Abstract
Sonophoresis uses ultrasound as a physical enhancer for systemic transdermal drug delivery (TDD). Low-frequency sonophoresis in the range of 20–100 kHz has been demonstrated to enhance the transdermal delivery of various low-molecular weight drugs and high-molecular weight proteins across the human skin. The bioeffects of ultrasound in tissues are mediated by thermal and nonthermal effects. Ultrasound-induced skin heating causes fluidization of stratum corneum (SC) lipids, facilitating transdermal permeation of molecules. Cavitation is the nonthermal effect of ultrasound and is believed to be the main mechanism of enhanced transdermal delivery in sonophoresis, by creating shock waves and acoustic micro-jets on the SC surface. Among the three different percutaneous penetration pathways including the intercellular, transcellular, and follicular penetration routes, both intercellular and transcellular pathways are primarily created and facilitated during ultrasound exposure for TDD. Sonophoresis may act synergistically with various other physical and chemical penetration enhancement methods in promoting transdermal drug delivery. The ratio of frequency and peak rare-fractional pressure, the distance between the surface of transducer and the skin surface, and the properties of the coupling medium such as the viscosity, density, acoustic impedance and the composition of the gas and liquid phases are factors affecting the efficacy of TDD using ultrasound. Sonophoresis has been widely used for TDD, such as transcutaneous immunization and gene therapy and as a noninvasive transdermal monitoring method. In addition, sonophoresis represents a safe and effective method for TDD.
Access provided by CONRICYT-eBooks. Download chapter PDF
Similar content being viewed by others
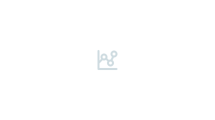
Keywords
1 Introduction
Sonophoresis uses ultrasound as a physical enhancer for systematic transdermal drug delivery (TDD). Historically, sonophoresis was first reported in 1950s along with other therapeutic ultrasound applications such as noninvasive surgical treatment of neurological disorders including Parkinson disease (Fry 1954; Fry et al. 1954, 1958). According to Fellinger and Schmidt, hydrocortisone ointment applied with ultrasound substantially improved the treatment of polyarthritis of the digital joints (Fellinger et al. 1954). Griffin et al. had published a series of sonophoresis experimental results for various applications (mostly pain-releasing application) of hydrocortisone with the aid of ultrasound in the 1960s (Griffin 1966; Griffin et al. 1967; Griffin and Touchstone 1968). Later in the1980s, McElnay et al. and Benson et al. showed that sonophoresis could be used for the application of local anesthetics (McElnay et al. 1985, 1987; Benson et al. 1988, 1991). Although these early reports had shown the application possibilities of sonophoresis, the effect of sonophoresis was still smaller than expected, and there was lack of rigorous physical and engineering approaches to improve sonophoresis in its early days.
Systemic research regarding the core parameters in sonophoresis, such as frequency, intensity, and duty cycles of the pulsed ultrasound, started in the 1990s, and the reported therapeutic efficacy of sonophoresis increased drastically during this period. Bommannan et al. hypothesized thermal effect of ultrasound would be the main mechanism of sonophoresis and conducted the experiments with 2–16 MHz ultrasound (Bommannan et al. 1992a, b). As they expected, 2 MHz ultrasound did not significantly enhance the amount of salicylic acid penetrated into pig skin, while 10 MHz ultrasound increased the delivery of salicylic acid fourfold with the same used intensity of 0.2 W/cm2. This frequency dependency could be linked to the higher absorption of ultrasonic energy in tissue as the frequency increases (Szabo 2004). On the other hand, Tachibana et al. and Mitragotri et al. have demonstrated that low-frequency ultrasound (~100 kHz) is effective in transdermal delivery of various therapeutic compounds (Tachibana and Tachibana 1991, 1993; Tachibana 1992; Mitragotri et al. 1995a, b, 1996).
Interestingly, low-frequency sonophoresis has shown much higher increase of skin permeability compared to that of sonophoresis with MHz range ultrasound. In some cases, the amount of transported permeants could be increased up to 3000 times with low-frequency sonophoresis compared to control permeability without sonophoresis treatment (Mitragotri et al. 1996). These intriguing reports brought worldwide attention to low-frequency sonophoresis, and numerous related studies have been conducted.
Sonophoresis has several characteristic properties compared to other physical TDD methods such as iontophoresis, electroporation, and microneedles. The first is that the electrical properties of the permeant are not significant factors in sonophoresis. Stratum corneum (SC) is a stiff barrier to foreign substances in general and charged molecules experience more difficulty to cross SC than neutral molecules, and therefore liposomes have been studied to encapsulate charged drugs (Cevc et al. 1995). However, sonophoresis can be used for both hydrophilic and lipophilic permeants effectively (Mitragotri et al. 1995a, b; Boucaud et al. 2001; Smith 2007). The second property is that sonophoresis can be a pretreatment method or a simultaneous delivery method with solutes. Even though Meidan et al. reported that the effect of sonophoresis rapidly decreased to normal with MHz range ultrasound (Meidan et al. 1998), most of recent low-frequency sonophoresis studies showed that the increased skin permeability sustains up to 48 h under certain conditions such as occlusion (Mitragotri et al. 1995a, b; Le et al. 2000; Lavon and Kost 2004; Gupta and Prausnitz 2009; Kim do et al. 2012). The third property is that sonophoresis can be combined with other physical TDD methods relatively easily. The sustained skin permeability provides time window of additional TDD methods, such as iontophoresis, electroporation, and chemical penetration enhancers (Le et al. 2000; Gupta and Prausnitz 2009; Kim do et al. 2012; Kost et al. 1996; Johnson et al. 1996; Mitragotri et al. 2000a; Mitragotri 2000; Tezel et al. 2002a; Dahlan et al. 2009a; Schroeder et al. 2009). Additionally, ultrasound transducers, which are the source of mechanical wave generation, can generally be manufactured to be chemically and electrically resistant in various shapes, so that simultaneous application of other methods with sonophoresis can also be achieved relatively easily (Maione et al. 2002; Park et al. 2007; Fiorillo et al. 2012). The last property characteristic for sonophoresis is that frequency is a critical factor in sonophoresis. Low-frequency sonophoresis (~100 kHz) induces a significant increase of the skin permeability compared to higher-frequency (1–20 MHz) sonophoresis (Mitragotri et al. 1995a, 1996; Smith 2007; Mitragotri 2000; Mitragotri et al. 2000c; Tezel et al. 2001, 2002b; Terahara et al. 2002; Al-Bataineh et al. 2011). Due to this fact, the main mechanism of sonophoresis is believed to be cavitation.
In this chapter, we will focus on the mechanisms which can explain the sonophoresis features mentioned above. The effects of ultrasound in tissue can be classified into two categories: thermal effects and nonthermal effects including cavitation and acoustic streaming (Wang et al. 2005). Thermal and cavitational factors will be discussed from the scientific viewpoint. In addition, the delivery pathway will be covered based on the current research evidences. Various applications of sonophoresis with commercially available equipment and safety issue will follow at the end of the chapter.
2 Mechanism of Sonophoresis
2.1 Thermal Effect
During sonication, ultrasound energy is partially attenuated into the tissue. The energy attenuation of ultrasound depends on absorption and scattering in each tissue (Nyborg 2001). Absorption of energy by tissue causes a local temperature increase, which depends upon ultrasound frequency, intensity, area of the ultrasound beam, duration of exposure, and the rate of heat removal from the target by blood flow or conduction (Merritt et al. 1992). The temperature increase in the skin may increase the permeability coefficient due to an increase of the diffusion coefficient. Merino et al. reported enhanced transdermal permeability of mannitol caused by this temperature increase (Merino et al. 2003). The skin temperature was increased for 20 °C with low-frequency ultrasound (20 kHz), and the transdermal mannitol delivery determined using the glucose dehydrogenase-NADPH coupled assay was enhanced 35-fold compared to that of passive control. However, only 25 % of this improvement in transdermal mannitol delivery was attributed to the increased temperature induced by ultrasound (Merino et al. 2003). This contradictory experimental result indicates that thermal energy alone may not play a significant role in promoting TDD, even though the temperature increase may affect the skin permeability. Skin heating by ultrasound increases transdermal transport by fluidizing SC lipids and/or increasing convective flow (Wang et al. 2005).
2.2 Cavitation Effect
Cavitation is defined as the creation of a new surface or expansion, contraction, and distortion of preexisting gaseous bubbles in a liquid medium (Leighton 1997). Acoustic cavitation occurs due to the nucleation of small gaseous cavities during the negative pressure cycles of ultrasound, followed by the growth of these bubbles throughout subsequent pressure cycles. Since cavitation nuclei are random in size, type, and shape in biological environments, cavitation is a stochastic event. Cavitation can be further classified into two categories according to activity of gaseous bubbles related to the acoustic field, namely, stable cavitation and inertial cavitation (Mitragotri et al. 1995a; Leighton 1997).
2.2.1 Stable Cavitation
Stable cavitation corresponds to a continuous oscillation of bubbles about the equilibrium radius in response to lower positive and negative pressures in an acoustic field (less than ~1 MPa with an inverse dependence on frequency) (Carvell and Bigelow 2011). Oscillation of bubbles around asymmetric boundary conditions by stable cavitation leads to microstreaming, which can generate high-velocity gradients and hydrodynamic shear stresses (Mitragotri et al. 1996). Microstreaming is the unidirectional flow of fluid along cell membranes in response to bubble dynamics in an acoustic field. The velocity of local fluid is determined by acoustic properties (acoustic attenuation, speed of sound) associated with properties of the fluid such as viscosity and density, as well as by the temporal average intensity, frequency, transducer aperture, and pressure amplitude (Nightingale et al. 1999). The velocity of acoustic streaming decreases with increasing fluid viscosity and increases with increasing attenuation (Barnett et al. 1994). Collis et al. studied several patterns of microstreaming, showing that many microstreaming patterns are possible around a microbubble on a surface. Each microstreaming pattern also generated different shear stresses with distributions of compression and stretch in the vicinity of a bubble on a surface (Collis et al. 2010). The states of uniform shear stress and two types of divergence are illustrated in Fig. 2.1.
Illustration of the difference between uniform shear and surface divergence. (a) Uniform, high-shear stress, no divergence, and thus no stretch of the cell surface; (b) high rate of positive divergence (as well as shear stress), cell/cell membrane in stretch-activated state; (c) high rate of negative divergence (as well as shear stress), cell/cell membrane compressed together (This figure is reproduced with permission from Collis et al. 2010)
Bubbles can also grow slowly due to asymmetric gas flow during stable cavitation, and this interesting phenomenon is called rectified diffusion. While a bubble is expanded in the ultrasound field, the surface area and the internal volume of the bubble increase, but thickness of bubble shell decreases. Due to the increased volume, the internal gas density will decrease so that the dissolved gas in surrounding liquid can flow into the bubble. The opposite directional gas flow can happen during the period of compressional ultrasound field. However, the inflow and the outflow of gas are asymmetric since the surface area and the gas pressure gradient at each stage are different. In other words, the surface area of bubble and the gas pressure gradient are greatest at the peak of expansion, so that gas inflow will be maximum. But outflow will be much smaller due to the minimized surface area and low gas pressure gradient due to thickened bubble shell. Harvey et al. suggested rectified diffusion during the study of the formation of bubbles in animals, and Crum estimated the minimally required external pressure to be 0.01 MPa in water (Harvey et al. 1944; Crum 1984). Lavon et al. suggested that rectified diffusion in intercellular lipid layers can be the main mechanism of sonophoresis (Lavon et al. 2007).
2.2.2 Inertial Cavitation
Inertial cavitation corresponds to the violent growth and collapse of bubbles that can occur within a period of a single cycle or a few cycles, depending on acoustic pressure as well as frequency and size distribution of bubbles associated with resonant frequency (Mitragotri et al. 1996; Suslick 1989; Colussi et al. 1998). Apfel and Holland derived a mechanical index (MI), which represents the likelihood that inertial cavitation will occur for medical ultrasound imaging systems (Apfel et al. 1991). The organizations such as the American Institute of Ultrasound in Medicine (AIUM), National Electrical Manufacturers Association (NEMA), and Food and Drug Administration (FDA) have adopted an MI, weighted for the frequency response, of \( {I}_{\mathrm{MI}}={P}_{\mathrm{neg}}\left[\mathrm{Mpa}\right]/\sqrt{f_0}\left[\mathrm{MHz}\right] \) (P neg, maximum negative pressure, f 0, frequency). The likelihood of inertial cavitation increases with decreasing frequency and lowering peak-negative pressure (Meltzer 1996). For inertial cavitation, lower frequencies give bubbles more time to grow in the expansion cycle (the rapid expansion of gaseous bubbles) and consequently produce more violent collapse during the compression cycle (the collapse of gaseous bubbles). Violent collapse of cavitation bubbles might either generate shock waves in the bulk of the liquid or a micro-jet which is from the asymmetric collapse of bubbles inducing fissures on membranes near a boundary. Figure 2.2 schematically shows the shock wave and micro-jet produced by inertial cavitation events. A spherical collapse of a bubble yields high-pressure cores that emit shock waves with amplitudes exceeding 10 kbar (Pecha and Gompf 2000). The disruption of a target exposed to such a pressure wave may occur through relative particle displacement, compressive failure, tensile stress, or shear strain (Lokhandwalla and Sturtevant 2000). When a bubble collapses asymmetrically near a boundary, it generally produces a well-defined high-velocity micro-jet (Katz 1995; Popinet and Zaleski 2002). Micro-jet distortion of bubble collapse depends on the surface of the bubble encounters. If the surface is larger than the resonant size of the bubble (diameter of 1 ~ 100um at 20 ~ 500 kHz (Leighton 1997)), the resulting collapse will be in micro-jet form (see Fig. 2.2) (Tezel et al. 2003).
Three possible modes through which inertial cavitation may enhance SC permeability: (a) spherical collapse near the SC surface emits shock waves, (b) impact of an acoustic micro-jet on the SC surface, and (c) micro-jet physically penetrates into the SC (The figure is reproduced with permission from Tezel et al. 2003)
Shock waves generated by inertial cavitation can cause structural alteration in the surrounding corneocyte lipid interface regions. Because channels for diffusion could be newly formed between keratinocyte-lipid interfaces, drugs can be delivered through these aqueous channels which are formed within the disordered lipid bilayers of SC. Furthermore, the impact pressure of the micro-jet on the skin surface may enhance SC permeability by disrupting SC lipid bilayers (Lee et al. 1998). A micro-jet possessing a radius about one-tenth of the maximum bubble diameter impacts the SC surface without penetrating into it. The impact pressure of the micro-jet on the skin surface may enhance SC permeability by disrupting SC lipid bilayers (Lee et al. 1998). When combined, these factors such as shock waves and micro-jet lead to the disordering of the lipid bilayers and formation of aqueous channels in the skin through which drugs can permeate (Bommannan et al. 1992a).
3 Penetration Pathways Induced by Sonophoresis
Cavitation nuclei and air pockets can be formed in the intracellular and/or intercellular structures upon application of ultrasound. In addition, bubbles can be formed on the skin and in the coupling medium on the skin surface. All of these bubbles can be a source of the local inertial and stable cavitation under an ultrasonic field.
3.1 Pathways Through Hair Follicles
In addition to the transcellular and intercellular pathways, hair follicle brought attention in TDD as a possible pathway. Although the area occupied by hair follicles is only 0.1 % or less of the total skin surface, the hair follicles appear to play a critical role in passive drug diffusion (Scheuplein et al. 1969; Scheuplein 1967). However, Sarheed and Frum reported that when the hydrocortisone was passively absorbed into the skin, 46 % of absorption involved the drug penetrating into the follicles; however, as the duration of sonication increased, the follicular contribution fell to zero even though total transepidermal flux dramatically increased (Sarheed and Frum 2012). Hence, hair follicles may not be the main pathway in sonophoresis.
3.2 Penetration Pathways Through the Stratum Corneum
If we rule out hair follicles as a penetration pathway, there are two other possible pathways: the intercellular route and the transcellular route (see Fig. 2.3). Hydrophilic substances can easily diffuse into the corneocytes and permeate the skin through the transcellular route; however, SC lipid bilayers act as the main barrier for the permeation of these substances (Mitragotri et al. 1995b; Boucaud et al. 2001; Smith 2007; Tezel et al. 2002b; Crum 1984; Tezel et al. 2003). Alvarez-Román et al. have shown that sonophoresis leads to lipid extraction in the SC, i.e., they found that approximately 30 % of lipid can be removed during sonophoresis (Alvarez-Román et al. 2003). Under this assumption, a number of aqueous porous membrane models were suggested and modified to explain the intercellular pathway (Edwards and Langer 1994; Mitragotri 2001; Tezel et al. 2003; Mitragotri and Kost 2004; Paliwal et al. 2006; Polat et al. 2011a, b). Based on these models, Tezel et al. (2003) implied that the increase of porosity of the SC, leading to an enhanced skin permeability, is also responsible for the penetration enhancement induced by sonophoresis. Lavon et al. suggested that the growth of bubble due to rectified diffusion can be the reason of increased porosity (Lavon et al. 2007). Several researchers also paid attention to the heterogeneous nature of the skin where sonophoresis was applied uniformly (Kushner et al. 2008a; Polat et al. 2011a). The spots which are called localized transport regions (LTRs) can be observed on the completion of sonophoresis, and LTRs are believed to be the localized channel opening of a tortuous intercellular route.
Schematic illustrating representation of the transport penetration pathways during (a) passive transport and (b) low-frequency sonophoresis (The figure is reproduced with permission from Mitragotri et al. 1996)
In summary, some researchers claim that stable cavitation inside the epidermis may cause the growth of lacuna and create larger space to be transport channels (Paliwal et al. 2006). Other researchers believe that multiple events of inertial cavitation on the surface of the skin and inside the epidermis lead to the formation of shock waves, which then microscopically disrupt the lipid bilayers and eventually create pathways through the tortuous intercellular lipid layers with causing significant structural changes in the epidermis.
3.3 Transcellular Pathways
Micro-jet can be formed on the epidermis surface. Micro-jets can be strong enough to create pits on aluminum surface and biological tissue. Lee et al. have observed 1–2 μm size pits on the epidermis on the completion of sonophoresis lasting for 5 min (Lee et al. 2010). Based on the visualized evidence and the micro-jet idea, a direct channel formation through transcellular region can be hypothesized. Cavitation on the skin surface is critical in this hypothesis, and the other research results support that transient cavitation outside the skin is the key mechanism responsible for LFS-induced skin permeabilization (Tang et al. 2002; Wolloch and Kost 2010) and the observation of LTRs can be also coped with this hypothesis as well.
Based on the evidence, both intercellular pathway and transcellular pathway appear to be plausible, but the major transport pathway is through the intercellular lipid domains. Further research will be required to clarify the main penetration pathways during sonophoresis-induced TDD.
4 Synergistic Effect of Low-Frequency Sonophoresis and Other Enhancers for Transdermal Drug Delivery
Previous studies have demonstrated that sonophoresis may act synergistically with other penetration enhancement methods on transdermal drug delivery (Mitragotri and Kost 2004). Low-frequency sonophoresis acts synergistically with various chemical penetration enhancers. Mitragotri et al. (Mitragotri et al. 2000a) reported that sodium lauryl sulfate enhanced skin permeability to mannitol synergistically in combination with ultrasound. Johnson et al. (Johnson et al. 1996) reported that combined application of linoleic acid and ethanol with ultrasound increased corticosteroid flux by up to 13,000-fold compared with passive flux, which was higher than that induced by each treatment alone. The proposed mechanism for these synergistic effects of ultrasound and chemical penetration enhancers on TDD is that the cavitation produced by ultrasound may induce mixing and dispersion of the chemical enhancer with SC lipids (Johnson et al. 1996). In addition, ultrasound combined with iontophoresis has been shown to have synergistic effect on enhancing heparin flux though the skin (Le et al. 2000). The possible mechanism for the synergistic effect of ultrasound and iontophoresis for transdermal drug delivery includes the decrease of skin’s impedance and size selectivity of the skin due to ultrasound-induced structural changes in the skin (Le et al. 2000). Ultrasound has been demonstrated to have synergistic effect for transdermal drug delivery when combined with electroporation. Kost et al. (1996) has reported that simultaneous application of ultrasound and electroporation enhanced transdermal delivery of two molecules, calcein and sulforhodamine. The possible mechanism for this synergistic effect of ultrasound and electroporation on transdermal drug delivery might be reduced skin impedance, enhanced convection, and reduced size selectivity of the skin (Le et al. 2000; Kost et al. 1996).
5 Factors Affecting Drug Delivery by Low-Frequency Sonophoresis
As indicated in the section regarding to the mechanism, cavitation appears to be the main mechanism in sonophoresis. Accordingly, the factors facilitating cavitation will be the key factors affecting drug delivery. The first factor is the ratio of frequency and peak rare-fractional pressure (also called peak-negative pressure) as explained in mechanical index (MI). Since low-frequency and high rare-fractional pressure increase MI, the inertial cavitation is more likely to occur at low-frequency sonophoresis. When the frequency is low enough, the given acoustic field can be assumed to be a quasi-static field. If small bubbles can rapidly grow and collapse with exposure to quasi-static rare-fractional pressure, these bubble activities can be related to inertia cavitation or asymmetric bubble collapse. Low-frequency sonophoresis has thus been the topic of extensive research over the last 20 years. The effects of low-frequency sonophoresis have been studied by Mitragotri et al. (Mitragotri et al. 1995a, 1996) to enhance the transport of various low-molecular weight drugs (including aldosterone, corticosterone, estradiol, histamine, mannitol, salicylic acid, and sucrose) as well as high-molecular weight drugs (such as heparin and insulin). They also found that the enhancement ratio induced by low-frequency sonophoresis (20 kHz) is 1000-fold higher than that induced by therapeutic sonophoresis (1–3 MHz). The results of several studies suggest that the greater efficiency of low-frequency sonophoresis compared with high-frequency sonophoresis originates from the increased incidence of cavitation events (Mitragotri et al. 1996; Tezel et al. 2001). The distance between the surface of transducer and the skin surface was also mentioned as a factor influencing the TDD by ultrasound in some articles (Terahara et al. 2002). However, the peak-negative pressure decreases as the distance increases, and hence the possibility of cavitation decreases accordingly. More importantly, the occurring level of cavitation could be the second factor influencing the efficacy of the TDD by ultrasound, since cavitation bubbles nucleating and collapsing on the SC surface are likely to make a significant contribution to permeabilization of the SC due to their proximity (Tang et al. 2002; Tezel et al. 2003). Tezel et al. (2003) suggested that only cavitation which occurs within 50 μm from the skin surface could effectively increase skin permeability. The third and the fourth factor affecting the efficacy of the TDD by ultrasound are the viscosity and the composition of the gas and liquid phases of drug where permeants are dissolved. In viscous medium, higher pressure is required to cause inertial cavitation since the viscous medium absolves mechanical energy by damping (Tang et al. 2002; Popinet and Zaleski 2002). On the other hand, solute highly saturated with gas can experience cavitation with small ultrasonic perturbation since small gas bubbles can act as cavitation nuclei (Ueda et al. 2009). In recent studies, Park et al. utilized even the ultrasound contrast agents, which are engineered microbubbles of a certain size distribution, to increase cavitation induction (Park et al. 2010; Polat et al. 2011b). Further studies will be needed to draw a definite conclusion regarding this factor (Cagnie et al. 2003; Sarheed and Abdul Rasool 2011; Herwadkar et al. 2012).
6 Therapeutic Application of Sonophoresis
Various applications of sonophoresis in TDD are summarized in Table 2.1.
Since the initial treatment of polyarthritis using transdermal delivery of hydrocortisone ointment in the 1950s, the transdermal delivery of therapeutic drugs such as fentanyl, caffeine, heparin, ketoprofen, and insulin has been a major concern for clinical treatment. Boucaud et al. evaluated the effects of sonophoresis at 20 kHz with 2.5 W/cm2 on transdermal transport of fentanyl and caffeine across both hairless rat and human skin (Boucaud et al. 2001). Fentanyl is generally used to relieve pain for surgery or for cancer patients, while caffeine is the most common stimulant used in the treatment of lipodystrophy (Dias et al. 1999; Paix et al. 1995). The results showed that sonophoresis enhanced the TDD of both fentanyl (about 35-fold greater than controls without sonophoresis) and caffeine (about fourfold greater than controls without sonophoresis) across human and hairless rat skin. In general, heparin, which is the most commonly used anticoagulant, is administered by intravenous or subcutaneous injections for the treatment of venous thromboembolism. Mitragotri et al. performed in vitro experiments with sonophoresis of 20 kHz with 7 W/cm2 (ISAPA) to deliver unfractionated heparin or low-molecular weight heparin (LMWH) across the skin (Mitragotri 2001). Biologic activity of transdermally delivered heparin was measured using activated clotting time assays and an antifactor Xa (aXa) chromogenic substrate assay. Transdermally delivered LMWH resulted in sustained aXa levels in the blood. This result was in strong contrast to subcutaneous or intravenous injections of LMWH, which resulted in only temporary elevations of aXa level. Mitragotri et al. proposed that patients might use a product based on this technology throughout the day to provide sustained levels of heparin concentration in the blood. The dose of heparin may be controlled through skin permeability, skin area, or LMWH concentration in the reservoir of the patch system used with sonophoresis. Ketoprofen is a nonsteroidal anti-inflammatory drug predominantly used in the treatment of rheumatoid arthritis and osteoarthritis (Cagnie et al. 2003). It is also used to relieve minor aches and menstrual pain (Maestrelli et al. 2006). Herwadkar et al. tested the effect of sonophoresis at 20 kHz with 6.9 W/cm2 for delivery of ketoprofen into and across the skin (Herwadkar et al. 2012). In vitro experiments were performed on excised hairless rat skin over a period of 24 h using Franz diffusion cells. Sonophoresis significantly enhanced the permeation of ketoprofen from 74.87 ± 5.27 μg/cm2 (for passive delivery) to 491.37 ± 48.78 μg/cm2. Further, drug levels in skin layers increased from 34.69 ± 7.25 μg following passive permeation to 212.62 ± 45.69 μg following sonophoresis. These results show that sonophoresis of 20 kHz is an effective active enhancement technique enhancing transdermal and topical delivery of ketoprofen.
Among the therapeutic drugs used in TDD, noninvasive transdermal delivery of insulin has received great attention due to the increasing incidence of diabetes, which is one of the most costly diseases that occur in all populations and age groups (Olefsky 2001). Management of diabetes often requires painful repetitive insulin subcutaneous injections up to three or four times each day (Park et al. 2007). Noninvasive insulin delivery through the skin would be therefore advantageous. Numerous studies of insulin delivery through the skin have been performed by many researchers (Tachibana 1992; Mitragotri et al. 1995b; Park et al. 2007; Smith et al. 2003; Lee et al. 2005; Al-Bataineh et al. 2012). The feasibility of using sonophoresis in insulin delivery in vitro across human skin has been evaluated by Smith et al. (Smith et al. 2003). They carried out experiments with two types of lightweight cymbal transducer arrays, i.e., a stack array with an intensity (ISPTP) of 15.4 ± 0.6 mW/cm2 and a standard array with an intensity (ISPTP) of 173.7 ± 1.2 mW/cm2 at 20 kHz, to improve the transport of insulin. Compared with passive transport method (in passive transmission, the skin surface was placed facing toward the compartment which was filled with a 50 U/mL insulin diluted with saline without ultrasound exposure over an exposure period of 1 h) (4.1 ± 0.5U), the stack and standard ultrasound array facilitated a greater than fourfold (20.3 ± 9.3U) and sevenfold (45.9 ± 12.9U) increase, respectively, in the noninvasive transdermal transport of Humulin® R insulin (Eli Lilly and Co., Indianapolis, IN). Compared to the control without sonophoresis, the standard array provided a fourfold increase in the sonophoresis-facilitated transdermal delivery of insulin. Regarding combined use of sonophoresis and iontophoresis, ultrasound reduces the duration and intensity of iontophoresis required to anesthetize the skin when using lidocaine hydrochloride. Ultrasound treatment of the skin followed by iontophoresis was more effective than only iontophoresis with regard to intensity of pain relief. Injection of a lidocaine hydrochloride can cause pain and tissue damage. Also its application in children is not recommended. The enhancement of transdermal permeation of lidocaine hydrochloride using ultrasound and iontophoresis is effective and safe in surface anesthesia.
6.1 Noninvasive Transdermal Monitoring
Another interesting application of sonophoresis in clinic is for noninvasive transdermal monitoring. Sonophoresis can enhance the skin permeability so that sufficient quantities of analyte, such as glucose, can be obtained for detection (Oliver et al. 2008; Ferrante doAmaral and Wolf 2008). Because traditional glucose meters for measurement of glucose level require frequent painful finger punctures to obtain samples several times a day, the development of rapid, convenient, noninvasive, painless methods for measuring glucose level was required. Indeed, Mitragotri et al. reported a potential method for noninvasive diagnostics based on ultrasonic skin permeabilization and subsequent extraction of interstitial fluid (ISF) across the skin, in which serum and ISF concentrations of various analytes can be measured (Mitragotri et al. 2000b). Glucose concentration in the extracted fluid correlated well with blood concentration of glucose over a rage of 50–250 mg/dl. The results demonstrated that sonophoresis can enhance skin permeability to obtain sufficient quantities of the analyte for the detection of the concentration of target molecules by application of vacuum for a short period.
6.2 Sonophoresis in Transcutaneous Immunization and Gene Therapy
Currently, the delivery of vaccines mostly relays on the needle-based methods. The biggest concern in needle vaccination is the potential reuse of needles and syringes causing a large number of HIV and hepatitis B virus cases (Kane et al. 1999), as well as the pain associated with the use of syringes. As an alternative, sonophoresis was proposed and has been researched recently. Tezel et al. used low-frequency sonophoresis at 20 kHz as a pretreatment prior to the application of tetanus toxin (TT) with sodium lauryl sulfate (SLS) added phosphate-buffered saline (Tezel et al. 2005). Approximately 10 μg of TT was permeated through the epidermis and induced the activation of Langerhans cells (LCs), which are highly potent immune cells replete within the epidermis (Babiuk et al. 2000). More recently, Dahlan et al. have reported a successful immunization by sonophoresis even without the aid of a chemical penetration enhancer (Dahlan et al. 2009b).
Another possible application for sonophoresis is in topical gene therapy. Gene therapy is a therapeutic method for correcting defective genes that are responsible for a certain disease, by replacing an abnormal disease-causing gene with a normal gene. For the purpose of targeting and protecting the genes, carrier molecules are used, and they are called vectors. Accordingly, gene vectors are relatively large-sized macromolecules, and hence topical delivery becomes difficult. Tezel et al. have shown the possibility of deoxyribonucleic acid (DNA) delivery through porcine skin in vitro (Tezel et al. 2004). Ultrasound sonication of 2.4 W/cm2 at 20 kHz frequency for 10 min induced 4.5 × 10−5 cm/h permeability compared to nearly undetectable values when using nontreated skin. However, topical delivery of gene using sonophoresis is still in its early stage for giving conclusions, and hence further studies are required.
6.3 Safety Issues and Commercial Devices
Ultrasound is generally accepted as a safe clinical method in both diagnostics and therapeutics as far as the system output is within IEC 60601 standard limit (IEC Standard 2009). Results from researches related to the bioeffect of sonophoresis to tissue also indicate similar results. Early reports of Singer et al. warned the possibility of skin burns at high intensity; however, IEC standard for safety regarding the intensity of therapeutic sonophoresis seems to be too high (Singer et al. 1998). In a more recent study investigating the bioeffect of sonophoresis, Marunai et al. applied ultrasound in the range from 1.57 to 3.5 W/cm2 intensity, at 35 kHz on the human skin (Maruani et al. 2012). The results showed that minor erythema could be occasionally observed, which disappeared within 24 h. Accordingly, sonophoresis is approved for human use by US Food and Drug Administration (FDA) SonoPrep® (Echo Therapeutics, Franklin, MA, USA) and is currently approved by US FDA as a sonophoresis specialized device for TDD (Polat et al. 2011b). However, the marketing of this device seems to be discontinued due to unknown reasons (Kalluri and Banga 2011).
Conclusion
In conclusion, sonophoresis, especially low-frequency (20–100 kHz) ultrasound, has been used in a wide range of therapeutic and industrial applications. Sonophoresis represents a safe and effective method for TDD; however, there exists gaps between the medical and industrial standards for ultrasound exposure. Therefore, optimized studies would be needed in the future to determine the safety standards for ultrasound exposure to tissues for various purposes.
References
Al-Bataineh OM, Lweesy K, Fraiwan L (2011) In-vivo evaluation of a noninvasive transdermal insulin delivery system utilizing ultrasound transducers. J Med Imag Health Inform 1:267–270
Al-Bataineh OM, Lweesy K, Fraiwan L (2012) Noninvasive transdermal insulin delivery using piston-shaped PZT transducers: in vivo rabbits evaluation. Jordan J Mech Indust Eng 6:11–16
Alvarez-Román R, Merino G, Kalia YN, Naik A, Guy RH (2003) Skin permeability enhancement by low frequency sonophoresis: lipid extraction and transport pathways. J Pharm Sci 92(6):1138–1146
Babiuk S, Baca-Estrada M, Babiuk LA, Ewen C, Foldvari M (2000) Cutaneous vaccination: the skin as an immunologically active tissue and the challenge of antigen delivery. J Control Rel 66(2/3):199–214
Barnett SB, ter Haar GR, Ziskin MC, Nyborg WL, Maeda K, Bang J (1994) Current status of research on biophysical effects of ultrasound. Ultrasound Med Biol 20:205–218
Benson HAE, McElnay JC, Harland R (1988) Phonophoresis of lignocaine and prilocaine from Emla cream. Int J Pharm 44:65–69
Benson HA, McElnay JC, Harland R, Hadgraft J (1991) Influence of ultrasound on the percutaneous absorption of nicotinate esters. Pharm Res 8(2):204–209
Bommannan D, Okuyama H, Stauffer P, Guy RH (1992a) Sonophoresis. I. The use of high-frequency ultrasound to enhance transdermal drug delivery. Pharm Res 9(4):559–564
Bommannan D, Menon GK, Okuyama H, Elias PM, Guy RH (1992b) Sonophoresis. II. Examination of the mechanism(s) of ultrasound-enhanced transdermal drug delivery. Pharm Res 9(8):1043–1047
Boucaud A, Machet L, Arbeille B, Machet MC, Sournac M, Mavon A, Patat F, Vaillant L (2001) In vitro study of low-frequency ultrasound-enhanced transdermal transport of fentanyl and caffeine across human and hairless rat skin. Int J Pharm 228:69–77
Cagnie B, Vinck E, Rimbaut S, Vanderstraeten G (2003) Phonophoresis versus topical application of ketoprofen: comparison between tissue and plasma levels. Phys Ther 83(8):707–712
Carvell KJ, Bigelow TA (2011) Dependence of optimal seed bubble size on pressure amplitude at therapeutic pressure levels. Ultrasonics 51:115–122
Cevc G, Schatzlein A, Blume G (1995) Transdermal drug carriers: basic properties, optimization and transfer efficiency in the case of epicutaneously applied peptides. J Control Release 36:3–16
Collis J, Manasseh R, Liovic P, Tho P, Ooi A, Petkovic-Duran K, Zhu Y (2010) Cavitation microstreaming and stress fields created by microbubbles. Ultrasonics 50:273–279
Colussi AJ, Weavers LK, Hoffmann MR (1998) Chemical bubble dynamics and quantitative sonochemistry. J Phys Chem 102:6927–6934
Crum LA (1984) Rectified diffusion. Ultrasonics 22:215–223
Dahlan A, Alpar HO, Murdan S (2009a) An investigation into the combination of low frequency ultrasound and liposomes on skin permeability. Int J Pharm 379:139–142
Dahlan A, Alpar HO, Stickings P, Sesardic D, Murdan S (2009b) Transcutaneous immunisation assisted by low-frequency ultrasound. Int J Pharm 368:123–128
Darrow H, Schulthies S, Draper D, Ricard M, Measom GJ (1999) Serum dexamethasone levels after Decadron phonophoresis. J Athl Train 34:338–341
Dias M, Farinha A, Faustino E, Hadgraft J, Pais J, Toscano C (1999) Topical delivery of caffeine from some commercial formulations. Int J Pharm 182:41–47
Edwards DA, Langer R (1994) A linear theory of transdermal transport phenomena. J Pharm Sci 83(9):1315–1334
Fellinger K, Schmid J, Klinik AN (1954) Therapie des Chronischen (transl.Clinical experience/practice about the therapy of the chronic (illness).Gelenkreumatismus (transl. Articular Rheumatism): 549–552
Ferrante doAmaral CE, Wolf B (2008) Current development in non-invasive glucose monitoring. Med Eng Phys 30:541–549
Fiorillo AS, Grimaldi D, Paolino D, Pullano SA (2012) Low-frequency ultrasound in medicine: an in vivo evaluation. IEEE Trans Instrument Measure 61(6):1658–1663
Fry WJ (1954) Intense ultrasound: a new tool for neurological research. J Ment Sci 100:85–96
Fry WJ, Mosberg W, Barnard JW (1954) Production of focal destructive lesions in the central nervous system with ultrasound. J Neurosurg 11:471–478
Fry FJ, Ades HW, Fry WJ (1958) Production of reversible changes in the central nervous system by ultrasound. Science 127:83–84
Griffin JE (1966) Physiological effects of ultrasonic energy as it is used clinically. J Am Phys Ther Assoc 46:18–26
Griffin JE, Touchstone JC (1968) Low-intensity phonophoresis of cortisol in swine. Phys Ther 48(12):1336–1344
Griffin JE, Echternach JL, Price RE, Touchstone JC (1967) Patients treated with ultrasonic driven hydrocortisone and with ultrasound alone. Phys Ther 47(7):594–601
Gupta J, Prausnitz MR (2009) Recovery of skin barrier properties after sonication in human subjects. Ultrasound Med Biol 35(8):1405–1408
Harvey EN, Barnes DK, McElroy WD, Whiteley AH, Pease DC, Cooper KW (1944) Bubble formation in animals. I. Physical factors. J Cell Comp Physiol 24(1):1–22
Herwadkar A, Sachdeva V, Taylor LF, Silver H, Banga AK (2012) Low frequency sonophoresis mediated transdermal and intradermal delivery of ketoprofen. Int J Pharm 423(2):289–296
International Electrotechnical Commission (IEC) Ed 3.0. EN 60601-2-5. Medical electrical equipment. Particular requirements for the basic safety and essential performance of ultrasonic physiotherapy equipment. IEC, Geneva, Switzerland (2009)
Johnson ME, Mitragotri S, Patel A, Blankschtein D, Langer R (1996) Synergistic effect of ultrasound and chemical enhancers on transdermal drug delivery. J Pharm Sci 85:670–679
Kalluri H, Banga AK (2011) Transdermal delivery of proteins. AAPS PharmSciTech 12(1):431–441
Kane A, Lloyd J, Zaffran M, Simonsen L, Kane M (1999) Transmission of hepatitis B, hepatitis C and human immunodeficiency viruses through unsafe injections in the developing world: model-based regional estimates. Bull World Health Organ 77(10):801–807
Katz JI (1995) Jets from collapsing bubbles. Proc R Soc Lond A 455:323–328
Kim do K, Choi SW, Kwak YH (2012) The effect of SonoPrep® on EMLA® cream application for pain relief prior to intravenous cannulation. Eur J Pediatr 171(6):985–988
Kost J, Pliquett U, Mitragotri S, Yamamoto A, Langer R, Weaver J (1996) Synergistic effect of electric field and ultrasound on transdermal transport. Pharm Res 13(4):633–638
Kushner J 4th, Blankschtein D, Langer R (2008a) Heterogeneity in skin treated with low-frequency ultrasound. J Pharm Sci 97(10):4119–4128
Kushner J 4th, Blankschtein D, Langer R (2008b) Evaluation of hydrophilic permeant transport parameters in the localized and non-localized transport regions of skin treated simultaneously with low-frequency ultrasound and sodium lauryl sulfate. J Pharm Sci 97:894–906
Lavon I, Kost J (2004) Ultrasound and transdermal drug delivery. Drug Discov Today 9(15):670–676
Lavon I, Grossman N, Kost J, Kimmel E, Enden G (2007) Bubble growth within the skin by rectified diffusion might play a significant role in sonophoresis. J Control Release 117(2):246–255
Le L, Kost J, Mitragotri S (2000) Combined effect of low-frequency ultrasound and iontophoresis: applications for transdermal heparin delivery. Pharm Res 17(9):1151–1154
Lee S, McAuliffe DJ, Flotte TJ, Kollias N, Doukas AG (1998) Photomechanical transcutaneous delivery of macromolecules. J Invest Dermatol 111:925–929
Lee S, Snyder B, Newnham RE, Smith NB (2005) Noninvasive ultrasonic transdermal insulin delivery in rabbits using the light-weight cymbal array. Diabetes Technol Ther 6:808–815
Lee S, Choi K, Menon GK, Kim H, Choi E, Ahn S, Lee S (2010) Penetration pathways induced by low-frequency sonophoresis with physical and chemical enhancers: iron oxide nanoparticles versus lanthanum nitrates. J Invest Dermatol 130:1063–1072
Leighton TG (1997) The acoustic bubble. Academic, London
Lokhandwalla M, Sturtevant B (2000) Fracture mechanics model of stone comminution in ESWL and implications for tissue damage. Phys Med Biol 45:1923–1940
Maestrelli F, González-Rodríguez ML, Rabasco AM, Mura P (2006) Effect of preparation technique on the properties of liposomes encapsulating ketoprofen–cyclodextrin complexes aimed for transdermal delivery. Int J Pharm 312:53–60
Maione E, Shung KK, Meyer RJ Jr, Hughes JW, Newnham RE, Smith NB (2002) Transducer design for a portable ultrasound enhanced transdermal drug-delivery system. IEEE Trans Ultrason Ferroelectr Freq Control 49(10):1430–1436
Maruani A, Vierron E, Machet L, Giraudeau B, Boucaud A (2010) Efficiency of low-frequency ultrasound sonophoresis in skin penetration of histamine: a randomized study in humans. Int J Pharm 385:37–41
Maruani A, Vierron E, Machet L, Giraudeau B, Halimi JM, Boucaud A (2012) Tolerance of low-frequency ultrasound sonophoresis: a double-blind randomized study on humans. Skin Res Technol 18(2):151–156
McElnay JC, Matthews MP, Harland R, McCafferty DF (1985) The effect of ultrasound on the percutaneous absorption of lignocaine. Br J Clin Pharmacol 20(4):421–424
McElnay JC, Kennedy TA, Harland R (1987) The influence of ultrasound on the percutaneous absorption of fluocinolone acetonide. Int J Pharm 40:105–110
Meidan VM, Docker MF, Walmsley AD, Irwin WJ (1998) Phonophoresis of hydrocortisone with enhancers: an acoustically defined model. Int J Pharm 170:157–168
Meltzer RS (1996) Food and Drug Administration ultrasound device regulation: the output display standard, the “mechanical index”, and ultrasound safety. J Am Soc Echocardiogr 9:216–220
Merino G, Kalia YN, Delgado-Charro MB, Potts RO, Guy RH (2003) Frequency and thermal effects on the enhancement of transdermal transport by sonophoresis. J Control Release 88:85–94
Merritt CR, Kremkau FW, Hobbins JC (1992) Diagnostic ultrasound: bioeffects and safety. Ultrasound Obstet Gynecol 2:366–374
Mitragotri S (2000) Synergistic effect of enhancers for transdermal drug delivery. Pharm Res 17(11):1354–1359
Mitragotri S (2001) Effect of therapeutic ultrasound on partition and diffusion coefficients in human stratum corneum. J Control Release 71(1):23–29
Mitragotri S, Kost J (2000) Low frequency sonophoresis: a noninvasive method of drug delivery and diagnostics. Biotech Prog 16:488–492
Mitragotri S, Kost J (2001) Transdermal delivery of heparin and low-molecular weight heparin using low-frequency ultrasound. Pharm Res 18:1151–1156
Mitragotri S, Kost J (2004) Low-frequency sonophoresis: a review. Adv Drug Deliv Rev 56(5):589–601
Mitragotri S, Edwards DA, Blankschtein D, Langer R (1995a) A mechanistic study of ultrasonically-enhanced transdermal drug delivery. J Pharm Sci 84(6):697–706
Mitragotri S, Blankschtein D, Langer R (1995b) Ultrasound-mediated transdermal protein delivery. Science 269(5225):850–853
Mitragotri S, Blankschtein D, Langer R (1996) Transdermal drug delivery using low-frequency sonophoresis. Pharm Res 13(3):411–420
Mitragotri S, Ray D, Farrell J, Tang H, Yu B, Kost J, Blankschtein D, Langer R (2000a) Synergistic effect of ultrasound and sodium lauryl sulfate on transdermal drug delivery. J Pharm Sci 89:892–900
Mitragotri S, Coleman M, Kost J, Langer R (2000b) Transdermal extraction of analytes using low-frequency ultrasound. Pharm Res 17(4):466–470
Mitragotri S, Farrell J, Tang H, Terahara T, Kost J, Langer R (2000c) Determination of threshold energy dose for ultrasound-induced transdermal drug transport. J Control Release 63(1-2):41–52
Mutalik S, Nayak UY, Kalra R, Kumar A, Kulkarni RV, Parekh HS (2012) Sonophoresis-mediated permeation and retention of peptide dendrimers across human epidermis. Skin Res Technol 18:101–107
Ngamratanapaiboon S, Iemsan-Arng J, Yambangyang P, Neatpisarnvanit C, Sirisoonthorn S, Sathirakul K (2012) In vitro study the transdermal permeation profiles of L-ascorbic acid in chitosan hydrogel formulation altered by sonophoresis. Adv J Pharma Sci 1:13–17
Nightingale KR, Kornguth PJ, Trahey GE (1999) The use of acoustic streaming in breast lesion diagnosis: a clinical study. Ultrasound Med Biol 25:75–87
Nyborg WL (2001) Biological effects of ultrasound development of safety guidelines. Path II general reviews. Ultrasound Med Biol 27:301–333
Olefsky JM (2001) Prospects for research in diabetes mellitus. JAMA 285:628–632
Oliver N, Toumazou CT, Cass A, Johnston D (2008) Glucose sensors: a review of current and emerging technology. Diabet Med 26:197–210
Paix A, Coleman A, Lees J, Grigson J, Brooksbank M, Thorne D, Ashby M (1995) Subcutaneous fentanyl and sufentanil infusion substitution for morphine intolerance in cancer pain management. Pain 63(2):263–269
Paliwal S, Menon GK, Mitragotri S (2006) Low-frequency sonophoresis: ultrastructural basis for stratum corneum permeability assessed using quantum dots. J Invest Dermatol 126(5):1095–1101
Park R, Jang K, Park S, Cho H, Jin C, Choi M, Chung S, Min B (2005) The effect of sonication on simulated osteoarthritis. Part I: effects of 1 MHz ultrasound on uptake of hyaluronan into the rabbit synovium. Ultrasound Med Biol 31:1551–1558
Park EJ, Werner J, Smith NB (2007) Ultrasound mediated transdermal insulin delivery in pigs using a lightweight transducer. Pharm Res 24(7):1396–1401
Park D, Yoon J, Park J, Jung B, Park H, Seo J (2010) Transdermal drug delivery aided by an ultrasound contrast agent: an in vitro experimental study. Open Biomed Eng J 4:56–62
Park D, Ryu H, Kim H, Kim Y, Choi K, Park H, Seo J (2012) Sonophoresis using ultrasound contrast agents for transdermal drug delivery: an in vivo experimental study. Ultrasound Med Biol 38:642–650
Pecha R, Gompf B (2000) Microimplosions: cavitation collapse and shock wave emission on a nanosecond time scale. Phys Rev Lett 84:1328–1330
Pires-de-Campos MSM, Polacow MLO, Granzotto TM, Spadari-Bratfisch RC, Leonardi GR, Grassi-Kassisse DM (2007) Influence of the ultrasound in cutaneous permeation of the caffeine: in vitro study. Pharmacol Online 1:477–486
Polat BE, Seto JE, Blankschtein D, Langer R (2011a) Application of the aqueous porous pathway model to quantify the effect of sodium lauryl sulfate on ultrasound-induced skin structural perturbation. J Pharm Sci 100:1387–1397
Polat BE, Figueroa PL, Blankschtein D, Langer R (2011b) Transport pathways and enhancement mechanisms within localized and non-localized transport regions in skin treated with low-frequency sonophoresis and sodium lauryl sulfate. J Pharm Sci 100(2):512–529
Popinet S, Zaleski S (2002) Bubble collapse near a solid boundary: a numerical study of the influence of viscosity. J Fluid Mech 464:137–163
Rosim GC, Barbieri CH, Lancas FM, Mazzer N (2005) Diclofenac phonophoresis in human volunteers. Ultrasound Med Biol 31:337–343
Sarheed O, Abdul Rasool BK (2011) Development of an optimized application protocol for sonophoretic transdermal delivery of a model hydrophilic drug. Open Biomed Eng J 5:14–24
Sarheed O, Frum Y (2012) Use of the skin sandwich technique to probe the role of the hair follicles in sonophoresis. Int J Pharm 423:179–183
Scheuplein RJ (1967) Mechanism of percutaneous absorption. II. Transient diffusion and the relative importance of various routes of skin penetration. J Invest Dermatol 1967(48):79–88
Scheuplein RJ, Blank IH, Brauner GJ, MacFarlane DJ (1969) Percutaneous absorption of steroids. J Invest Dermatol 52:63–70
Schroeder A, Kost J, Barenholz Y (2009) Ultrasound, liposomes, and drug delivery: principles for using ultrasound to control the release of drugs from liposomes. Chem Phys Lipids 162:1–16
Singer AJ, Homan CS, Church AL, McClain SA (1998) Low-frequency sonophoresis: pathologic and thermal effects in dogs. Acad Emerg Med 5(1):35–40
Smith NB (2007) Perspectives on transdermal ultrasound mediated drug delivery. Int J Nanomedicine 2(4):585–594
Smith NB, Lee S, Maione E, Roy RB, Mcelligott S, Shung KK (2003) Ultrasound-mediated transdermal transport of insulin in vitro through human skin using novel transducer designs. Ultrasound Med Biol 29:311–317
Suslick KS (1989) The chemical effects of ultrasound. Sci Am 260:80–86
Szabo TL (2004) Diagnostic ultrasound imaging: inside out. Elsevier, Amsterdam/Boston
Tachibana K (1992) Transdermal delivery of insulin to alloxan-diabetic rabbits by ultrasound exposure. Pharm Res 9(7):952–954
Tachibana K, Tachibana S (1991) Transdermal delivery of insulin by ultrasonic vibration. J Pharm Pharmacol 43(4):270–271
Tachibana K, Tachibana S (1993) Use of ultrasound to enhance the local anesthetic effect of topically applied aqueous lidocaine. Anesthesiology 78(6):1091–1096
Tang H, Wang CC, Blankschtein D, Langer R (2002) An investigation of the role of cavitation in low-frequency ultrasound-mediated transdermal drug transport. Pharm Res 19(8):1160–1169
Terahara T, Mitragotri S, Kost J, Langer R (2002) Dependence of low-frequency sonophoresis on ultrasound parameters; distance of the horn and intensity. Int J Pharm 235(1-2):35–42
Tezel A, Sens A, Tuchscherer J, Mitragotri S (2001) Frequency dependence of sonophoresis. Pharm Res 18(12):1694–1700
Tezel A, Sens A, Tuchscherer J, Mitragotri S (2002a) Synergistic effect of low-frequency ultrasound and surfactants on skin permeability. J Pharm Sci 91(1):91–100
Tezel A, Sens A, Mitragotri S (2002b) A theoretical analysis of low-frequency sonophoresis: dependence of transdermal transport pathways on frequency and energy density. Pharm Res 19(12):1841–1846
Tezel A, Sens A, Mitragotri S (2003) Description of transdermal transport of hydrophilic solutes during low-frequency sonophoresis based on a modified porous pathway model. J Pharm Sci 92(2):381–393
Tezel A, Dokka S, Kelly S, Hardee GE, Mitragotri S (2004) Topical delivery of anti-sense nucleotides using low-frequency sonophoresis. Pharm Res 21:2219–2225
Tezel A, Paliwal S, Shen Z, Mitragotri S (2005) Low-frequency ultrasound as a transcutaneous immunization adjuvant. Vaccine 23(29):3800–3807
Tiwari SB, Pai RM, Udupa N (2000) Influence of ultrasound on the percutaneous absorption of ketorolac tromethamine in vitro across rat skin. Drug Deliv 11:447–451
Ueda H, Mutoh M, Seki T, Kobayashi D, Morimoto Y (2009) Acoustic cavitation as an enhancing mechanism of low-frequency sonophoresis for transdermal drug delivery. Biol Pharm Bull 32(5):916–920
Wang Y, Thakur R, Fan Q, Michniak B (2005) Transdermal iontophoresis: combination strategies to improve transdermal iontophoretic drug delivery. Eur J Pharm Biopharm 60:179–191
Wolloch L, Kost J (2010) The importance of microjet vs shock wave formation in sonophoresis. J Control Release 148(2):204–211
Yang J, Kim D, Yun M, Kim T, Shin S (2006) Transdermal delivery system of triamcinolone acetonide from a gel using phonophoresis. Arch Pharm Res 29:412–417
Author information
Authors and Affiliations
Corresponding author
Editor information
Editors and Affiliations
Rights and permissions
Copyright information
© 2017 Springer-Verlag Berlin Heidelberg
About this chapter
Cite this chapter
Lee, S.E., Seo, J., Lee, S.H. (2017). The Mechanism of Sonophoresis and the Penetration Pathways. In: Dragicevic, N., I. Maibach, H. (eds) Percutaneous Penetration Enhancers Physical Methods in Penetration Enhancement. Springer, Berlin, Heidelberg. https://doi.org/10.1007/978-3-662-53273-7_2
Download citation
DOI: https://doi.org/10.1007/978-3-662-53273-7_2
Published:
Publisher Name: Springer, Berlin, Heidelberg
Print ISBN: 978-3-662-53271-3
Online ISBN: 978-3-662-53273-7
eBook Packages: MedicineMedicine (R0)