Abstract
Many of the species of Lactobacillus can be considered to be “probiotics” with a variety of benefits, including imparting antioxidative effects to the host. Lactobacillus species evolve different mechanisms to defend themselves against oxygen toxicity, such as superoxide dismutases (SODs), hydroperoxidases and high intracellular levels of metal ions. The SODs provide a cellular defense mechanism against oxidative stress by scavenging O −2 . Most Lactobacillus species appear to lack a sod gene. To date, only two species of Lactobacillus (Lactobacillus casei and L. sakei) may have sod genes, as evidenced by sequence analysis of the genome, but no experimental verification, including cloning and heterologous expression of the Lactobacillus sod gene, has been reported. It is therefore unknown whether these sod genes can express SOD or are functional. We have PCR-amplified the gene from L. casei Lc18 that encodes the SOD using primers designed according to the genome of L. casei ATCC 334 and ligated it into the vector pET-28a(+) for heterologous expression in Escherichia coli BL21(DE3). After being induced with IPTG, the fusion protein was efficiently expressed in a soluble form. The superoxide radical scavenging activity of the recombinant strain was found to be increased relative to that of the control strain. The SOD was also purified by nickel ion affinity chromatography and found to consist of a single band, as determined by SDS-PAGE analysis, with an activity of 39.97 U/mg. N-terminal amino acid sequence analysis indicated that it may be a manganese-containing SOD. This is the first report of a sod gene from Lactobacillus spp. being expressed in other prokaryotes.
Similar content being viewed by others
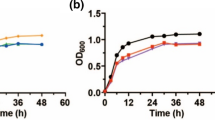
Introduction
Probiotics are living microorganisms that impart health benefits to the host beyond inherent basic nutrition upon ingestion in certain numbers (Guarner and Schaafsma 1998). Lactobacilli are the most widely used probiotics and are considered to be key commensals involved in the promotion of the host’s health. Various studies have indicated that lactobacilli may alleviate lactose intolerance, have a positive influence on the intestinal flora of the host, stimulate or modulate mucosal immunity, reduce blood cholesterol and impart antioxidative effects to the host (Vizoso Pinto et al. 2006; Wang et al. 2009). Among these potential health-promoting benefits, the antioxidative effect is one of the most interesting properties.
Oxidative stress is the adverse effect of oxidants on physiological function. Oxidative stress, which has been implicated in the progression of aging and disease, occurs when abnormally high levels of reactive oxygen species (ROS) are generated, resulting in DNA, protein and lipid damage (Kullisaar et al. 2002). During the past decade, oxidative stress and antioxidative potency have been identified as key points in the molecular regulation of cellular stress responses. Free radicals, such as the superoxide radical anion (O −2 ) and hydroxyl radical (OH.), and other ROS are considered to be highly potent oxidants that can react with all biomacromolecules in living cells and are associated with carcinogenesis and mutagenesis (Kodali and Sen 2008). The radicals may cause oxidative damage by oxidizing biomolecules, thereby resulting in cell death and tissue injury.
Various synthetic and natural antioxidants have been reported (Brioukhanov and Netrusov 2004; Sánchez-Venegas et al. 2009); however, the safety and long-term effects of synthetic antioxidants on health are uncertain. Accordingly, antioxidants from natural sources are both more desirable (Lin and Yen 1999) and more valuable. Among natural antioxidants, live microorganisms have the highest applicability. Lactobacillus spp., which are generally recognized as safe microorganisms with respect to human health (Adams and Marteau 1995), are commonly used in the fermentation of foods, including dairy, meat, vegetable and bakery products. Some of them are reported to have excellent antioxidative activities in the host, where they are able to decrease the risk of an accumulation of ROS during the ingestion of food, while others apparently lack these activities (Korpela et al. 1997).
Some protection against ROS in aerobes and facultative anaerobes (such as Lactobacillus) is provided by antioxidative defense enzymes, one of the most important of which is superoxide dismutase (SOD) (Brioukhanov and Netrusov 2004). The SODs (EC 1.15.1.1), which provide a cellular defense mechanism against the toxicity of oxygen by catalytically scavenging O −2 (Archibald and Fridovich 1981), are metalloenzymes that catalyze the conversion of the superoxide anion into hydrogen peroxide and dioxygen. This significant antioxidative activity is the basis for the increased resistance of some Lactobacillus strains to toxic oxidative compounds, allowing them to survive in the host, and to serve as defensive components in the intestinal microbial ecosystem (Kullisaar et al. 2002).
The SOD enzymes can be distinguished into three types based on the composition of their metal center: manganese, copper–zinc or iron. SODs are found across a broad range of organisms that utilize one, two, or all three types to meet their antioxidant needs (Hassan 1997). The majority of SODs comprise two identical subunits, each containing a metal ion, a disulfide bridge, a sulfhydryl group and an acetylated terminal amino group. The molecular weights of Cu,ZnSODs are approximately 32 kDa, and they are homodimers. Prokaryotes were initially believed to possess FeSODs and/or MnSODs which, unlike the Cu,ZnSODs of eukaryotes, are insensitive to the cyanide radical (CN−). However, Cu,ZnSODs have since also been identified in prokaryotes, and these are also inhibited by CN−. FeSODs and MnSODs are highly homologous and have similar three-dimensional structures, molecular weights of approximately 40 kDa (2 × 20 kDa) and similar metal-binding ligands in the active site of the enzyme (Meile et al. 1995). MnSODs are cambialistic (functionally active with either metal) SODs of anaerobic microorganisms (Brioukhanov and Netrusov 2004); they were first isolated from Escherichia coli and found to be unlike the corresponding enzymes of eukaryotes with respect to all parameters except their catalytic activity (Keele et al. 1970).
Until recently, most lactobacilli were believed to lack SODs (Bruno-Bárcena et al. 2004); however, Lactobacillus plantarum and several other aerotolerant lactobacilli have been identified as possessing a nonenzymatic dismutation system based on the accumulation of high intracellular levels of Mn2+, which can stoichiometrically remove O −2 (Archibald and Fridovich 1981). Analyses of genome sequences indicate that L. casei and L. sakei likely contain the sod gene, but the presence of these genes and the expression and activity of SOD have not yet been demonstrated by experimental methods, such as cloning or heterologous expression.
In this study, the sod gene from Lactobacillus casei Lc18 was heterologously expressed in E. coli BL21(DE3). The fusion protein (SOD enzyme) was then purified and its antioxidative activity detected.
Materials and methods
Bacterial strains and plasmids
The bacterial strains, plasmids and primers used in this study are listed in Table 1. Escherichia coli DH5α was used for gene cloning and E. coli BL21(DE3) was used as a host for the expression of the recombinant proteins. L casei Lc18 and Lc2 and L. acidophilus LA11 and LA12 were provided by Shanghai Jiao Da Onlly Co. (Shanghai, PR China. The L. casei and L. acidophilus strains were cultivated in De Man, Rogosa and Sharpe (MRS) medium without shaking at 37°C for 18 h to reach the stationary phase. The E. coli strains were cultivated in Luria–Bertani (LB) broth with shaking (200 rpm) at 37°C for 12 h to reach the stationary phase. E. coli transformants were selected with 100 μg/ml of ampicillin or 50 μg/ml of kanamycin. As soon as the OD600 of recombinant E. coli BLSod reached 0.6, isopropyl β-D-1-thiogalactopyranoside (IPTG) was added to 1 mM to induce protein expression.
Chemicals and enzymes
Restriction enzymes (NdeI and BamHI), Thermus aquaticus DNA Polymerase, LA Taq polymerase and T4 DNA ligase were purchased from TaKaRa (Shiga, Japan). All chemicals and antibiotics used were purchased from Sigma (St. Louis, MO) unless otherwise specified.
Construction of the expression vector
Lactobacillus genomic DNA was isolated according to Sambrook et al. (2001) with some modifications. Briefly, 50 μl of proteinase K was added during the lysing step, after which the mixture was incubated for 30 min at 37°C, followed by the addition of 600 μl of phenol/chloroform/isoamyl alcohol (25:24:1). The extracted DNA was electrophoresed on a 1% agarose gel, quantified spectrophotometrically at 260 nm and diluted to 50 ng/μl for PCR.
The coding sequence of SOD was subjected to PCR amplification from the chromosomal DNA template of L. casei Lc18 using a forward primer containing a NdeI restriction site SS and a reverse primer containing a BamHI restriction site SR. These two primers were derived from the whole-genome sequences of L. casei ATCC 334 using Primer Premier software, ver. 5.0 (PREMIER Biosoft Int, Palo Alto, CA ).
The PCR reactions were carried out in a 50-μl volume containing 100 ng DNA template solution, 5 μl of 10× LA PCR Buffer II (Mg2+ plus), 2.5 U of LA Taq Polymerase, 10 nmol of each deoxyribonucleoside triphosphate and 10 pmol of each primer. The PCR reaction was performed under following conditions: an initial denaturation step at 94°C for 5 min followed by 30 cycles of denaturation at 94°C for 1 min, annealing at 58°C for 40 s, and extension at 72°C for 40 s, with a final elongation step at 72°C for 10 min. All PCRs were carried out with Eppendorf Mastercycler ep gradient S (Hamburg, Germany). The PCR product, which was 700 bp, as expected, was purified by using the Agarose Gel DNA Purification kit ver.2.0 (TaKaRa), ligated into the vector pMD19-T and used to transform competent cells of E. coli DH5α with heat shock according to the manufacturer’s instructions. Transformants were identified using primers M13-47 and RV-M. The nucleotide sequences were determined by AmlipTaq FS DNA polymerase fluorescent dye terminator reactions by means of an Applied Biosystems 3730 automated sequencer (Foster City, CA).
The purified PCR products were digested with NdeI and BamHI and ligated to the pET-28a(+) expression vector, yielding the pETsod plasmid (Fig. 1). Restriction enzymes, T4 ligase and other DNA-modifying enzymes were used according to the protocols of the manufacturers. The recombinant plasmid pETsod was introduced into competent E. coli BL21(DE3) according to Tu et al. (2005). Three positive transfomants were grown in liquid LB medium containing 50 μg/ml kanamycin and identified through PCR and restriction enzyme digestion.
Preparation of dialyzed cell-free extracts
Fresh single colony-forming units (CFUs) of each test strain were inoculated into 10 ml of proper culture medium. After incubation at 37°C, total cell numbers were adjusted to 1010 CFU/ml, then cells of each strain were harvested by centrifugation at 5000 g for 10 min. Cell pellets were then quickly washed twice and resuspended in deionized water followed by ultrasonic disruption (5 min, 200 W, 50% working time) on a Sonicator JY96-II (Scientz Biotechnology, Ningbo, China) with constant cooling on ice, The supernatant (cell-free extract, CFE) was recovered and used immediately in subsequent experiments.
Antioxidant activity assays
Measurement of superoxide radical scavenging activity
Superoxide radical scavenging activity was measured by the method of Nishikimi et al. (1972). Briefly, 1 ml of nitroblue tetrazolium (NBT) solution (156 μM in 100 mM phosphate buffer, pH 7.4), 1 ml of coenzyme I reduced disodium salt (NADH) solution (468 μM in 100 mM phosphate buffer, pH 7.4) and 0.1 ml of fresh sample or distilled water (control) were mixed. The reaction was started by adding 100 μl of phenazine methyl sulfate (PMS) solution (60 μM PMS in 100 mM phosphate buffer, pH 7.4) to the mixture. The mixture was then incubated at 25°C for 5 min and the absorbance measured at 560 nm. Decreased absorbance of the reaction mixture indicated increased superoxide anion scavenging activity. The scavenging activity of superoxide radical was calculated as follows:
Measurement of hydrogen peroxide scavenging activity
Hydrogen peroxide scavenging activity was measured according to Pick and Keisari (1980) with minor modification. Briefly, 50 μl of 5 mM H2O2 solution and 50 μl of fresh sample or distilled water (control) were mixed and incubated at room temperature for 20 min. Then, 100 μl of horseradish peroxidase (HRP)–phenol red solution (300 μg/ml HRP and 4.5 mM phenol red in 100 mM phosphate buffer) was added and after 10 min of incubation, the sample absorbance was monitored at 610 nm. The scavenging effect was calculated according to the following equation:
Expression, purification and sodium dodecyl sulfate-polyacrylamide gel electrophoresis analysis of the recombinant protein
A single colony of recombinant E. coli BLSod was inoculated in 2 ml LB medium containing 50 μg/ml kanamycin and cultivated at 37°C at 200 rpm. The overnight culture was diluted 100-fold into 50 ml of fresh LB medium containing 50 μg/ml kanamycin, grown to an OD600 of about 0.6 and then induced with 1 mM isopropylthiogalactoside (IPTG). The culture was subsequently incubated to express the fusion protein. After 9 h, wet cells were collected from 50 mL of bacteria culture by centrifugation and resuspended in 5 ml of Native Binding Buffer (50 mM NaH2PO4, 0.5 M NaCl, pH 8.0); 8 mg lysozyme was then added and the mixture incubated on ice for 30 min and subjected to ultrasonication. In order to determine whether the recombinant SOD protein was expressed, or not, and if expressed whether it is in the form of inclusion bodies, the bacteria cells were sonicated and separated into soluble and insoluble fractions by centrifugation at 10,000 g at 4°C for 10 min. Both the soluble and insoluble fractions were analyzed by 12% sodium dodecyl sulfate–polyacrylamide gel electrophoresis (SDS–PAGE) according to the protocol of the manufacturer (Bio-Rad, Hercules, CA). The ultrasonicated supernatant (soluble fraction) was then subjected to His-tag purification and purified by the Ni-NTA Purification system (Invitrogen, Carlsbad, CA) according to the manufacturer’s instructions. Both the crude and purified protein fractions were boiled for 5 min and analyzed by SDS–PAGE. Proteins were visualized by Coomassie brilliant blue staining.
Measurement of purified SOD activity
Enzyme activity was measured as described above. One unit of SOD activity was defined as the amount of purified enzyme that consumed 1 μmol of PMS from the substrate per minute. The specific activity was defined as units of activity per milligram of purified protein (purified SOD).
The protein concentrations were determined by the Lowry method (Liong and Shah 2005) using bovine serum as the standard.
Amino acids sequence analysis
The deduced amino acid sequence of the sod gene was analyzed using BioEdit software ver. 7.0.1.
Nucleotide sequence accession number
The sod gene nucleotide sequences and the 16S rDNA gene of L. casei Lc18 and Lc2 has been deposited in the GenBank database under accession numbers HM070825, HM070024, HM070025.
Results
Construction and Identification of recombinants
The 700-bp PCR-fragment cloned from L. casei Lc18 was identified as the sod gene by a BLAST search of the GenBank database. The cloned sod gene contained an 618-bp open reading frame (ORF) that encoded a protein of 205 amino acids with a predicted molecular weight of 23.3 kDa. The recombinant plasmid pETsod was demonstrated to be correct by restriction enzyme digestion (Fig. 2) and DNA sequencing (nucleotide sequence accession number HM070825). Figure 2 shows that the recombinant plasmid pETsod was successfully constructed and transformed into E. coli BL21(DE3) to form the recombinant strain E. coli BLSod.
Expression and purification of the recombinant protein
Using the T7 promoter and the N-terminal His-tag of pETsod in recombinant E. coli BLSod, the expression of the fusion protein was induced by incubation in the presence of 1 mM IPTG at 37°C for 9 h when the OD600 reached 0.6. SDS–PAGE analysis of the soluble and insoluble fractions revealed that most of the induced protein was present in the soluble fraction. SDS–PAGE also revealed a distinct band of the expected size (about 24 kDa) in the recombinant E. coli BLSod lane following induction with 1 mM IPTG (Fig. 3, lane 4) and the absence of this band in the controls, namely, BL21(DE3) (Fig. 3, lane 1), BL21(DE3) harboring pET-28a(+) (Fig. 3, lane 2) and BLSod without induction (Fig. 3, lane 3). These findings indicate that the SOD protein may be expressed normally in E. coli BLSod with the induction of IPTG. We then used the Ni-NTA purification system to confirm these results. Following nickel ion metal affinity chromatography, the final eluate (the purified target protein) produced a single distinct band (Fig. 3, lane 7) that had the same size as the expected SOD (about 24 kDa) in lane 4 of Fig. 3; there was no similar band in the other two controls, BL21(DE3) (Fig. 3, lane 5) and BL21(DE3) harboring pET-28a(+) (Fig. 3, lane 6). An enzyme activity test of the purified SOD revealed that the enzyme activity of O −2 scavenging was 39.97 U/mg. An additional band of about 27 kDa was visualized in both lanes 4 and 7, which was interpreted as an endogenous protein of BL21(DE3) containing several seriate histidines that was induced by IPTG.
Sodium dodecyl sulfate–polyacrylamide gel electrophoresis analysis of SOD heterologous expression in Escherichia coli BLSod. Isopropyl β-D-1-thiogalactopyranoside (IPTG) was added to the growth medium at a concentration of 1 mM for SOD induction, and the culture was then incubated at 37°C for 9 h prior to the analysis of cellular proteins. Lanes: M pre-stained protein molecular weight marker, 1 total protein of control strain [E. coli BL21(DE3)], 2 total protein of control strain [E. coli BL21(DE3) harboring pET-28a(+)], 3 total protein of recombinant strain BLSod [E. coli BL21(DE3) harboring pETsod] without induction of IPTG, 4 total protein of recombinant strain BLSod [E. coli BL21(DE3) harboring pETsod] with induction of IPTG, 5 purified protein of control strain [E. coli BL21(DE3)] by Ni-NTA purification system, 6 purified protein of control strain [E. coli BL21(DE3) harboring pET-28a(+)] by Ni-NTA purification system, 7 purified recombinant SOD of strain BLSod with IPTG induction by Ni-NTA purification system. Arrows Target SOD proteins
Antioxidative activity of the tested strains
Table 2 shows antioxidative activities, including superoxide radical scavenging activity and hydrogen peroxide scavenging activity, from both intact cells and CFEs of E. coli BL21 (DE3), E. coli BLSod, L. casei Lc18, L. casei Lc2, L. acidophilus LA11 and L. acidophilus LA12.
The results suggested that both the intact cells and CFEs of all six strains had some antioxidative ability and that the abilities of the CFEs were generally higher than those of intact cells. The antioxidative abilities of both L. acidophilus are quite low compared to the other four strains. The superoxide radical scavenging activity of E. coli increased from 37.17 to 43.24% (intact cells) and from 44.31 to 55.20% (CFEs) after recombination with the sod gene; the hydrogen peroxide scavenging activity also increased, from 52.08 to 55.37% (intact cells) and from 51.04 to 58.15% (CFEs) after recombination with sod gene.
Sequence analysis and comparison
The results of the antioxidant activity assays and recombinant protein analysis demonstrated that the sod gene was amplified successfully from the genome DNA of L. casei Lc18. A BLAST search of the GenBank database revealed that the sod gene of L. casei Lc18 is quite different from that of many other species, including humans, animals, plants and other bacteria. However, a homology comparison of the sequence of the L. casei Lc18 sod gene with other SOD protein sequences revealed that the SOD protein sequence deduced from the sod gene of L. casei Lc18 is more similar to the three MnSOD protein sequences from Streptococcus thermophilus (Bruno-Bárcena et al. 2004), L. lactis (Sanders et al. 1995) and E. coli (Roy et al. 1993) (Fig. 4) than to the two FeSODs from Pseudomonas ovalis (Isobe et al. 1987) and Photobacterium leiogathi (Barra et al. 1987). The iron- and manganese-containing SODs can be distinguished by analysis of their primary structures (Parker and Blake 1988). The size of the L. casei Lc18 SOD (205 amino acid residues) is within the range of the size of SODs from the five aforementioned bacteria (194–206 residues) (Fig. 4). The amino acids involved in the binding of metal ligands are present in the L. casei Lc18 SOD (His-27, His-82, Asp-174 and His-178; Fig. 4), as are the postulated active-site residues (Parker et al. 1987; Parker and Blake 1988). The L. casei Lc18 SOD contains the typical residues (Gly-76, Gly-77, Phe-85, Gln-153, Asp-154; Fig. 4) of MnSODs and none of the typical residues (Gla-76, Gln-77, Thr-85, Gla-153, Gly-154; Fig. 4) of FeSODs (Parker and Blake 1988), which indicates that the L. casei Lc18 SOD belongs to the MnSODs.
Discussion
The SODs are very important because they provide a cellular defense mechanism against the toxicity of oxygen by catalytically scavenging O −2 . During the past 20 years, many sod genes have been cloned from various organisms, including E. coli (Roy et al. 1993), L. lactis (Sanders et al. 1995), P. ovalis (Isobe et al. 1987), S. thermophilus (Bruno-Bárcena et al. 2004), Trichoderma harzianum (Yang et al. 2009), Deschampsia antarctica (Sánchez-Venegas et al. 2009), and humans (Tibell et al. 1987). For some widely used probiotics, such as L. acidophilus, that have no SOD and low antioxidative activities, heterologous expression of the sod gene from L. casei or L. sakei is of critical importance in the construction of genetic engineering of Lactobacillus with higher SOD activity. The functional gene from L. casei is more suitable for expression in other Lactobacillus because L. casei is a Gram-positive non-spore-forming organism belonging to the same group that has a low GC content; as such the L. casei sod gene is close to that of other strains in the Lactobacillus group. In this study, the sod gene from Lactobacillus was heterologously expressed in another prokaryote for the first time.
The antioxidative effects of every bacterial strain tested in this study were examined in intact cells and in intracellular extracts (CFEs). The results shown in Table 2 show that all of the tested bacterial strains had some antioxidative activity and that the antioxidative effects of disrupted cells were higher than those of intact cells. A similar finding was previously reported by Saide and Gilliland (2005) who found that intracellular extracts of lactobacilli possessed higher antioxidative activity than intact cells. The higher antioxidative activity of the intracellular extracts could be due to the better accessibility of antioxidative enzymes or metal ions to the oxidant substrates.
Although the antioxidative activities of L. casei Lc2 were higher than those of L. casei Lc18, we failed to amplify the sod gene from L. casei Lc2 using the same PCR conditions employed for L. casei Lc18. The PCR primers were designed based on the genome of L. casei ATCC 334. Therefore, the inability to amplify the sod gene from L. casei Lc2 may indicate that this strain was genetically altered during its evolution or did not contain a sod gene at all. However, this strain still displayed high superoxide radical scavenging activity and hydrogen peroxide scavenging activity, which might have been due to the high concentration of Mn2+ in its cells or other unknown antioxidative mechanisms.
The O −2 scavenging activity and H2O2 scavenging activity of the recombinant E. coli BLSod was slightly higher than that of E. coli BL21(DE3). The slight increase in hydrogen peroxide scavenging activity may be explained by the positive interaction between SOD and catalase. Superoxide radicals can inactivate catalase; therefore, SOD can protect catalase due to its O −2 scavenging activity (Brioukhanov and Netrusov 2004).
The aim of this study was to clone the sod gene from L. casei into E. coli BL21(DE3) for ectopic expression in order to demonstrate that the SOD from Lactobacillus is able to function in a heterologous prokaryote with considerable enzyme activity. Further work is required to clone the sod of L. casei into other species of Lactobacillus, such as L. acidophilus, which is widely used as a probiotic but lacks the SOD protein and has low antioxidative activity.
References
Adams MR, Marteau P (1995) On the safety of lactic acid bacteria from food. Int J Food Microbiol 27(2–3):263–264
Archibald FS, Fridovich I (1981) Manganese, superoxide dismutase, and oxygen tolerance in some lactic acid bacteria. J Bacteriol 146(3):928–936
Barra D, Schininà ME, Bannister WH, Bannister JV, Bossa F (1987) The primary structure of iron-superoxide dismutase from Photobacterium leiognathi. J Biol Chem 262(3):1001–1009
Brioukhanov AL, Netrusov AI (2004) Catalase and superoxide dismutase: distribution, properties, and physiological role in cells of strict anaerobes. Biochemistry 69(9):949–962
Bruno-Bárcena JM, Andrus JM, Libby SL, Klaenhammer TR, Hassan HM (2004) Expression of a heterologous manganese superoxide dismutase gene in intestinal lactobacilli provides protection against hydrogen peroxide toxicity. Appl Environ Microbiol 70(8):4702–4710
Guarner F, Schaafsma GJ (1998) Probiotics. Int J Food Microbiol 39(3):237–238
Hassan HM (1997) Cytotoxicity of oxyradicals and the evolution of superoxide dismutases. In: Massaro D, Clerch L (eds) Oxygen, gene expression and cellular function. Marcel Dekker, New York, pp 25–52
Isobe T, Fang YI, Muno D, Okuyama T, Ohmori D, Yamakura F (1987) Amino acid sequence of iron-superoxide dismutase from Pseudomonas ovalis. FEBS Lett 223(1):92–96
Keele BB Jr, McCord JM, Fridovich I (1970) Superoxide dismutase from Escherichia coli B. A new manganese-containing enzyme. J Biol Chem 245(22):6176–6181
Kodali VP, Sen R (2008) Antioxidant and free radical scavenging activities of an exopolysaccharide from a probiotic bacterium. Biotechnol J 3(2):245–251
Korpela R, Peuhkuri K, Laehteenmaeki T, Sievi E, Saxelin M, Vapaatalo H (1997) Lactobacillus rhamnosus GG shows antioxidative properties in vascular endothelial cell culture. Milchwissenschaft 52(9):503–505
Kullisaar T, Zilmer M, Mikelsaar M, Vihalemm T, Annuk H, Kairane C, Kilk A (2002) Two antioxidative lactobacilli strains as promising probiotics. Int J Food Microbiol 72(3):215–224
Lin MY, Yen CL (1999) Antioxidative ability of lactic acid bacteria. J Agric Food Chem 47(4):1460–1466
Liong MT, Shah NP (2005) Bile salt deconjugation ability, bile salt hydrolase activity and cholesterol co-precipitation ability of lactobacilli strains. Int Dairy J 15(4):391–398
Meile L, Fischer K, Leisinger T (1995) Characterization of the superoxide dismutase gene and its upstream region from Methanobacterium thermoautotrophicum Marburg. FEMS Microbiol Lett 128(3):247–253
Nishikimi M, Appaji N, Yagi K (1972) The occurrence of superoxide anion in the reaction of reduced phenazine methosulfate and molecular oxygen. Biochem Biophys Res Commun 46(2):849–854
Parker MW, Blake CC (1988) Iron- and manganese-containing superoxide dismutases can be distinguished by analysis of their primary structures. FEBS Lett 229(2):377–382
Parker MW, Blake CC, Barra D, Bossa F, Schinina ME, Bannister WH, Bannister JV (1987) Structural identity between the iron- and manganese-containing superoxide dismutases. Protein Eng 1(5):393–397
Pick E, Keisari Y (1980) A simple colorimetric method for the measurement of hydrogen peroxide produced by cells in culture. J Immunol Methods 38(1–2):161–170
Roy DG, Klaenhammer TR, Hassan HM (1993) Cloning and expression of the manganese superoxide dismutase gene of Escherichia coli in Lactococcus lactis and Lactobacillus gasseri. Mol Gen Genet 239(1–2):33–40
Sánchez-Venegas JR, Navarrete A, Dinamarca J et al (2009) Cloning and constitutive expression of Deschampsia antarctica Cu/Zn superoxide dismutase in Pichia pastoris. BMC Res Notes 2:207
Saide JA, Gilliland SE (2005) Antioxidative activity of Lactobacilli measured by oxygen radical absorbance capacity. J Dairy Sci 88(4):1352–1357
Sambrook J, MacCallum P, Russell D (2001) Molecular cloning: a laboratory manual, 3rd edn. Cold Spring Harbor Press, New York
Sanders JW, Leenhouts KJ, Haandrikman AJ, Venema G, Kok J (1995) Stress response in Lactococcus lactis: Cloning, expression analysis, and mutation of the lactococcal superoxide dismutase gene. J Bacteriol 177(18):5254–5260
Tibell L, Hjalmarsson K, Edlund T, Skogman G, Engström A, Marklund SL (1987) Expression of human extracellular superoxide dismutase in Chinese hamster ovary cells and characterization of the product. Proc Natl Acad Sci USA 84(19):6634–6643
Tu Z, He G, Li X, Chen J, Chang J, Chen L, Yao Q, Liu P, Ye H, Shi J, Wu X (2005) An improved system for competent cell preparation and high efficiency plasmid transformation using different Escherichia coli strains. Electron J Biotechnol 8(1):113–120
Vizoso Pinto MG, Franz CM, Schillinger U, Holzapfel WH (2006) Lactobacillus spp. with in vitro probiotic properties from human faeces and traditional fermented products. Int J Food Microbiol 109(3):205–214
Wang AN, Yi XW, Yu HF, Dong B, Qiao SY (2009) Free radical scavenging activity of Lactobacillus fermentum in vitro and its antioxidative effect on growing-finishing pigs. J Appl Microbiol 107(4):1140–1148
Yang LM, Yang Q, Li S, Sun KN, Tian Y (2009) Cloning, expression and biological activity identification of Cu-Zn SOD from Trichoderma harzianum. Harbin Gongye Daxue Xuebao/J Harbin Inst Technol 41(3):102–107
Acknowledgments
This work was supported by the National Hi-Tech Research and Development Program of China (“863” Program, grant 2007AA10Z355), the National Basic Research Program of China (“973” Program, grant 2007CB714301) and the Medical-Engineering Joint Research Foundation of Shanghai Jiao Tong University (grant YG2009MS11).
Author information
Authors and Affiliations
Corresponding author
Rights and permissions
About this article
Cite this article
Liu, Q., Hang, X., Liu, X. et al. Cloning and heterologous expression of the manganese superoxide dismutase gene from Lactobacillus casei Lc18. Ann Microbiol 62, 129–137 (2012). https://doi.org/10.1007/s13213-011-0237-2
Received:
Accepted:
Published:
Issue Date:
DOI: https://doi.org/10.1007/s13213-011-0237-2