Abstract
For p-(dimethylamino)chalcone (p-DMAC), the N atom is the most basic site in the liquid phase, whereas the O atom possesses the highest proton affinity in the gas phase. A novel and interesting observation is reported that the N- and O-protonated p-DMAC can be competitively produced in atmospheric pressure chemical ionization (APCI) with the change of solvents and ionization conditions. In neat methanol or acetonitrile, the protonation is always under thermodynamic control to form the O-protonated ion. When methanol/water or acetonitrile/water was used as the solvent, the protonation is kinetically controlled to form the N-protonated ion under conditions of relatively high infusion rate and high concentration of water in the mixed solvent. The regioselectivity of protonation of p-DMAC in APCI is probably attributed to the bulky solvent cluster reagent ions (SnH+) and the analyte having different preferred protonation sites in the liquid phase and gas phase.

Figure
ᅟ
Similar content being viewed by others
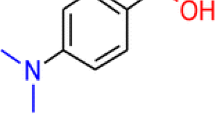
Avoid common mistakes on your manuscript.
1 Introduction
Electrospray ionization (ESI) and atmospheric pressure chemical ionization (APCI) are important and popular ionization techniques. Based on these methods, mass spectrometry has been a powerful analytical tool in many areas of science and applications. Despite widespread use of these two methods, their fundamental aspects on the ionization process have not yet been fully understood, which is crucial for further applications and developments. In these two soft ionization methods, protonation (or deprotonation) usually takes place predominantly on the most basic (or acidic) site of a molecule to produce the thermodynamically stable ions. However, when the most basic (or acidic) site of a compound in the gas phase differs from that in solution, it is still unclear that the ionization should follow the liquid-phase or gas-phase thermodynamics. In ESI, the charged analyte is formed in the solution first and then transferred to the gas phase, but recently several researchers reported the regioselectivity of protonation (or deprotonation) of a given molecule through changing the spraying solvent [1–7]. For example, in the protonation of p-aminobenzoic acid, Kass and Tian observed that ESI produces gas-phase structure (O-protonation) in methanol/water while liquid-phase structure (N-protonation) in acetonitrile/water [1]. APCI is a good complement to ESI. It is best for low molecular weight (<1000 Da), thermally stable, and moderately to low polar samples [8–10]. In APCI, the analyte and solvent are converted to a gaseous state first and then the analyte is charged through gas-phase ion/molecule reactions with solvent reagent ions, thus it seems no doubt that the gas-phase thermodynamically favored ion should be formed. However, in this work, we newly found that the protonation site of p-(dimethylamino)chalcone (p-DMAC, structure see Table 1) upon APCI is changeable at different ionization conditions leading to produce either the gas-phase or liquid-phase thermodynamically favored protonated species.
2 Experimental
2.1 Materials
N,N-Dimethylaniline, p-dimethylaminobenzophenone, and p-aminobenzoic acid were all commercial products, and were used without further treatment. p-(Dimethylamino)chalcone was synthesized and purified following reported procedures [11]. The structure was confirmed by NMR spectroscopy and mass spectrometry. The purity was checked by HPLC.
2.2 Mass Spectrometry
The mass spectrometric experiments were performed on a Varian 500-MS ion trap mass spectrometer equipped with an APCI interface. Nitrogen was used as the nebulizing gas and the drying gas. A solution containing the analytes (1 μg mL–1) was infused to the mass spectrometer with a syringe pump at a flow rate of 5–50 μL min–1. Unless stated otherwise, APCI in positive ion mode was performed using the following settings: spray chamber temperature 65 °C, nebulizer gas pressure 35 psi, drying gas pressure 10 psi, drying gas temperature 350 °C, vaporizer gas pressure 20 psi, vaporizer gas temperature 350 °C, corona current 5 μA, spray shield voltage 600 V, capillary voltage 75 V, rf loading 75 %, scan mass range 50–600 m/z. The collision-induced dissociation (CID) mass spectra were obtained with helium as the collision gas after isolation of the desired precursor ion. The isolation window is 1 m/z, waveform type is resonant, and excitation amplitude is 0.7 V.
2.3 Theoretical Calculations
All theoretical calculations were carried out at the B3LYP/6-311++G(2d,p) level of theory using the Gaussian 03 suit of programs [13]. Frequency analysis was performed at the same theoretical level to confirm that the optimized species have no imaginary frequency. The proton affinities (PAs) were calculated using the semiclassical approximation: PA = −ΔE + 5/2RT, where ΔE = E(BH+) − E(B) [13]. The resulting energetics were reported as electronic and thermal energies at 298 K corrected for zero-point energy (ZPE).
3 Results and Discussion
APCI mass spectrometry was used in the fragmentation studies of protonated chalcone and its derivatives in our previous work [14, 15]. The most favorable gas-phase protonation site for the unsubstituted chalcone is the carbonyl oxygen [15]. The dimethylamino group of p-DMAC is a competitive protonation site with the carbonyl oxygen. The PA of N,N-dimethylaniline is higher than that of aromatic ketones (e.g., PA(C6H5N(CH3)2) = 941.1 kJ mol–1, PA(C6H5COC6H5) = 882.3 kJ mol–1, and PA(C6H5COCH3) = 861.1 kJ mol–1) [16], but this does not insure such priority is retained in a conjugated molecule (p-DMAC) consisting of these two parts. The p-dimethylamino group, as a strong electron-donating group, increases the PA of the carbonyl group, while the benzoyl vinyl group, as an electron-withdrawing group, decreases the PA of the p-dimethylamino group. Such conjugated effect makes the preferred protonation site in p-DMAC uncertain.
To predict the most favored protonation site in the gas phase, density functional theory (DFT) calculations at the B3LYP/6-311++G(2d,p) basis set were carried out. Protonation at the carbonyl oxygen forms the thermodynamically stable ion, which is 70.9 kJ mol–1 lower than the N-protonated ion in terms of energy (Table 1). Therefore, the carbonyl oxygen of p-DMAC is the preferred protonation site in the gas phase.
It is well-known that the amino group of an organic compound is usually the preferred protonation site in solution. The 13C NMR spectra of p-DMAC were recorded to clearly determine the most basic site of p-DMAC in solution and the spectra are presented in the supplementary material [17]. The N-methyl carbon resonance was found to shift downfield (Δδ = 6.6 ppm) in 3:1 (vol/vol) CD3CN/H2O-HCl(1 M) solution compared with in 3:1 (vol/vol) CD3CN/H2O solution. The change of carbonyl carbon resonance was quite small in contrast to the known significant shift of a carbonyl carbon upon its protonation [18]. The N-methyl carbon resonance of a reference compound, N,N-dimethylaniline, was also found to shift downfield (Δδ = 6.3 ppm) under identical acidified conditions. The changes in the ring carbon shifts for the protonated p-DMAC and N,N-dimethylaniline also mimic that for the N-protonated p-aminobenzoic acid [2, 19]. These results indicate that the nitrogen of p-DMAC is the preferred site for protonation in liquid phase.
Determination of the actual site of protonation in the gas phase can be achieved with the aid of tandem mass spectrometry (MS/MS). The CID spectra of the [M + H]+ ion of p-DMAC generated by APCI from 2:1 (vol/vol) CH3CN/H2O at infusion rates of 5 and 50 μL min–1 respectively exhibit clear difference in relative abundance (RA) of product ions, as shown in Figure 1. The most abundant product ion at a low infusion rate is m/z 105 but that at a high infusion rate is m/z 237, which indicates that the [M + H]+ ions have different structures. In dissociation reactions, the O- and N-protonated p-DMAC can show different fragmentation behavior (Scheme 1). Product ion m/z 237 is generated by loss of a methyl radical from the N-protonated p-DMAC, which is a common fragmentation reaction observed in other protonated N-methyl analogues [20–22]. Fragmentation of the O-protonated p-DMAC leads to the product ions at m/z 105, 174, and 234 [13, 14]. Multi-step proton transfers from O to N in CID may be considered [23, 24], but the huge difference in fragmentation behavior suggests this process probably does not occur in the fragmentation of protonated p-DMAC. It is noteworthy that the collision energy and sample concentration have little effect on the abundance ratio of m/z 237/105 (Supplementary Figures S1 and S2). Furthermore, the protonation of p-DMAC was also studied by ESI and CID fragmentations (Supplementary Figure S3). The formation of O-protonated ion (gas-phase structure) is preferred in 2:1 (vol/vol) CH3OH/H2O while the formation of N-protonated ion (liquid-phase structure) is preferred in 2:1 (vol/vol) CH3CN/H2O. This result is well consistent with that observed in the case of p-aminobenzoic acid [1].
The most commonly used solvents in the reversed-phase liquid chromatography-mass spectrometry, CH3CN/H2O and CH3OH/H2O in different proportions, were further investigated for protonation of p-DMAC upon APCI (Table 2). The O-protonated species is always dominant at an infusion rate of 5 μL min–1 irrespective of the solvent used in this study (Supplementary Figures S4 and S5). At an infusion rate of 50 μL min–1, in neat methanol or acetonitrile, the O-protonated species is still the predominant protonated ion, however, the presence of water and its higher concentration in the mixed solvents favor the formation of the N-protonated species. Using the solvent mixtures, when the flow rate is stepwise increased from 5 μL min–1 to 50 μL min–1, the formation of the N-protonated species is more and more favored (Supplementary Figures S4 and S5). These results indicate that the solvent and infusion rate are two important factors to control the protonation process upon APCI.
APCI is a form of chemical ionization (CI) that takes place at atmospheric pressure. Kinetic control of protonation means a thermodynamically less favored site is protonated preferentially. Kinetically controlled protonation under CI conditions is known because of steric hindrance, reagent ion, temperature, or other factors [25–31]. Recently, kinetic control of protonation in ESI was reported [1, 2, 32]. However, such phenomenon has not been investigated in APCI. The ion formation in APCI requires a series of successive gas-phase reactions initiated by the corona discharge [9, 10]. N2 +• and N4 +• are the primary reagent ions and S+• (S = solvent) is the secondary reagent ion. Then S+• undergoes repeated collisions with the analyte (M) resulting in the formation of the protonated analyte as described in Equations. 1, 2, 3, 4 below. It is well acknowledged that protonated solvent clusters (SnH+) in APCI play a very important role, even more important than the protonated single solvent molecule (SH+) [10]. By using different mixed solvents or different infusion rates,
the clustering degree (n) of SnH+ is different. Water is easier to form clusters than methanol and acetonitrile. Higher concentration of water in the mixed solvent or faster infusion rate leads more water to be sprayed into the source chamber, which results in the formation of bulky cluster reagent ions. When n is large, the intermediate solvated analyte ion (SnMH+) adopts liquid-phase structure (N-protonation) and it can be retained in the desolvation process. The kinetic control of protonation upon APCI is probably attributed to the bulky cluster reagent ions and the analyte having different preferred protonation sites in the liquid phase and gas phase. When n is small, the protonation upon APCI is a thermodynamically controlled process to form the O-protonated ion. In addition, the APCI interface parameters, including drying gas pressure, nebulizer gas pressure, vaporizer gas pressure, drying gas temperature, and vaporizer gas temperature, were also examined (Supplementary Figures S6–S9). Generally, the higher values of them disfavor the formation of bulky cluster reagent ions and so the thermodynamically controlled protonation is favored. If the solvent is CH3OH/H2O, the initial liquid-phase structure may be modified to the gas-phase structure by methanol clusters in the desolvation process (a proposed explanation also in ESI [1]), so only when the ratio of methanol in CH3OH/H2O is low enough (see Table 2 for detail), the N-protonated ion can be generated.
As additional evidence, the protonation of p-dimethylaminobenzophenone [(CH3)2NC6H4COC6H5] and p-aminobenzoic acid (H2NC6H4COOH) upon APCI was also investigated (Supplementary Figures S10 and S11). By using different solvents and infusion rates, the regioselectivity of protonation (O- versus N-protonation) is in accordance with that in the case of p-DMAC.
4 Conclusions
The kinetically or thermodynamically controlled protonation of p-DMAC in APCI can be observed with the change of solvents and ionization conditions. An interface is a bridge of liquid phase and gas phase, so the ionization process is influenced by both liquid- and gas-phase chemistry. Generally, the protonation upon APCI should be under the control of gas-phase thermodynamics. However, the liquid-phase thermodynamics can also largely affect the protonation process because the intermediate gas-phase microsolvated analyte ions have certain properties of ions in solution. This study improves our knowledge on the mechanism of APCI and the ion chemistry at the boundary of liquid phase and gas phase. It also reminds us of the importance of condition control in qualitative and quantitative analysis using APCI mass spectrometry.
References
Tian, Z., Kass, S.R.: Gas-phase versus liquid-phase structures by electrospray ionization mass spectrometry. Angew. Chem. Int. Ed. 48, 1321–1323 (2009)
Schmidt, J., Meyer, M.M., Spector, I., Kass, S.R.: Infrared multiphoton dissociation spectroscopy study of protonated p-aminobenzoic acid: does electrospray ionization afford the amino- or carboxy-protonated ion? J. Phys. Chem. A 115, 7625–7632 (2011)
Tian, Z., Pawlow, A., Poutsma, J.C., Kass, S.R.: Are carboxyl groups the most acidic sites in amino acids? Gas-phase acidity, H/D exchange experiments, and computations on cysteine and its conjugate base. J. Am. Chem. Soc. 129, 5403–5407 (2007)
Tian, Z., Kass, S.R.: Does electrospray ionization produce gas-phase or liquid-phase structures? J. Am. Chem. Soc. 130, 10842–10843 (2008)
Tian, Z., Wang, X.-B., Wang, L.-S., Kass, S.R.: Are carboxyl groups the most acidic sites in amino acids? Gas-phase acidities, photoelectron spectra, and computations on tyrosine, p-hydroxybenzoic acid, and their conjugate bases. J. Am. Chem. Soc. 131, 1174–1181 (2009)
Steill, J.D., Oomens, J.: Gas-phase deprotonation of p-hydroxybenzoic acid investigated by IR spectroscopy: solution-phase structure is retained upon ESI. J. Am. Chem. Soc. 131, 13570–13571 (2009)
Schröder, D., Buděšínský, M., Roithová, J.: Deprotonation of p-hydroxybenzoic acid: does electrospray ionization sample solution or gas-phase structures? J. Am. Chem. Soc. 134, 15897–15905 (2012)
Sunner, J., Nicol, G., Kebarle, P.: Factors determining relative sensitivity of analytes in positive mode atmospheric pressure ionization mass spectrometry. Anal. Chem. 60, 1300–1307 (1988)
Andrade, F.J., Shelley, J.T., Wetzel, W.C., Webb, M.R., Gamez, G., Ray, S.J., Hieftje, G.M.: Atmospheric pressure chemical ionization source. 1. Ionization of compounds in the gas phase. Anal. Chem. 80, 2646–2653 (2008)
Terrier, P., Desmazières, B., Tortajada, J., Buchmann, W.: APCI/APPI for synthetic polymer analysis. Mass Spectrom. Rev. 30, 854–874 (2011)
Moulton, B.E., Duhme-Klair, A.K., Fairlamb, I.J.S., Lynam, J.M., Whitwood, A.C.: A rationale for the linear correlation of aryl substituent effects in iron(0) tricarbonyl complexes containing α, β-unsaturated enone (chalcone) ligands. Organometallics 26, 6354–6365 (2007)
Frisch, M.J., Trucks, G.W., Schlegel, H.B., Scuseria, G.E., Robb, M.A., Cheeseman, J.R., Montgomery Jr., J.A., Vreven, T., Kudin, K.N., Burant, J.C., Millam, J.M., Iyengar, S.S., Tomasi, J., Barone, V., Mennucci, B., Cossi, M., Scalmani, G., Rega, N., Petersson, G.A., Nakatsuji, H., Hada, M., Ehara, M., Toyota, K., Fukuda, R., Hasegawa, J., Ishida, M., Nakajima, T., Honda, Y., Kitao, O., Nakai, H., Klene, M., Li, X., Knox, J.E., Hratchian, H.P., Cross, J.B., Adamo, C., Jaramillo, J., Gomperts, R., Stratmann, R.E., Yazyev, O., Austin, A.J., Cammi, R., Pomelli, C., Ochterski, J.W., Ayala, P.Y., Morokuma, K., Voth, G.A., Salvador, P., Dannenberg, J.J., Zakrzewski, V.G., Dapprich, S., Daniels, A.D., Strain, M.C., Farkas, O., Malick, D.K., Rabuck, A.D., Raghavachari, K., Foresman, J.B., Ortiz, J.V., Cui, Q., Baboul, A.G., Clifford, S., Cioslowski, J., Stefanov, B.B., Liu, G., Liashenko, A., Piskorz, P., Komaromi, I., Martin, R.L., Fox, D.J., Keith, T., Al-Laham, M.A., Peng, C.Y., Nanayakkara, A., Challacombe, M., Gill, P.M.W., Johnson, B., Chen, W., Wong, M.W., Gonzalez, C., Pople, J.A.: Gaussian 03, Revision B, Gaussian, Inc., Pittsburgh, PA, 2003
Bortolini, O., Fantin, G., Ferretti, V., Fogagnolo, M., Giovannini, P.P., Medici, A.: Relative acidity scale of bile acids through ESI-MS measurements. Org. Biomol. Chem. 8, 3674–3677 (2010)
Tai, Y., Pei, S., Wan, J., Cao, X., Pan, Y.: Fragmentation study of protonated chalcones by atmospheric pressure chemical ionization and tandem mass spectrometry. Rapid Commun. Mass Spectrom. 20, 994–1000 (2006)
Hu, N., Tu, Y.-P., Liu, Y., Jiang, K., Pan, Y.: Dissociative protonation and proton transfers: fragmentation of α, β-unsaturated aromatic ketones in mass spectrometry. J. Org. Chem. 73, 3369–3376 (2008)
Hunter, E.P.L., Lias, S.G.: Evaluated gas phase basicities and proton affinities of molecules: an update. J. Phys. Chem. Ref. Data 27, 413–656 (1998)
Zhang, X.X., Oscarson, J.L., Izatt, R.M., Schuck, P.C., Li, D.: Thermodynamics of macroscopic and microscopic proton ionization from protonated 4-aminobenzoic acid in aqueous solution from 298.15 to 393.15 K. J. Phys. Chem. B 104, 8598–8605 (2000)
Olah, G.A., White, A.M.: Stable carbonium ions. LVIII. Carbon-13 resonance investigation of protonated carboxylic acids (carboxonium ions) and oxocarbonium ions (acyl cations). J. Am. Chem. Soc. 89, 7072–7075 (1967)
Laufer, D.A., Gelb, R.I., Schwartz, L.M.: 13C NMR determination of acid–base tautomerization equilibria. J. Org. Chem. 49, 691–696 (1984)
Schröder, D., Roithová, J., Gruene, P., Schwarz, H., Mayr, H., Koszinowski, K.: Unexpected gas-phase reactivity of the CH3OH adduct of Michler’s hydrol blue: proton-shuttle catalysis and stepwise radical expulsions. J. Phys. Chem. A 111, 8925–8933 (2007)
Levsen, K., Schiebel, H.-M., Terlouw, J.K., Jobst, K.J., Elend, M., Preiβ, A., Thiele, H., Ingendoh, A.: Even-electron ions: a systematic study of the neutral species lost in the dissociation of quasi-molecular ions. J. Mass Spectrom. 42, 1024–1044 (2007)
Zhang, X., Wang, H., Liao, Y., Ji, H., Guo, Y.: Study of methylation of nitrogen-containing compounds in the gas phase. J. Mass Spectrom. 42, 218–224 (2007)
Kuck, D.: Mass spectrometry of alkylbenzenes and related compounds. Part II. Gas phase ion chemistry of protonated alkylbenzenes (alkylbenzenium ions). Mass Spectrom. Rev. 9, 583–630 (1990)
Kuck, D.: Protonated aromatics and arenium ions. In: Nibbering, N.M.M. (ed.) Encyclopedia of mass spectrometry, 4, pp. 229–242. Elsevier, Amsterdam (2005)
Wood, K.V., Burinsky, D.J., Cameron, D., Cooks, R.G.: Site of gas-phase cation attachment. Protonation, methylation, and ethylation of aniline, phenol, and thiophenol. J. Org. Chem. 48, 5236–5242 (1983)
Nacson, S., Harrison, A.G., Davidson, W.R.: Effect of method of ion preparation on low-energy collision-induced dissociation mass spectra. Org. Mass Spectrom. 21, 317–319 (1986)
Mason, R., Milton, D., Harris, F.: Proton transfer to the fluorine atom in fluorobenzene: a dramatic temperature dependence in the gas phase. J. Chem. Soc. Chem. Commun. 1453–1455 (1987)
Weisz, A., Cojocaru, M., Mandelbaum, A.: Site specific gas-phase protonation of 2-t-butylmaleates and 2-t-butylsuccinates upon chemical ionization: stereochemical effects and kinetic control. J. Chem. Soc. Chem. Commun. 331–332 (1989)
Nakata, H., Suzuki, Y., Shibata, M., Takahashi, K., Konishi, H., Takeda, N., Tatematsu, A.: Chemical ionization mass spectrometry of bifunctional compounds. The behavior of bifunctional compounds on protonation. Org. Mass Spectrom. 25, 649–654 (1990)
Vais, V., Etinger, A., Mandelbaum, A.: Intramolecular proton transfers in stereoisomeric gas-phase ions and the kinetic nature of the protonation process upon chemical ionization. J. Mass Spectrom. 34, 755–760 (1999)
Denekamp, C., Mandelbaum, A.: Proton transfer via strained transition states in the elimination of alcohols from MH+ ions of stereoisomeric diethers and hydroxy esters upon chemical ionization and collision-induced dissociation. J. Mass Spectrom. 36, 422–429 (2001)
Joyce, J.R., Richards, D.S.: Kinetic Control of protonation in electrospray ionization. J. Am. Soc. Mass Spectrom. 22, 360–368 (2011)
Acknowledgments
The authors thank Dr. Y.-P. Tu, GlaxoSmithKline, for many discussions and suggestions. This work was supported by the National Science Foundation of China (no. 21025207).
Author information
Authors and Affiliations
Corresponding author
Electronic supplementary material
Below is the link to the electronic material.
ESM 1
(PDF 740 kb)
Rights and permissions
About this article
Cite this article
Chai, Y., Hu, N. & Pan, Y. Kinetic and Thermodynamic Control of Protonation in Atmospheric Pressure Chemical Ionization. J. Am. Soc. Mass Spectrom. 24, 1097–1101 (2013). https://doi.org/10.1007/s13361-013-0626-9
Received:
Revised:
Accepted:
Published:
Issue Date:
DOI: https://doi.org/10.1007/s13361-013-0626-9