Abstract
This study analyzed the interaction between the phosphate-solubilizing fungus (PSF) Talaromyces flavus and the arbuscular mycorrhizal fungus (AMF) Gigaspora rosea in vitro, and whether the in vivo application of T. flavus was able to stimulate the efficiency of the symbiosis between G. rosea and wheat (Triticum aestivum). In vitro, the soluble chemical substances released by T. flavus promoted the development of pre-infective mycelium from germinating AMF spores, increasing the length of each branch and the number of branches. In vivo, the inoculation of T. flavus increased plant wet and dry weight of mycorrhizal plants, regardless of the P conditions. AMF root colonization was inhibited under high P conditions but was promoted by T. flavus inoculation. The inoculation of T. flavus also improved the symbiotic efficiency of mycorrhizal plants, measured as APA, and increased the total plant phosphate content and shoot:root phosphate ratio in mycorrhizal plants. To our knowledge, this is the first report where exudates produced by a PSF as T. flavus promote pre-infective development, root colonization and symbiotic efficiency of G. rosea in wheat. Finally, the role of T. flavus in rhizosphere interactions is discussed.
Similar content being viewed by others
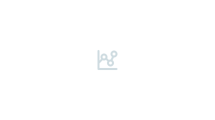
Explore related subjects
Discover the latest articles, news and stories from top researchers in related subjects.Avoid common mistakes on your manuscript.
1 Introduction
Phosphorus (P) is one of the essential nutrients required by plants and microorganisms for their growth and development. This nutrient has low bioavailability in the soil because phosphate anions may be immobilized through sorption and precipitation with cations (Garg and Bahl 2008; Antoun 2012). Immobilized anions can be released into the soil through biological solubilization processes, such as the production of organic acids and a decrease in pH by some bacteria and fungi, the latter known as P-solubilizing fungi (PSF) (Rodriguez and Fraga 1999; Antoun 2012). Several studies have shown that the application of PSF as bioinoculants can improve plant P nutrition and growth (Richardson 2001, 2007; Richa et al. 2007).
Arbuscular mycorrhizal fungi (AMF) are mutualistic symbionts that colonize plant roots. By means of the interaction, plants increase the absorption surface and AMF receive photosynthates (Landeweert et al. 2001). AMF also transport soluble P from the soil solution to the roots, thus improving P plant nutrition (Richardson et al. 2009). The symbiotic efficiency is an important factor that determines the final effect on plant growth and P nutrition. Tisserant et al. (1993) proposed the intraradical alkaline phosphatase activity (APA) as a marker of the symbiotic efficiency of plant root colonization by AMF. It is known that the development of the mycorrhizal symbiosis is modified by the exudates of rhizospheric microorganisms, such as PSF which indirectly modify the fungal colonization of plants (Fracchia et al. 2004; Scervino et al. 2008). PSF solubilize P and modulate mycorrhizal colonization (Barea et al. 1997; Arshad and Frankenberger 1998).
Gigaspora rosea is an AMF that promotes the growth of various plant species (Hernández-Martínez et al. 2006; Khan et al. 2007), and has relatively large spores that facilitate in vitro microbial interaction studies. Talaromyces flavus is a PSF that can increase soluble P, act as a biocontrol fungus and protect plants against microbial diseases (Naraghi et al. 2010a, b). However, the in vitro and in vivo interaction between T. flavus and G. rosea is not well studied.
The aim of the present study was to analyze in vitro the relationship between the PSF T. flavus and the AMF G. rosea and verify in vivo whether the application of T. flavus is able to stimulate the symbiotic efficiency in Triticum aestivum.
2 Materials and methods
2.1 T. flavus: growth, P release and fungal P solubilization capacity
A strain of T. flavus isolated from wheat rhizospheric soil by Rodríguez (2004) and selected for its P-solubilizing activity by Scervino et al. (2010) was used in this study. This strain was cultured in Malt Extract Agar (MEA) for 5 days at 28 °C in darkness.
To characterize the growth of T. flavus and to quantify the amount of soluble P, a growth curve was performed. Erlenmeyer flasks (100 mL) were filled with 20 mL of liquid National Botanical Research Institute’s phosphate growth medium (NBRIP, Nautiyal 1999) with the addition of MES buffer (pH 6.5) in triplicate. This medium contains (L−1): glucose 10 g, MgSO4 0.12 g, KCl 0.2 g, MgCl2.6H2O 5 g, (NH4)2SO4 0.1 g and Ca3(PO4)2 5 g as an insoluble P source. The inoculated Erlenmeyer flasks were placed on an orbital shaker at 250 rpm and 28 °C.
Cultures were harvested at 6, 12, 18, 24, 48, 72, 120 and 144 h. At each sample time, mycelium was obtained by filtering the culture on a Whatman No. 1 paper. Mycelial dry weight (MDW) was measured by drying the samples for 48 h at 80 °C until constant weight. To remove Ca3(PO4)2 excess, supernatants were centrifuged at 10,600 g for 20 min. The pH was measured by means of a pH meter and the soluble P quantified by spectrophotometric measurement at 405 nm using the ascorbic acid molybdenum blue method (Murphy and Riley 1962). The fungal solubilization capacity (SC) was calculated with the following formula: SC = μg P / g MDW, where SC: solubilization capacity (μg P × g MDW−1), P: amount of soluble P released, and MDW: mycelial dry weight. If the SC ratio is constant over time, there is a direct relationship between the amount of P released and fungal biomass.
2.2 Effect of T. flavus exudates on the development of mycelium from G. rosea spores
G. rosea spores were isolated (Gerdemann 1955) from an open pot culture grown with T. aestivum as a host. Spore surfaces were sterilized using a Chloramine-T 2 %, Streptomycin 0.1 % and Tween 80 0.04 % solution for 13 min, rinsed with sterile distilled water (Mosse 1962) and transferred to Petri dishes (5 cm) containing 10 mL of sterile Phytagel semi-liquid medium (0.4 % Phytagel, Sigma-Aldrich P8169) amended with different concentrations (0, 0.1, 0.5, 1 and 3 %V/V) of sterile T. flavus exudates or culture medium (as control). T. flavus was grown in standard medium (Fracchia et al. 2003) for 144 h under agitation. The standard medium consisted of (L−1): PO4H2K 0.5 g, glucose 10 g and L-asparagine 4 g, and was used due to the lack of interference in normal development of mycelium from AMF spores. The supernatants were sterilized using Millipore filters (0.22 μm). Five Petri dishes were prepared for each concentration, with 10 spores each, and placed in the dark at 25 °C. The dishes were checked for spore germination under the microscope every 2 days for 2 weeks, then dried at 60 °C and stained with Trypan Blue-lactic acid (0.05 %) to estimate the percentage of germination, hyphal length and number of hyphal branches (Scervino et al. 2005). Hyphal length was measured using the intersection method (Brundrett et al. 1994). Mycelium morphology was characterized by means of the ratio between hyphal length and number of branches, to test for changes in the spatial distribution of the AMF.
2.3 Effect of T. flavus inoculation on the G. rosea-T. aestivum symbiosis
A greenhouse experiment was performed to analyze the in vivo effect of T. flavus on the G. rosea-T. aestivum symbiosis. The soil used was collected from the Experimental Field of the Facultad de Ciencias Exactas y Naturales of the University of Buenos Aires (−34° 32′ 47.04′ S, −58° 26′ 24.83′ W), Argentina, and sieved with a 1-cm2 mesh. The soil composition was: total C 12.08 g × kg−1, total N 1.10 g × kg−1, P 34.2 mg × kg−1, and cationic exchange capacity (CEC) 13.4 cmol × kg−1.
The experiment was carried out in 1-L pots filled with a sterile mix of soil and perlite at 2:1 V/V (1 h at 121 °C for 3 days), one half supplemented with (+P) and the other half without (−P) tribasic calcium P. Insoluble P was added to a final concentration of 30 mg P × kg−1 (Omar 1998). Treatments were: non-inoculated, inoculation with G. rosea, inoculation with T. flavus and co-inoculation with G. rosea and T. flavus, under two P levels (−P and + P) with five replicates per treatment. Wheat seeds (bread wheat cultivar, provided by Rizobacter Argentina S. A.) were surface-sterilized with a sodium hypochlorite 30 % and Triton X-100 0.01 % solution for 3 min, rinsed with sterilized distilled water and sown in moistened chambers. After 3 days, uniform seedlings were transplanted to the pots.
Plants were inoculated with 5 mL of an aqueous suspension containing 103 × mL−1 conidia of T. flavus (Omar 1998) and/or 5 g of the G. rosea inoculum. The G. rosea inoculum consisted of rhizospheric soil from a T. aestivum open pot culture, which contained G. rosea spores (21 spores × g−1), mycelia and colonized root fragments. Soil filtrate (Whatman N°1 filter paper) from the rhizosphere of mycorrhizal plants was added to the treatments without G. rosea inoculation. The filtrate contained common soil microorganisms, including bacteria and fungi, but no propagules of G. rosea.
The greenhouse experiment was conducted under controlled light and temperature (16/8 h light/dark, 25/18 °C day/night) conditions. During the experiment, the soil was kept at 80 % of field capacity by weight and supplemented with 50 mL of Hewitt (1966) solution without P every 20 days. Plants were grown for 9 weeks and harvested at the end of this period. Two hundred samples of each root (1 cm) were taken randomly before drying. The percentage of root length colonization (Phillips and Hayman 1970) was quantified and the percentage of APA associated with the intraradical mycelium (Tisserant et al. 1993) measured.
Total wet and dry plant weight (TWW and TDW respectively), total plant P content (TPP), shoot:root P ratio and soil water-extractable P (WEP) were measured. To estimate TDW, the plants were dried at 80 °C for 48 h until constant weight. TPP was estimated by drying the plants at 80 °C, which were then powdered (<1 mm). After that, samples were calcinated at 500 °C for 5 h. The remaining ashes were moistened with distilled water (1 mL). P was extracted by adding 10 mL HCl 2 M and heating up to the boiling point. After cooling, the extract was filtered through a 0.45-μm membrane and P was quantified by spectrophotometric measurement at 405 nm (Sadzawka et al. 2007), using the ascorbic acid molybdenum blue method (Murphy and Riley 1962). Soil WEP was measured using the Water Extractable Phosphate method (Kovar and Pierzynski 2009).
2.4 Data analysis
Data obtained were compared by two-way analysis of variance (ANOVA), one-way ANOVA and correlation tests using the statistical software Statistica 7. All assumptions were tested before ANOVA analysis. When significant differences (p < 0.05) as a result of the treatments were found, post-hoc comparisons were made to analyze the differences among treatments. Means were compared by Fisher’s least significant differences (LSD) test, and main effects were studied every time when no differences were found (experiments of root length colonization and shoot P:root P ratio).
3 Results
3.1 T. flavus: growth, P release and fungal P solubilization capacity
During the P solubilization assay, fungal biomass ranged from 0.014 ± 0.003 g to 0.102 ± 0.001 g of MDW. The amount of P released followed the fungal growth, showing the highest solubilization at 96 h (Fig. 1a). The fungal SC (μg P released × g−1 MDW) was constant along the entire experiment (Fig. 1b). The pH values decreased from 6.49 ± 0.01 to 5.33 ± 0.09 throughout the experiment (Fig. 1c) and the statistical analysis showed a negative correlation between the pH values and the amount of P released (Fig. 1d).
3.2 Effect of T. flavus exudates on the development of mycelium from G. rosea spores
The presence of T. flavus exudates had no effect on G. rosea spore germination (data not shown) but showed a significant effect on G. rosea pre-infective mycelial development (one-way ANOVA p < 0.05; Fig. 2).
Effect of Talaromyces flavus exudates on Gigaspora rosea pre-infective development. a) Hyphal length (mm) (F = 5.23; P = 0.001). b) Number of hyphal branches (F = 7.19; P = 0.0001). c) Mycelial morphology calculated as hyphal length per branch (F = 2.57; P = 0.04). Vertical bars are SD. Columns with the same letters above them indicate column values that are not significantly different, as determined by Fisher’s LSD test (P < 0.05)
Sterile exudates of T. flavus (1 and 3 % V/V) increased four-fold the hyphal length of germinating G. rosea spores (F = 5.23; p = 0.001) (Fig. 2a) and three-fold the hyphal branching (as the number of hyphal tips) compared with the control at concentrations above 0.5 % (F = 7.19; p = 0.0001) (Fig. 2b). G. rosea mycelium morphology changed with 1 and 3 % of T. flavus exudates compared with the control (F = 2.57; p = 0.04) (Fig. 2c). Development of G. rosea mycelium from germinating spores under different T. flavus culture medium concentrations (0.1, 0.5, 1 and 3 %) did not differ from those with 0 % fungal exudates (data not shown).
3.3 Effect of T. flavus inoculation on the G. rosea-T. aestivum symbiosis
We found no significant interaction (F = 4.35; p = 0.07) between the fungal inoculation (G. rosea, T. flavus and co-inoculation) and the P condition (−P and + P) for AMF root colonization. The main effects showed that the absence of P amendment led to an increase in the mycorrhizal root length colonization (F = 12.8; p = 0.007) (Fig. 3a). On the other hand, the inoculation of T. flavus increased AMF root colonization (F = 22.75; p = 0.001) (Fig. 3b). AMF colonization was only present in treatments with G. rosea inoculation (data not shown).
Effect of Talaromyces flavus inoculation on Gigaspora rosea root colonization. Main effect of the factors (Treatments: G. rosea, G. rosea-T.flavus × P condition: −P, +P). a) Root length colonization observed in P condition factor (F = 12.8; P = 0.007). b) Root length colonization observed in Treatments factor (F = 22.75; P = 0.001). Vertical bars denote 0.95 confidence intervals. Values with the same letter are not significantly different, as determined by Fisher’s LSD test (P < 0.05)
The symbiotic efficiency of G. rosea-T. aestivum, measured as the percentage of intraradical APA, increased with T. flavus inoculation, regardless of the P conditions. This difference was still greater when P was added to the soil (F = 6.48; p = 0.03). The amendment with insoluble P did not modify the mycorrhizal APA only when G. rosea was present. Furthermore, T. flavus inoculation increased soil WEP when insoluble P was added (F = 75.21; p < 0.0001), while in the absence of T. flavus this parameter did not change (Fig. 4).
Effect of Talaromyces flavus inoculation on alkaline phosphatase activity (APA) and water extractable phosphate (WEP). Columns show the %APA associated with intraradical mycorrhizal mycelia (F = 6.48; P = 0.03). The secondary axis shows the soil WEP under two P conditions: −P (dotted line) or + P (filled line) (F = 75.21; P < 0.0001). Columns with the same letters above them (APA) or asterisk absence (WEP) indicate values that are not significantly different, as determined by Fisher’s LSD test (P < 0.05)
TPP and plant growth measured by means of TWW and TDW showed significant differences with the factors’ combination. Under -P conditions, the co-inoculation of both microorganisms led to an increase in plant TWW compared to plants inoculated only with T. flavus and non-inoculated plants. Also, the plants with the lowest TWW values were those without inoculation (regardless of the P condition) or inoculated only with G. rosea and grown in + P conditions (F = 5.32; p = 0.02). TWW increased with the co-inoculation of both microorganisms in –P conditions. When T. flavus was added to + P soil, TDW increased (F = 11.65; p = 0.001). The presence of both microorganisms in –P conditions increased TPP. Under + P conditions, T. flavus produced the highest TPP values, while G. rosea inoculation showed the lowest values for all plant parameters (F = 8.86; p = 0.04; Table 1).
The results showed no significant interaction (F = 0.48; p = 0.6) between the fungal inoculation (G. rosea, T. flavus and co-inoculation) and the P condition (−P and + P) for the symbiotic efficiency measured as the shoot P:root P ratio. Therefore, regardless of the P condition, the combination of G. rosea and T. flavus led to a synergic increase in the shoot P:root P ratio (F = 9.13; p = 0.003). On the other hand, the inoculation of T. flavus or G. rosea did not change the shoot P:root P ratio compared to control (non-inoculated, Fig. 5).
Effect of Talaromyces flavus inoculation on the shoot P:root P ratio (SP:RP). Main effect of the Treatments factor (F = 9.13; P = 0.003). Treatments are: non-inoculated (NI), Gigaspora rosea, Talaromyces flavus and their co-inoculation. Vertical bars denote 0.95 confidence intervals. Values with the same letter are not significantly different, as determined by Fisher’s LSD test (P < 0.05)
4 Discussion
T. flavus enhances the dissolution of inorganic insoluble P by releasing organic acids such as lactic, fumaric and gluconic and by lowering the medium pH (Scervino et al. 2011). Our results showed a negative correlation between the pH values and the amount of P released when T. flavus was grown in NBRIP medium, confirming the hypothesis that the availability of soluble P may be related to a decrease in pH and the production of organic acids through fungal exudates.
AMF pre-infective development could be modulated by several factors including substances released by roots like flavonoids and strigolactones (Scervino et al. 2005; Steinkellner et al. 2007), abiotic soil status (Vivas et al. 2003) and presence of other microorganisms (Frey-Klett et al. 2007). Previous studies have reported that various concentrations of rhizospheric microbial exudates modulate the development of the pre-infective state of mycorrhizal fungi, indirectly affecting the establishment of the AMF symbiosis (Fracchia et al. 2004; Scervino et al. 2008; Nagahashi and Douds 2011). Our assays showed that T. flavus exudates change the mycelial morphology of G. rosea spores by increasing the length of each branch and increasing the number of hyphal branches. These results suggest that the stimulation of G. rosea hyphal development is not due to direct cell-cell interaction, but to the secretion of soluble chemical substances produced by the metabolism of T. flavus. Moreover, AMF colonization is decreased by high P concentrations in the soil, an effect observed in our experiments. This could be the consequence of a delay in colonization produced by high P levels due to direct effects on hyphal growth from spores and/or reduction in growth of external hyphae and/or the development of entry point hyphae (Guillemin et al. 1955; Schwab et al. 1983; Amijee et al. 1989; Smith and Gianinazzi-Pearson 1990). The increase in the mycorrhizal status with T. flavus inoculation observed in plants could be due to the increase in the development of the pre-infective state of G. rosea, which counteracts the delay by high-P inhibition of the root colonization.
Co-inoculation of mycorrhization-helper bacteria and AMF increased the root colonization probably due to released bacterial effectors that could be plant cell wall-digesting enzymes (Frey-Klett et al. 2007), which would enhance penetration and spreading of AMF within the root tissues (Mosse 1962), or suppressors of plant defense responses (Lehr et al. 2007). It is possible that some of these mechanisms are also present in fungi. Indeed, fungi are well known as extracellular enzyme-producing organisms. Unlike bacteria, direct cell-cell contact between PSF and AMF is not required to produce a beneficial effect on the mycorrhizal colonization (Aspray et al. 2006).
The APA of intraradical mycelia is a useful parameter to assess symbiotic efficiency (Tisserant et al. 1996). APA is induced by high soluble P concentrations and calcium ions (Van Aarle et al. 2002; Tran et al. 2011). In our experiments, the symbiotic efficiency increased in both P conditions. This increase could be explained by the increased soluble P (WEP) and calcium in the soil caused by the P solubilization of T. flavus. Interestingly, APA was also increased without P addition when T. flavus was inoculated. These results suggest that the chemical substances produced by T. flavus metabolism also act as mycorrhizal APA activators, increasing the symbiotic efficiency. However, molecular studies are needed to support this hypothesis. In addition to the level of APA as an efficiency indicator, the increase in P uptake by AMF-colonized plants has been consistently used as an index of symbiotic efficiency. In this work, T. flavus inoculation increased the P uptake of mycorrhizal plants under both P conditions. Although the sole PSF addition led to an increase in TPP, as reported by Mittal et al. (2008), our results showed a greater effect under fungal co-inoculation (AMF + PSF) without P amendment. These results suggest that the inoculation of T. flavus caused a synergic effect on the G. rosea- T. aestivum symbiotic efficiency by increasing both APA and mycorrhizal colonization. The co-inoculation of these microorganisms led the plant-microbe interaction to use the soil P more efficiently through PSF activity and to improve the transport performed by the AMF, resulting in an increase in biomass production.
Under P limitation, plants increase their root system, relocate P from older leaves, and deplete the vacuolar stores of P (Mimura et al. 1996). In soils with available P, most of the P absorbed by the roots is transported through the xylem to younger leaves (Schachtman et al. 1998). In our study, the shoot P:root P ratio was used as an additional tool to estimate AMF symbiotic efficiency. The shoot P:root P ratio increased with T. flavus inoculation, suggesting that this microorganism benefited the plant P status, regardless of the P level. Although P translocation is not the only parameter to estimate the symbiosis efficiency, it should be used along with APA and TPP in future studies to provide a more complete picture.
To our knowledge, this is the first study in vitro and in vivo of the effect of T. flavus on G. rosea development and symbiotic efficiency with T. aestivum. The results obtained contribute to a better understanding of the microbial interaction in the rhizosphere and its effect on plant nutrition and growth.
They reveal a close tripartite relationship between the PSF T. flavus and the AMF G. rosea in symbiosis with T. aestivum plants. Our experiments support the hypothesis that there are microorganisms in the rhizosphere that play multiple roles as P solubilizers, AMF helpers and biocontrol microorganisms. The study of these roles will contribute to a better understanding of the soil ecology and are a challenge for further research.
References
Amijee F, Tinker PB, Stribley DP (1989) The development of endomycorrhizal root systems. A detailed study of effects of soil phosphorus on colonization. New Phytol 11:435–446
Antoun H (2012) Beneficial microorganisms for the sustainable use of phosphates in agriculture. Proc Eng 46:62–67
Arshad M, Frankenberger WT Jr (1998) Plant growth-regulating substances in the rhizosphere: microbial production and functions. Adv Agron 62:46–51
Aspray TJ, Jones E, Whipps JM, Bending GD (2006) Importance of mycorrhization helper bacteria cell density and metabolite localization for the Pinus sylvestris–Lactarius rufussymbiosis. FEMS Microbiol Ecol 56:25–33
Barea JM, Azcón-Aguilar C, Azcón R (1997) Interactions between mycorrhizal fungi and rhizosphere microorganisms within the context of sustainable soil-plant systems. In: Gange AC, Brown VK (eds) Multitrophic interactions in terrestrial systems. Blackwell Science, Cambridge
Brundrett M, Melville L, Peterson L (1994) Practical Methods in Mycorrhiza Research. Mycologue Publications, Waterloo
Fracchia S, Godeas A, Scervino JM, Sampedro I, Ocampo JA, Garcia-Romero I (2003) Interaction between soil yeast Rhodotorula mucilaginosa and the arbuscular mycorrhizal fungi Glomus mosseae and Gigaspora rosea. Soil Biol Biochem 35:701–707
Fracchia S, Sampedro I, Scervino JM, Garcia Romera I, Godeas A, Ocampo JA (2004) Influence of saprobe fungi and its exudates on arbuscular mycorrhizal symbiosis. Symbiosis 36:169–182
Frey-Klett P, Garbaye J, Tarkka M (2007) The mycorrhiza helper bacteria revisisted. New Phytol 176:22–36
Garg S, Bahl GS (2008) Phosphorus availability to maize as influenced by organic manures and fertilizer P associated phosphatase activity in soils. Bioresour Technol 99(13):5773–5777. doi:10.1016/j.biortech.2007.10.063
Gerdemann JW (1955) Relation of a large soil-borne spore to phycomycetous mycorrhizal infections. Mycologia 47:619–632
Guillemin JP, Orozco MO, Gianinazzi-Pearson V, Gianinazzi S (1955) Influence of phosphate fertilization on fungal alkaline phosphatase and succinate dehydrogenase activities in arbuscular mycorrhiza of soybean and pineapple. Agric Ecosyst Environ 53:63–69
Hernández-Martínez M, Cetina-Alcalá VM, González-Chávez MC, Cervantes-Martínez CT (2006) Mycorrhizal Inoculation and its Effect on the Growth of Two Arboreous Leguminosae. TERRA Latinoam 24(1):65–73
Hewitt EJ (1966) Sand and water culture methods used in the study of plant nutrition, vol 22. Second edn. Commonwealth Bureau of Horticulture and Plantation Crops. Commun., East Malling, Maidstone, Kent. Tech.
Khan IA, Ahmad S, Mirza SN, Nizami M, Athar M, Shabbir SM (2007) Growth response of buffel grass (Cenchrus ciliaris) to phosphorus and mycorrhizal inoculation. Agric Conspec Sci 72(2):129–132
Kovar JL, Pierzynski GM (2009) Methods of Phosphorus Analysis for Soils, Sediments, Residuals and Waters. Second edn. Southern Cooperative Series Kansas
Landeweert R, Hoffland E, Finlay RD, Kuyper TW, van Breemen N (2001) Linking plants to rocks- ectomycorrhizal fungi mobilize nutrients from minerals. Trends Ecol Evol 16(5):248–254
Lehr NA, Schrey SD, Bauer R, Hampp R, Tarkka MT (2007) Suppression of plant defense response by a mycorrhiza helper bacterium. New Phytol 174:892–903
Mimura T, Sakano K, Shimmen T (1996) Studies on the distribution, re-translocation and homeostasis of inorganic phosphate in barley leaves. Plant Cell Environ 19:311–320
Mittal V, Singh O, Nayyar H, Kaur J, Tewari R (2008) Stimulatory effect of phosphate-solubilizing fungal strains (Aspergillus awamori and Penicillium citrinum) on the yield of chickpea (Cicer arietinum L. cv. GPF2). Soil Biol Biochem 40(3):718–727. doi:10.1016/j.soilbio.2007.10.008
Mosse B (1962) The establishment of vesicular arbuscular mycorrhiza under aseptic conditions. J Gen Microbiol 27:509–520
Murphy J, Riley JP (1962) A modified single solution method for the determination of phosphate in natural waters. Anal Chim Acta 27:31–36
Nagahashi G, Douds DD (2011) The effects of hydroxyl fatty acids on the hyphal branching of germinated spores of AM fungi. Fungal Biol 115:351–358
Naraghi L, Heydari A, Rezaee S, Razavi M, Afshari-Azad H (2010a) Biological control of Verticillium wilt of greenhouse cucumber by Talaromyces flavus. Phytopathol Mediterr 49:321–329
Naraghi L, Heydari A, Rezaee S, Razavi M, Jahanifar H, Khaledi EM (2010b) Biological Control of Tomato Verticillium Wilt Desease by Talaromyces flavus. J Plant Prot Res 50(3):360–365
Nautiyal CS (1999) An efficient microbiological growth medium for screening phosphate solubilizing microorganisms. FEMS Microbiol Lett 170:265–270
Omar SA (1998) The role of rock-phosphate-solubilizing fungi and vesicular-arbuscular-mycorrhiza (VAM) in growth of wheat plants fertilized with rock phosphate. World J Microbiol Biotechnol 14:211–218
Phillips JM, Hayman DS (1970) Improved procedure for clearing roots and staining parasitic and vesicular-arbuscular fungi for rapid assessment of infection. Trans Br Mycol Soc 55:158–161
Richa G, Khosla B, Sudhakara Reddy M (2007) Impovement of maize plant growth by phosphate solubilizing fungi in rock phosphate amended soils. J Agric Sci 3(4):481–484
Richardson AE (2001) Prospects for using soil microorganisms to improve the acquisition of phosphorus by plants. Aust J Plant Physiol 28:897–906
Richardson AE (2007) Making microorganisms mobilize soil phosphorus. In: Velázquez E, Rodríguez-Barrueco C (eds) First International Meeting on Microbial Phosphate Solubilization, vol 102, Developments in Plant and Soil Sciences. Springer, Netherlands, pp 85–90
Richardson AE, Barea JM, McNeill AM, Prigent-Combaret C (2009) Acquisition of phosphorus and nitrogen in the rhizosphere and plant growth promotion by microorganisms. Plant Soil 321:305–339
Rodríguez A (2004) Hongos del suelo antagonistas de Sclerotinia sclerotiorum. Selección y estudio de potenciales agentes de biocontrol. Universidad de Buenos Aires, Argentina
Rodriguez H, Fraga R (1999) Phosphate solubilizing bacteria and their role in plant growth promotion. Biotechnol Adv 17:319–339
Sadzawka RA, M.A. Carrasco R, R. Demanet F, H. Flores P, R. Grez Z, M.L. Mora G, Neaman A (2007) Métodos de Análisis de Tejidos Vegetales. Instituto de Investigaciones Agropecuarias, 2nd Edition edn., Santiago, Chile
Scervino JM, Ponce MA, Erra-Bassells R, Ocampo JA, Godeas A (2005) Presymbiotic development of Gigaspora and Glomus genera in presence of flavonoids. Mycol Res 109:789–794
Scervino JM, Sampedro I, Ponce MA, Rodriguez MA, Ocampo JA, Godeas A (2008) Rhodotorulic acid enhances root colonization of tomato plants by arbuscular mycorrhizal (AM) fungi due to its stimulatory effect on the pre-symbiotic stages of the AM fungi. Soil Biol Biochem 40:2474–2476
Scervino JM, Mesa MP, Della Mónica I, Recchi M, Sarmiento Moreno N, Godeas A (2010) Soil fungal isolates produce different organic acid patterns involved in phosphate salts solubilization. Biol Fert Soils 46(7):755–763. doi:10.1007/s00374-010-0482-8
Scervino JM, Papinutti VL, Godoy MS, Rodriguez MA, Della Monica I, Recchi M, Pettinari MJ, Godeas AM (2011) Medium pH, carbon and nitrogen concentrations modulate the phosphate solubilization efficiency of Penicillium purpurogenum through organic acid production. J Appl Microbiol 110(5):1215–1223. doi:10.1111/j.1365-2672.2011.04972.x
Schachtman DP, Reid RJ, Ayling SM (1998) Phosphorus Uptake by Plants: From Soil to Cell. Plant Physiol 116:447–453
Schwab SM, Menge JA, Leonard RT (1983) Quantitative and qualitative effects of phosphorus on extracts and exudates of sudangrass roots in relation to vesicular-arbuscular mycorrhiza formation. Plant Physiol 73:761–765
Smith SE, Gianinazzi-Pearson V (1990) Phosphate uptake and vesicular-arbuscular activity in mycorrhizal Allium cepa L.: effect of photon irradiance and phosphate nutrition. Aust J Plant Physiol 17:177–188
Steinkellner S, Lendzemo V, Langer I, Schweiger P, Khaosaad T, Toussaint J-P, Vierheilig H (2007) Flavonoids and strigolactones in root exudates as signals in symbiotic and pathogenic plant-fungus interactions. Molecules 12:1290–1306
Tisserant B, Gianinazzi-Pearson V, Gianinazzi S, Gollotte A (1993) In planta histochemical staining of fungal alkaline phosphatase activity for analysis of efficient arbuscular mycorrhizal infections. Mycol Res 97:245–250
Tisserant B, Gianinazzi S, Gianinazzi-Pearson V (1996) Relationships between lateral root order, arbuscular mycorrhiza development, and the physiological state of the symbiotic fungus in Platanus acerifolia. Can J Bot 74:1947–1955
Tran TT, Hashim SO, Gaber Y, Mamo G, Mattiasson B, Hatti-Kaul R (2011) Thermostable alkaline phytase from Bacillus sp. MD2: effect of divalent metals on activity and stability. J Inorg Biochem 105(7):1000–1007. doi:10.1016/j.jinorgbio.2011.04.005
Van Aarle IM, Rouhier H, Saito M (2002) Phosphatase activities of arbuscular mycorrhizal intraradical and extraradical mycelium, and their relation to phosphorus availability. Mycol Res 106(10):1224–1229. doi:10.1017/s0953756202006470
Vivas A, Marulanda A, Ruiz-Lozano JM, Barea JM, Azcón R (2003) Influence of a Bacillus sp. on physiological activities of two arbuscular mycorrhizal fungi and on plant responses to PEG-induced drought stress. Mycorrhiza 13:249–256
Acknowledgments
This work was supported by the following institutions: Universidad de Buenos Aires (UBA), Consejo Nacional de Investigaciones Científicas y Técnicas (CONICET), and Agencia Nacional de Promoción Científica y Tecnológica (ANCYPT).
Author information
Authors and Affiliations
Corresponding author
Additional information
I. F. Della Mónica and P. J. Stefanoni Rubio contributed equally to this work
Rights and permissions
About this article
Cite this article
Della Mónica, I.F., Stefanoni Rubio, P.J., Cina, R.P. et al. Effects of the phosphate-solubilizing fungus Talaromyces flavus on the development and efficiency of the Gigaspora rosea-Triticum aestivum symbiosis. Symbiosis 64, 25–32 (2014). https://doi.org/10.1007/s13199-014-0299-6
Received:
Accepted:
Published:
Issue Date:
DOI: https://doi.org/10.1007/s13199-014-0299-6