Abstract
Particulate matter concentrations in terms of occupational health, namely inhalable, thoracic, and alveolic, were measured using GRIMM aerosol spectrometer in Basundhara Grajanbahal Area, Sundergarh, India. At mining sites, average inhalable, thoracic, and alveolic particle concentrations were found to be 1.8 to 2, 1.8, and 1.2 to 1.6 times the concentrations at residential sites, respectively. Ratio of thoracic/inhalable, alveolic/inhalable, and alveolic/thoracic varied in the range 73–91%, 42–73%, and 55–80% at residential sites and 69–84%, 37–52%, and 49–63% at mining sites, respectively. From correlation analysis, significant positive correlations among particulate matter concentrations, positive correlation of particulate matter concentrations with relative humidity, and negative correlation with temperature and wind speed were obtained.
Similar content being viewed by others
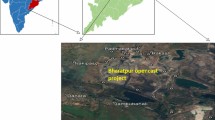
Explore related subjects
Discover the latest articles, news and stories from top researchers in related subjects.Avoid common mistakes on your manuscript.
Introduction
Particulate matter (PM) is defined as the mixture of several materials, such as organic content, metals, acids, ions, and soot (Dubey et al. 2012; Rai 2015; Tecer et al. 2008). It varies in size and shape, surface area, solubility, origin, and chemical, physical, and biological characteristics (Yadav et al. 2015; Ciencewicki and Jaspers 2007; Poschl 2005). In order to assess the different diseases or health hazards, size of a particle is considered as the most significant parameter due to its penetrability into deeper parts of the lungs. Based on aerodynamic diameter, PM is classified as coarse particles (PM of aerodynamic diameter between 2.5 and 10 μm), fine particles (PM of aerodynamic diameter below 2.5 μm), and ultrafine particles (PM of aerodynamic diameter below 0.1 μm). Particles of different sizes which pass through the respiratory system of a human being are categorized as inhalable particles (size range 10–20 μm), thoracic particles (size range 4–10 μm), and alveolic particles (size range < 4 μm). This categorization provides a widespread understanding of association of PM size with human health hazards.
Nowadays, air pollution from opencast mines is of major concern and its occurrence is mainly due to the fugitive emission of PM and gases, including methane, sulfur dioxide, and oxides of nitrogen (Zhengfu et al. 2010). In comparison to underground mines, opencast mines provide higher production due to the large-scale operations and use of high capacity and heavy machines (Kurth et al. 2014; Onder and Yigit 2009). These machines however generate large quantities of PM leading to increased pollution levels in and around opencast mines. In an opencast mine, unit operations, such as top soil removal, drilling, blasting, loading, transport, and unloading, are responsible for generation of PM in diverse size ranges (Chaulya et al. 2003; Onder and Yigit 2009). In order to assess the ambient air quality status, a study was carried out at Padampur OCP, India (Trivedi et al. 2009). The 24-h average of Total Suspended PM (TSPM) concentrations ranged from 294.3 to 1078.1 μg m−3 in industrial, i.e. mining area, and from 390.3 to 654.38 μg m−3 in residential area, respectively. The 24-h average of PM10 concentration was found in the range 120.34–226.4 μg m−3 in industrial and 103.03–130.88 μg m−3 in residential area, respectively (Trivedi et al. 2009). Ambient air quality monitoring for Suspended PM (SPM) and PM10 was carried out at 20 locations in Jharia coal fields (JCF), India, during winter (2008–2009). The 24-h average maximum SPM concentrations ranged 426–711.2 μg m−3. The 24-h average maximum concentrations varied from 214.6 to 342.9 μg m−3 (Jaiprakash et al. 2010). A study in coal mining area of Zonguldak, Turkey, revealed that relative humidity, cloudiness, and low temperature are highly related to the increase of PM episodic events. Average mass concentrations of 29.38, 23.85, and 53.23 μg m−3 for PM2.5, PM2.5–10, and PM10, respectively, were reported (Tecer et al. 2008). A study investigated the environmental exposure of residents in southwest Virginia to PM10 (144.8–250.4 μg m−3) generated by trucks, hauling coal from surface coal mining operations (Aneja et al. 2012). Maximum PM2.5 concentration is observed in the month of January (28 μg m−3) at night and in the month of June (15 μg m−3) during the day in mining area (Asif et al. 2018). A study on potential regional impacts of PM, emitted from one of the world’s largest open-pit coal mines (El Cerrejon), Colombia, revealed that annual average TSP concentrations for the period 2012–2016 were 86 μg m−3 while the annual average PM10 concentration was 34 μg m−3 (Rojano et al. 2018).
Earlier studies on occupational exposure have demonstrated the association of increased level of PM concentration with adverse health effects (Hendryx and Ahern 2008; Finkelman et al. 2002; Pless-Mulloli et al. 2000). For example, presence of black lung disease was identified among coal mine workers in China (Finkelman et al. 2002) and presence of diseases like kidney diseases, cardiovascular problems, and hypertension was confirmed in coal mining areas of Appalachia (Hendryx 2009). Pless-Mulloli et al. (2000) found the evidences of illness, asthma, etc. near the opencast mines in England. These specific health hazards are dependent on the type of material mined and the methodology that is being used in mining. Presence of some common hazardous chemicals in the mined materials includes lead, manganese, cadmium, cobalt, mercury, nickel, selenium, beryllium, iron, asbestos, and crystalline silica (Quintana et al. 2006; Hendryx and Ahern 2008; Donoghue 2004; Aneja et al. 2012; Dubey et al. 2012). Metal composition of dust is responsible for poor health condition as it is able to generate oxygen species that are reactive and may scar lung tissues (Taylor 2002). A study on indoor metallic pollution due to mining activity was carried out in the Bolivian Altiplano. Concentrations of various metallic elements (As, Cd, Cu, Pb, Sb, Sn, and Zn) present in indoor dust were higher at the mining district than at the peripheral district. Pb and Sb concentrations were four times higher in the mining district (Pb ~ 1290 ppm; Sb ~ 490 ppm) than in the peripheral district (Pb ~ 220 ppm; Sb ~ 46 ppm) (Fontúrbel et al. 2011). The associations of the metallic concentrations (As, Cd, Pb, Sb, Sn, Cu, and Zn) with household dust and children hair were studied in two different contamination contexts: a mining district and a suburban non-mining area in the mining city of Oruro, Bolivia. Significant correlations were obtained between metallic elements (As, Cd, Pb, Sb, Sn) in dust and hair in the mining district (Barbieri et al. 2014). A study reported lead, As, and Cd concentrations in aerosols, dust wipes, and surface soils in Broken Hill, Australia (Dong et al. 2015).
This paper presents the findings of occupational exposure to PM around opencast mines. The objectives of this study were to investigate spatio-temporal variability of inhalable, thoracic, and alveolic particle concentrations at both residential and mining sites and influence of meteorological parameters on PM level and to develop constituent relationships among PM concentrations.
Methodology
Description of study area and monitoring locations
The study was conducted at seven residential sites and at ten locations located in the immediate vicinity of two opencast projects (OCPs), namely Basundhara OCP and Kulda OCP, Basundhara Grajanbahal Area, Sundergarh, India (Fig. S1). Kulda OCP produced 10 million tonnes of coal per annum whereas the production of Basundhara OCP was 3 million tonnes per annum (2016–2017). Sampling locations, namely R1, R2, and R3, are situated within immediate vicinity of coal transportation road of Basundhara OCP. Locations namely R4 and R6 are located near Kulda OCP. R5 is located near the road which is being used for coal transportation by both the OCPs whereas R7 is situated away from the mining area. Movement of heavy duty vehicles through unpaved coal transportation road, biomass burning, spontaneous combustion of coal, coal spillage from dumpers, bonfires, primary school playground nearer to monitoring locations, and construction activities for new railway line to facilitate coal dispatch are the major sources of PM at residential sites. Sampling locations M1–M10 are located within 100 m of Kulda OCP. Lease hold area of Kulda OCP is 536 ha. At Kulda OCP, surface RL (RL stands for “Reduced Level”) is 273.41 m, and the pit bottom RL is 189.24 m. The pit size of Kulda OCP is 1600 × 550 m. Operations, such as coal cutting by surface miners, coal loading by pay loaders, and coal stocks, are the major sources of PM at mining sites.
Instrumentation
PM concentrations
PM concentrations in terms of occupational health, namely inhalable (10–20 μm), thoracic (4–10 μm), and alveolic (< 4 μm), were monitored using aerosol spectrometer (Model 1.108). Air sample is drawn through a sampling head perpendicular to the path of a focused light beam produced by a laser diode, and the scattered signal is detected using a high-speed photodiode. Based on the amplitude of the light pulse, the particle is classified in one of the 15 size channels, and the accumulated count in each channel is taken after a fixed duration. From this, the particle number density per cubic meter of sampled air is calculated. An internal flow controller maintains a constant airflow rate of 1.2 l min−1. Time interval for sampling of this instrument ranges from 6 s to 60 min (Grimm 2010). Sampling was carried out daily for 6–8 h during the present study. Data were recorded at 1 min interval and were retrieved through software (Windows Software model 1.177).
Meteorological parameters
In order to assess the impact of local meteorological parameters, such as relative humidity (RH), temperature (T), wind speed (WS), and wind direction, a portable weather station (Spectrum Technologies, Inc., Model WatchDog 2000) (Spectrum 2010) was placed close to Grimm aerosol spectrometer at the sampling site. The resolution of the measurements performed by portable weather station is ± 3 km h−1 for wind speed, ± 4° for wind direction, ± 0.6 °C for temperature, and ± 3% for relative humidity.
Data analysis
Analysis of variance (ANOVA)
The purpose of one-way ANOVA is to explore whether there are significant differences in the mean PM concentrations between sites. This is accomplished by the use of F distribution (Sivaramasndaram and Muthusubramanian 2010). Further, in order to investigate which sites differ, multiple comparisons between all pairs of groups (using Tukey–Kramer procedure) were performed using SPSS Statistics 20.0.
Correlation analysis
The Pearson correlation coefficients (R) among PM concentrations and between PM concentrations and meteorological parameters were estimated with the aid of SPSS package (IBM SPPS Statistics 20).
Results
Meteorological parameters
Relative humidity (RH) typically varied from 18 to 98% (Table S1) during the study period. The high RH at the start of the days was observed between 6 and 8 AM (Fig. S2). The temperature increased from about ~ 10 °C in early morning to ~ 30 °C at mid-day (Fig. S2). Average wind speed varied from 0.05 ± 0.18 to 0.49 ± 0.79 m s−1 (Table S1), and instantaneous wind speed was up to 5 m s−1. For a significant period of time (40%), calm condition (wind speed = 0 m s−1) prevailed, especially during the morning hours, due to less atmospheric turbulence. Wind speed increased as day progressed because of increase of temperature.
Spatial variation of PM concentrations
Frequency distributions
For residential sites, frequency distributions for inhalable, thoracic, and alveolic particle concentrations in the interval of 250, 150, and 100 μg m−3 are depicted in Fig. 1a–c. As indicated by cumulative values, approximately 27% of inhalable data remained in the range 0–250 μg m−3. For thoracic, 15% of data varied in the range 0–150 μg m−3. Similarly, 17% of alveolic data confined to the range 0–100 μg m−3. Figure 2a–c shows frequency distributions in the interval of 500 μg m−3 for inhalable, 250 μg m−3 for thoracic, and 200 μg m−3 for alveolic particle concentrations. Approximately 54% of inhalable data remained in the range 0–500 μg m−3. For thoracic, 26% of measurement data varied in the range 0–250 μg m−3 and 42% of alveolic data confined to the range 0–200 μg m−3.
Descriptive statistics
Average inhalable, thoracic, and alveolic particle mass concentrations were analyzed statistically for both residential and opencast mining areas (Tables 1 and 2). Average concentration of inhalable particles varied in the range 313.38 ± 228.95–823.80 ± 720.56 μg m−3 at residential sites and 560.92 ± 543.51–1712.23 ± 2046.29 μg m−3 within 100 m of Kulda OCP. At residential sites, average thoracic particle concentration varied in the range 233.21 ± 182.14–576.62 ± 463.66 μg m−3 and 409.89 ± 235.25–1022.37 ± 1134.75 μg m−3 at mining sites. Average concentration of alveolic particles varied in the range 128.69 ± 104.90–334.33 ± 126.61 μg m−3 at residential and 211.42 ± 150.06–397.37 ± 105.27 μg m−3 in the immediate vicinity of Kulda OCP. Concentration profiles of different particle sizes are depicted in Fig. 3a–c. Average PM mass concentration ratios (thoracic/inhalable, alveolic/inhalable, and alveolic/thoracic) were calculated, and results are presented in Tables S2–S3. At residential sites, ratio of thoracic/inhalable, alveolic/inhalable, and alveolic/thoracic varied in the range 73–91%, 42–73%, and 55–80%, respectively (Table S2). Ratio of thoracic/inhalable, alveolic/inhalable, and alveolic/thoracic varied in the range 69–84%, 37–52%, and 49–63% at mining sites, respectively (Table S3).
Analysis of variance (ANOVA)
The results of multiple-comparison test (Tukey–Kramer procedure) helped in identifying the pairs of sampling sites for which PM concentrations were significantly different (p < 0.05). An example of post-hoc table of Tukey–Kramer procedure for each site with alveolic particle concentration at residential sites is given in Table S4. The alveolic particle concentration variances between R1 and other five sampling sites (R2, R3, R4, R6, R7) were significant (Table S4). Similarly, significant difference in mean alveolic particle concentrations was also obtained in the case of remaining sampling sites (Table S4).
Temporal variation of PM concentrations
Temporal variation of PM concentrations at both residential and mining sites is depicted in Figs. 4 and 5. Between the start of the monitoring and 8:00, average inhalable, thoracic, and alveolic particle concentrations varied in the range 242.86 ± 89.98–2407.73 ± 2406.59 μg m−3, 218.43 ± 61.36–1458.25 ± 1335.03 μg m−3, and 174.07 ± 45.87–520.36 ± 326.43 μg m−3 during monitoring at mining sites. Between 8:00 and 10:30, the average inhalable (722.90 ± 391.07–2948.53 ± 2586.66), thoracic (465.22 ± 227.88–2044.20 ± 1717.78), and alveolic (234.02 ± 71.24–731.45 ± 523.67) particle concentrations increased. After 10:30, the PM levels started falling until the end of the experiment (inhalable 232.19 ± 56.41–1000.54 ± 1367.63 μg m−3; thoracic 179.36 ± 125.01–560.72 ± 691.01 μg m−3; alveolic 108.36 ± 41.58–297.54 ± 35.59 μg m−3).
Correlation analysis
The Pearson correlation coefficients between occupational particle concentrations and meteorological parameters at each location were estimated with the aid of SPSS package (IBM SPPS Statistics 20.0) (Figs. S3–S4).
Correlation among PM concentrations
Significant positive correlations (p < 0.01) were obtained between thoracic and inhalable, alveolic and inhalable, and alveolic and thoracic at both residential and mining sites. Correlation coefficient, i.e. R = 0.96–0.98 between thoracic and inhalable, R = 0.79–0.91 between alveolic and inhalable, and R = 0.91–0.96 between alveolic and thoracic, was obtained (Figs. S3–S4).
Correlation between PM concentrations and meteorological parameters
Meteorological parameters have significant role for distribution of pollutants (Yadav et al. 2015; Tiwari et al. 2012). Correlation analysis was also carried out to know the degree of association between PM concentrations and meteorological parameters. Significant correlations were obtained between PM concentrations and meteorological parameters at both residential and mining sites. Negative correlation was obtained between temperature and PM concentrations (R = − 0.28 for inhalable; R = ~− 0.40 for thoracic; R = ~− 0.50 for alveolic) and between wind speed and PM concentrations (R = ~− 0.15 for inhalable; R = ~− 0.25 for thoracic; R = ~− 0.40 for alveolic). Positive correlation was obtained between RH and all particle sizes (R = 0.12–0.29 for inhalable; R = 0.15–0.39 for thoracic; and R = 0.25–0.52 for alveolic) (Figs. S3–S4). No significant correlation was found between inhalable particle concentration and temperature at mining sites.
Bivariate regression analysis between PM concentrations
Bivariate linear regression analysis was carried out to establish relationships between PM concentrations. Linear relationships with coefficient of determination, i.e. R2 = 0.93 between thoracic and inhalable, R2 = 0.63 between alveolic and inhalable, and R2 = 0.83 between alveolic and thoracic, were obtained at residential sites (Fig. 6). Comparatively, higher values of R2 = 0.95 between thoracic and inhalable, R2 = 0.83 between alveolic and inhalable, and R2 = 0.93 between alveolic and thoracic were obtained at mining sites (Fig. 7).
Discussion
Spatio-temporal variability of PM concentrations
The large variability of PM concentrations was due to higher concentrations during peak production time and because of complex dispersion phenomena during winter due to inversion conditions, low wind speed, and high relative humidity (Jena and Singh 2017; Tiwari et al. 2014; Srimuruganandam and Nagendra 2010). At mining sites, average inhalable, thoracic, and alveolic particle concentrations were 1.8 to 2, 1.8, and 1.2 to 1.6 times the concentrations at residential sites respectively. Average inhalable, thoracic, and alveolic concentrations in the present study are compared with the average values as reported in previous studies, conducted in different parts of India (Table S5). However, average inhalable particle concentration in the present study is ~ 1.2–3.7 times as high as the average concentration reported at Dhanbad; the highest average thoracic concentration is ~ 1.6–3.3 times the average concentration that was reported from other Indian studies (Table S5). The highest average alveolic concentration in our study is higher than that reported in the study at Dhanbad by a factor of ~ 1.5–2.2.
During the study period, particularly at mining sites, it was observed that PM concentrations attained their highest values between 8:00 and 10:30 (Fig. 5). Although production started before 8:00, PM generated from mining operations could not disperse beyond the pit limit due to high relative humidity (foggy atmosphere), low temperature, and low wind speed (Jena and Singh 2017; Tiwari et al. 2014). Gradual progress of the day resulted in increase of temperature and decrease in relative humidity beyond 8:00 causing good dispersion of PM from the mine. Production level was also at its peak during 8:00–10:30. After 10:30, high wind speed enhanced the dispersion and, thus, PM concentration decreased.
Correlation and regression analysis
During winter, pollutants could not be dispersed as the majority of wind was calm and the region experiences a thick foggy weather with low boundary layer heights (Tiwari et al. 2014). In our study, dispersion of PM generated in the mine to its surroundings up to 8:00 was restricted due to high RH and fog. With gradual increase of temperature, the PM from mining dispersed to its surroundings and, therefore, concentrations were higher at measurement locations between 8:00 and 10:30. High wind speed causes the pollution dispersion by good mixing of pollutants with surrounding air (Tiwari et al. 2012). Therefore, PM concentration decreases with increase of temperature and marginal increase in wind speed, which was observed after 10:30. From bivariate linear regression analysis, higher coefficient of determination (R2) values between thoracic and inhalable, alveolic and inhalable, and alveolic and thoracic were obtained at mining sites in comparison to residential sites. A comparative study of R2 values obtained in this study with the values obtained from earlier studies is given in Table S6.
Conclusion
The present study was carried out to analyze the spatio-temporal variations of occupational PM (inhalable, thoracic, and alveolic) around opencast coal projects. The prominent findings are as follows:
-
(a)
A peak in the distribution of inhalable particle concentration data (approximately 38% of data) remained in the range 250–500 μg m−3, ~ 33% of thoracic particle concentration data were in the range 150–300 μg m−3, and approximately 34% of alveolic particle concentration data remained in the range 100–200 μg m−3 at residential sites.
-
(b)
More than 50% of inhalable particle concentration data remained in the range 0–500 μg m−3 at mining sites. Approximately 41% of thoracic particle concentration data were in the range 250–500 μg m−3 and ~42% of alveolic particle concentration data remained in the range 0–200 μg m−3 at mining sites.
-
(c)
At mining sites, average PM concentrations were ~ 1.2–2 times the concentrations at residential sites.
-
(d)
Overall ratio of thoracic/inhalable, alveolic/inhalable, and alveolic/thoracic varied in the range 69–91%, 37–73%, and 49–80%, respectively.
-
(e)
PM generated from mining operations could not be dispersed beyond the pit limit due to high relative humidity, low temperature, and low wind speed up to 8:00. Higher PM concentrations were observed during 8:00–10:30 (peak production time) as plume of PM started coming out of the pit with gradual increase in temperature and wind speed. After 10:30, PM concentrations started decreasing due to better dispersion (because of rapid increase in wind speed). Therefore, average PM concentrations during 8:00–10:30 were 2–3 times the average concentration for the periods up to 8:00 and after 10:30 at mining sites.
The results obtained from this study are of higher significance for understanding occupational exposure not only to workers who are working away from the source but also for people who are residing in the vicinity of the mine. Implementation of more effective dust control methodologies/technologies, such as water sprinkling at regular intervals through mobile and fixed sprinklers, mist spray arrangement, and application of liquid calcium chloride by the mine management/authority, is required so as to protect the health of the people. This study is mainly confined to occupational health exposure to particulate matter around opencast coal mines in winter. The authors therefore propose more studies on occupational exposure in different mines other than coal under varying weather conditions.
References
Aneja VP, Isherwood A, Morgan P (2012) Characterization of particulate matter (PM10) related to surface coal mining operations in Appalachia. Atmos Environ 54:496–501
Asif Z, Chen Z, Guo J (2018) A study of meteorological effects on PM2.5 concentration in mining area. Atmos Pollut Res. https://doi.org/10.1016/j.apr.2018.01.004
Barbieri E, Fontúrbel FE, Herbas C, Barbieri FL, Gardon J (2014) Indoor metallic pollution and children exposure in a mining city. Sci Total Environ 487:13–19
Ciencewicki J, Jaspers I (2007) Air pollution and respiratory viral infection. Inhal Toxicol 19(14):1135–1146
Chaulya SK, Ahmad M, Singh RS, Bandopadhyay L, Bondyopadhay C, Mondal GC 2003 Validation of two air quality models for Indian mining conditions. Environmental Monitoring and Assessment 82(1):23–43.
Dong C, Taylor MP, Kristensen LJ, Zahran S (2015) Environmental contamination in an Australian mining community and potential influences on early childhood health and behavioural outcomes. Environ Pollut 207:345–356
Donoghue AM (2004) Occupational health hazards in mining: an overview. Occup Med 54(5):283–289
Dubey B, Pal AK, Singh G (2012) Trace metal composition of airborne particulate matter in the coal mining and non-mining areas of Dhanbad Region, Jharkhand, India. Atmos Pollut Res 3(2):238–246
Finkelman RB, Orem W, Castranova V, Tatu CA, Belkin HE, Zheng B, Bates AL (2002) Health impacts of coal and coal use: possible solutions. Int J Coal Geol 50(1):425–443
Fontúrbel FE, Barbieri E, Herbas C, Barbieri FL, Gardon J (2011) Indoor metallic pollution related to mining activity in the Bolivian Altiplano. Environ Pollut 159(10):2870–2875
Grimm (2010) Operational manual of portable laser aerosol spectrometer and dust monitor (model 1.108/1.109). GRIMM Aerosol Technik GmbH & Co. KG, Ainring
Hendryx M (2009) Mortality from heart, respiratory, and kidney disease in coal mining areas of Appalachia. Int Arch Occup Environ Health 82(2):243–249
Hendryx M, Ahern MM (2008) Relations between health indicators and residential proximity to coal mining in West Virginia. Am J Public Health 98(4):669–671
Jaiprakash P, Singh G, Pal AK (2010) Air pollution dispersion modeling performance for mining complex. Environ We Int J Sci Technol 5:205–222
Jena S, Singh G (2017) Human health risk assessment of airborne trace elements in Dhanbad, India. Atmos Pollut Res 8(3):490–502
Kurth LM, McCawley M, Hendryx M, Lusk S (2014) Atmospheric particulate matter size distribution and concentration in West Virginia coal mining and non-mining areas. J Expo Sci Environ Epidemiol 24(4):405–411
Onder M, Yigit E (2009) Assessment of respirable dust exposures in an opencast coal mine. Environ Monit Assess 152(1–4):393–401
Pless-Mulloli T, Howel D, King A, Stone I, Merefield J, Bessell J, Darnell R (2000) Living near opencast coal mining sites and children’s respiratory health. Occup Environ Med 57(3):145–151
Pöschl U (2005) Atmospheric aerosols: composition, transformation, climate and health effects. Angewandte Chemie International Edition 44(46):7520–7540.
Quintana C, Bellefqih S, Laval JY, Guerquin-Kern JL, Wu TD, Avila J, Patino C (2006) Study of the localization of iron, ferritin, and hemosiderin in Alzheimer’s disease hippocampus by analytical microscopy at the subcellular level. J Struct Biol 153(1):42–54
Rai PK (2015) Multifaceted health impacts of particulate matter (PM) and its management: an overview. Environ Skep Crit 4(1):1
Rojano RE, Manzano CA, Toro R, Morales RG, Restrepo G, Leiva MA (2018) Potential local and regional impacts of particulate matter emitted from one of the world’s largest open-pit coal mines. Air Qual Atmos Health:1–10
Spectrum (2010) Operational manual of Watchdog 2000 series Portable Weather Station. Spectrum Technologies, Inc., USA
Srimuruganandam B, Nagendra SMS (2010) Analysis and interpretation of particulate matter—PM10, PM2.5 and PM1 emissions from the heterogeneous traffic near an urban roadway. Atmos Pollut Res 1(3):184–194
Sivaramasundaram K, Muthusubramanian P 2010 A preliminary assessment of PM10 and TSP concentrations in Tuticorin, India. Air Quality, Atmosphere & Health 3(2):95–102.
Taylor DA (2002) Dust in the wind. Environ Health Perspect 110(2):A80–a87
Tecer LH, Süren P, Alagha O, Karaca F, Tuncel G (2008) Effect of meteorological parameters on fine and coarse particulate matter mass concentration in a coal-mining area in Zonguldak, Turkey. J Air Waste Manage Assoc 58(4):543–552
Tiwari S, Bisht DS, Srivastava AK, Pipal AS, Taneja A, Srivastava MK, Attri SD (2014) Variability in atmospheric particulates and meteorological effects on their mass concentrations over Delhi, India. Atmos Res 145:45–56
Tiwari S, Chate DM, Srivastava MK, Safai PD, Srivastava AK, Bisht DS, Padmanabhamurty B (2012) Statistical evaluation of PM10 and distribution of PM1, PM2.5, and PM10 in ambient air due to extreme fireworks episodes (Deepawali festivals) in megacity Delhi. Nat Hazards 61(2):521–531
Trivedi R, Chakraborty MK, Tewary BK (2009) Dust dispersion modeling using fugitive dust model at an opencast coal project of Western Coalfields Limited, India. J Sci Ind Res 68:71–78
Yadav S, Praveen OD, Satsangi PG (2015) The effect of climate and meteorological changes on particulate matter in Pune, India. Environ Monit Assess 187(7):402
Zhengfu BIAN, Inyang HI, Daniels JL, Frank OTTO, Struthers S (2010) Environmental issues from coal mining and their solutions. Int J Min Sci Technol (China) 20(2):215–223
Acknowledgements
The authors are thankful to Prof. Aditya Kumar Patra, Associate Professor, Department of Mining Engineering, Indian Institute of Technology Kharagpur, for providing necessary help during the research work. The valuable suggestions and comments of the anonymous reviewers are highly appreciated as it contributed to extensive improvement of quality of the manuscript.
Author information
Authors and Affiliations
Corresponding author
Electronic supplementary material
ESM 1
(DOCX 12217 kb)
Rights and permissions
About this article
Cite this article
Sahu, S.P., Yadav, M., Rani, N. et al. Assessment of occupational health exposure to particulate matter around opencast coal mines, India: a case study. Arab J Geosci 11, 373 (2018). https://doi.org/10.1007/s12517-018-3631-2
Received:
Accepted:
Published:
DOI: https://doi.org/10.1007/s12517-018-3631-2