Cui J, Li Y, Jin Q, Li F (2020) Silica nanoparticles inhibit arsenic uptake into rice suspension cells via improving pectin synthesis and the mechanical force of the cell wall. Environ Sci: Nano 7:162–171. doi:https://doi.org/10.1039/c9en01035a
Cuin TA, Bose J, Stefano G, Jha D, Tester M, Mancuso S, Shabala S (2011) Assessing the role of root plasma membrane and tonoplast Na+/H+ exchangers in salinity tolerance in wheat: in planta quantification methods. Plant Cell Environ 34:947–961. doi:https://doi.org/10.1111/j.1365-3040.2011.02296.x
Dai S et al (2021) Astaxanthin and its gold nanoparticles mitigate cadmium toxicity in rice by inhibiting cadmium translocation and uptake. Sci Total Environ 786. doi: https://doi.org/10.1016/j.scitotenv.2021.147496
Farooq MA et al (2022) The potential of nanomaterials for sustainable modern agriculture: present findings and future perspectives. Environ Sci Nano 9:1926–1951. doi:https://doi.org/10.1039/d1en01124c
Frazier TP, Burklew CE, Zhang BH (2014) Titanium dioxide nanoparticles affect the growth and microRNA expression of tobacco (Nicotiana tabacum). Funct Integr Genomics 14:75–83. doi:https://doi.org/10.1007/s10142-013-0341-4
Hochella MF et al (2019) Natural, incidental, and engineered nanomaterials and their impacts on the Earth system. Science 363:1414–1414. doi: https://doi.org/10.1126/science.aau8299
Hu J et al (2020) TiO2 nanoparticle exposure on lettuce (Lactuca sativa L.): dose-dependent deterioration of nutritional quality. Environ Sci Nano 7:501–513. doi:https://doi.org/10.1039/c9en01215j
Javot H, Maurel C (2002) The role of aquaporins in root water uptake. Ann Bot 90:301–313. doi: https://doi.org/10.1093/aob/mcf199
Ji Y et al (2017) Jointed toxicity of TiO2 NPs and cd to rice seedlings: NPs alleviated cd toxicity and cd promoted NPs uptake. Plant Physiol Biochem 110:82–93. doi:https://doi.org/10.1016/j.plaphy.2016.05.010
Kabata-Pendias A, Mukherjee AB (2007)Humans. Springer
Kurepa J et al (2010) Uptake and distribution of ultrasmall anatase TiO2 alizarin red S nanoconjugates in Arabidopsis thaliana. Nano Lett 10:2296–2302. doi:https://doi.org/10.1021/nl903518f
Larue C et al (2012a) Accumulation, translocation and impact of TiO2 nanoparticles in wheat (Triticum aestivum spp.): influence of diameter and crystal phase. Sci Total Environ 431:197–208. doi:https://doi.org/10.1016/j.scitotenv.2012.04.073
Larue C, Veronesi G, Flank AM, Surble S, Herlin-Boime N, Carriere M (2012b) Comparative uptake and impact of TiO2 nanoparticles in wheat and rapeseed. J Toxicol Environ Health A 75:722–734. doi:https://doi.org/10.1080/15287394.2012.689800
Li H, Ye X, Guo X, Geng Z, Wang G (2016) Effects of surface ligands on the uptake and transport of gold nanoparticles in rice and tomato. J Hazard Mater 314:188–196. doi:https://doi.org/10.1016/j.jhazmat.2016.04.043
Lin DH, Xing BS (2008) Root uptake and phytotoxicity of ZnO nanoparticles. Environ Sci Technol 42:5580–5585. doi:https://doi.org/10.1021/es800422x
Li YJ et al (2022) Calcium-mobilizing properties of Salvia miltiorrhiza-derived carbon dots confer enhanced environmental adaptability in plants. ACS Nano 16:4357–4370. doi:https://doi.org/10.1021/acsnano.1c10556
Ma C, White JC, Dhankher OP, Xing B (2015) Metal-based nanotoxicity and detoxification pathways in higher plants. Environ Sci Technol 49:7109–7122. doi:https://doi.org/10.1021/acs.est.5b00685
Onelli E, Prescianotto-Baschong C, Caccianiga M, Moscatelli A (2008) Clathrin-dependent and independent endocytic pathways in tobacco protoplasts revealed by labelling with charged nanogold. J Exp Bot 59:3051–3068. doi:https://doi.org/10.1093/jxb/ern154
Ren HX et al (2011) Physiological investigation of magnetic iron oxide nanoparticles towards chinese mung bean. J Biomed Nanotechnol 7:677–684. doi:https://doi.org/10.1166/jbn.2011.1338
Ruotolo R, Maestri E, Pagano L, Marmiroli M, White JC, Marmiroli N (2018) Plant response to metal-containing engineered nanomaterials: an omics-based perspective. Environ Sci Technol 52:2451–2467. doi:https://doi.org/10.1021/acs.est.7b04121
Sattelmacher B, Horst WJ (2007) The apoplast of higher plants: compartment of storage, transport and reactions: the significance of the apoplast for the mineral nutrition of higher plants. Springer
Schwab F, Zhai G, Kern M, Turner A, Schnoor JL, Wiesner MR (2016) Barriers, pathways and processes for uptake, translocation and accumulation of nanomaterials in plants - critical review. Nanotoxicology 10:257–278. doi: https://doi.org/10.3109/17435390.2015.1048326
Servin AD, Castillo-Michel H, Hernandez-Viezcas JA, Diaz BC, Peralta-Videa JR, Gardea-Torresdey JL (2012) Synchrotron micro-XRF and micro-XANES confirmation of the uptake and translocation of TiO2 nanoparticles in cucumber (Cucumis sativus) plants. Environ Sci Technol 46:7637–7643. doi:https://doi.org/10.1021/es300955b
Shen CX, Zhang QF, Li J, Bi FC, Yao N (2010) Induction of programmed cell death in Arabidopsis and rice by single-wall carbon nanotubes. Am J Bot 97:1602–1609. doi:https://doi.org/10.3732/ajb.1000073
Tedja R, Lim M, Amal R, Marquis C (2012) Effects of serum adsorption on cellular uptake profile and consequent impact of titanium dioxide nanoparticles on human lung cell lines. ACS Nano 6:4083–4093. doi: https://doi.org/10.1021/nn3004845
Xu X, McGrath SP, Zhao F (2007) Rapid reduction of arsenate in the medium mediated by plant roots. New Phytol 176:590–599. doi: https://doi.org/10.1111/j.1469-8137.2007.02195.x
Zhang WY, Wang Q, Li M, Dang F, Zhou DM (2019) Nonselective uptake of silver and gold nanoparticles by wheat. Nanotoxicology 13:1073–1086. doi:https://doi.org/10.1080/17435390.2019.1640909
Zhao L et al (2012) Effect of surface coating and organic matter on the uptake of CeO2 NPs by corn plants grown in soil: insight into the uptake mechanism. J Hazard Mater 225–226:131–138. doi:https://doi.org/10.1016/j.jhazmat.2012.05.008
Acknowledgements
This work was supported by the National Natural Science Foundation of China (41701580).
Author information
Authors and Affiliations
Corresponding authors
Additional information
Publisher’s Note
Springer Nature remains neutral with regard to jurisdictional claims in published maps and institutional affiliations.
Rights and permissions
Springer Nature or its licensor (e.g. a society or other partner) holds exclusive rights to this article under a publishing agreement with the author(s) or other rightsholder(s); author self-archiving of the accepted manuscript version of this article is solely governed by the terms of such publishing agreement and applicable law.
About this article
Cite this article
Yan, H., Zhang, W., Li, C. et al. Uptake of TiO2 Nanoparticles was Linked to Variation in net Cation flux in Wheat Seedlings. Bull Environ Contam Toxicol 110, 71 (2023). https://doi.org/10.1007/s00128-022-03665-6
Received:
Accepted:
Published:
DOI: https://doi.org/10.1007/s00128-022-03665-6