Abstract
A combined process, de-algination followed by enzymatic saccharification, was designed to produce alginate and glucose from Saccharina japonica consecutively. The process conditions of de-algination were optimized separately for each stage of acidification and alkaline extraction. Collectively, the de-algination yield was 70.1% under the following optimized conditions: 2.4 wt% of Na2CO3, 70 °C, and 100 min with the acidified S. japonica immersed in a 0.5 wt% H2SO4 solution for 2 h at room temperature. The glucan content in the de-alginated S. japonica increased to 38.0%, which was approximately fivefold higher than that of the raw S. japonica. The enzymatic hydrolysis of the de-alginated S. japonica almost completed in 9 h, affording 5.2 g (96.8% of glucan digestibility) of glucose at a de-alginated S. japonica loading of 14.2 g.
Similar content being viewed by others
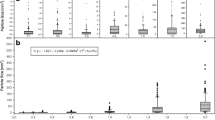
Explore related subjects
Discover the latest articles, news and stories from top researchers in related subjects.Avoid common mistakes on your manuscript.
Introduction
Brown seaweeds typically have a high content of carbohydrates, which are easily degradable, making them a potential substrate for the production of biofuels and chemicals. Most of the studies concerning the bioconversion of seaweed have been related to methane production, whereas the production of liquid fuels such as ethanol has received very little attention probably because alginate, the main constituent of brown seaweed, is a nonfermentable substrate and cannot be utilized directly as a substrate for bioethanol production [1, 2]. Because the cost of fermentation substrates contribute to >60% of the total production costs, it is crucially important, from a process economics perspective, to corroborate inexpensive biomass feedstocks that can be fermented for the production of biofuels and chemicals. While a number of low-cost fermentation substrates have previously been evaluated, macroalgae, particularly the brown seaweeds, are yet to be investigated in terms of their potential for the biological conversion process [3, 4].
Up to now, most of the biofuel and chemical have been produced from sugar and starch, namely called the first-generation biomass. However, the increases in biofuel production from first-generation biomass have encountered the problems such as conflicts with food supplies and land protection [5, 6].
For this reason, lignocellulosic biomass, the second-generation biomass, has become more promising feedstock for the production of fuel and chemical. Although lignocellulosic biomass has numerous advantages such as relatively low cost, great abundance, and sustainable supply over the first-generation biomass, the initial conversion of biomass to sugars is high cost and energy consuming because of their recalcitrance to enzymatic hydrolysis. Therefore, new solutions for the degradation of lignocellulosic biomass are still required to improve the production efficiencies and reduce the cost [7].
As the next generation of biomass, seaweed is being called as third-generation biomass, as this not only can solve the abovementioned problems but also is interesting because of many advantages as feedstock for biofuel and chemical production [8, 9]. Brown seaweeds have critical advantages over the second-generation biomass by having no lignin in their chemical compositions. Furthermore, they require no land, irrigation water, or fertilizers for cultivation. They can be easily cultivated in the sea with the low or almost no external energy and water inputs, and their productivity is higher than that of tropical rainforests, which exhibit the highest productivity among terrestrial plants [10]. Like other cellulosic biomasses, glucans constituting of a component of brown algae, can be enzymatically hydrolyzed into fermentable sugars to be converted into biofuels and chemicals. Establishment of a pretreatment method to make glucan in the brown algae more accessible to enzymes is a key aspect of effective fermentable sugar production. Earlier pretreatment studies have reported that the high sugar contents of several brown seaweeds can be successfully recovered [11]. The alginate, however, takes up a large portion of carbohydrates in brown algae and is a nonfermentable sugar polymer. Brown macroalgae such as Saccharina japonica contain large amounts of carbohydrate composed of alginate, laminaran, fucoidan, and cellulose. Alginate is the most abundant component of brown macroalgae, comprising up to 40% of dry matter. Alginate, polysaccharide, has a linear chain structure made up of α-l-guluronate (G) and β-d-mannuronate(M) as monomeric units arranged in heteropolymeric random poly M and G [12].
Efficient fractionation of brown seaweeds into alginate and glucan can improve the overall efficacy in the algal biorefining industry. For effective valorization of each component in brown algae, fractionation has become the prerequisite and central unit operation. Therefore, appropriate fractionation of brown seaweeds, hereafter referred to as de-algination, can increase the economic feasibility for obtaining the fermentable monosaccharides from brown seaweeds. In addition, alginate is a very high value-added substance, a type of phycocolloids, has stabilizing, emulsifying, and unique gelling properties and can be used to generate numerous products in the food, beverage, paper, biomaterials, and pharmaceutical industries with biotechnological interests [13]. Therefore, to ensure the overall economic affordability of algal bioindustries, fermentable sugar, and alginate should also be conserved without further decomposition.
De-algination process is mainly divided into two stages: The first stage is acidification, which consists of immersing brown seaweed in a dilute acid solution for several hours to convert insoluble alginate salts into alginic acid. In the second stage, alkaline extraction, corresponding to the de-algination, is performed. The acidified brown seaweed is soaked in a Na2CO3 solution, and insoluble alginic acid is converted into soluble sodium alginate, which passes into the aqueous phase. It requires several hours to reach the optimum de-algination yield, depending on the brown seaweed species considered [14]. As shown in Table 1, many researchers have studied on the de-algination process through two-stage acidification and alkaline extractions of brown algae. The previous study suggested that preextraction of alginate has been considered as an essential step because early removal of alginate makes the alginate more readily soluble in an alkaline solution. In the previous study, water was used for de-algination instead of acidifying stage, and the reactive extrusion process was found to be more efficient fractionation method in a large-scale alginate industry [14–19, 21–25].
In our previous study [13], bioethanol production was described using S. japonica as a feedstock by the extremely low acid pretreatment process. According to this study, despite the algae’s higher growth rate and productivity, facilitating the utilization of the carbohydrate in S. japonica such as alginate is essential, because the glucan content in S. japonica is very low than the terrestrial biomass. In this study, we developed the biorefinery concept, using S. japonica as a model brown seed, by the assistance of fractionation technology. A combined process, de-algination followed by enzymatic saccharification, was designed to produce alginate and glucose consecutively. Taking into account the potential application of S. japonica, de-algination conditions were optimized and the yield of glucose by enzymatic hydrolysis was investigated.
Materials and Methods
Feedstock Preparation
S. japonica (Wando, Korea), >provided by the Institute of Cleaner Production Technology (ICPT, Pukyong National University, Busan, Korea), was used as the feedstock for de-algination experiments. S. japonica was air-dried for 24 h at 45 ± 5 °C and milled to an average size of 8 mesh (1.40–2.36 mm) using a blade mill (Blender 7012s, Warning Commercial, CT, USA) and a US standard sieve. The moisture content of the milled S. japonica was 6.3% based on the total oven-dried weight.
Experimental Equipment for Alkaline Extraction
The de-algination experiments were performed in a sealed bomb tubular reactor. Stainless steel tubing with a length, an inside diameter, and a thickness of 20, 1.07, and 0.2 cm was constructed and capped at either end with Swagelok fittings, giving an internal volume of 17.9 cm3. The reactor was loaded with 1 g of the acidified sample, providing a final ratio of 10 mL of alkaline liquor per gram of the oven-dried sample. In order to determine the de-algination conditions, reaction temperature, catalyst concentration, and reaction time were set as independent variables using 0.5–4.0 wt% sodium carbonate solutions at reaction temperature and time in the ranges 40–80 °C and 60–120 min, respectively. The supernatant was collected, quantified, and then freeze-dried for the next experiments.
Determination of the De-Algination Yield
The sodium alginate content of the supernatant was quantified for each sample according to the Kennedy and Bradshaw [26] protocol. A 20% (w/v) polyhexamethylenebiguanidinium chloride (PHMB+Cl) commercial solution (provided by Bayrol France S.A.) was diluted with 1 wt% sodium acetate solution. Ten milliliter of this 0.3 wt% PHMB+Cl solution was stirred with 5 mL of the supernatant sample for 5 min before centrifugation (5000g, 10 min) to separate the precipitate from the supernatant. The absorbance of the supernatant was measured at 235 nm against distilled water as blank by diluting it 100 times. Sodium alginate concentration was obtained from the calibration curve, which was created using commercial Na-alginate (Sigma-Aldrich Co., USA). The de-algination yield was calculated as the ratio of the dry weight of Na-alginate in the liquid phase to the dry weight of alginate in raw S. japonica [20].
Enzymatic Digestibility of De-Alginated S. japonica
Enzymatic hydrolysis was performed according to the NREL standard procedure NREL/TP-510-42629 [27]. Commercial hydrolase, Celluclast (1.5 L), and Novozym 188 (Novozyme A/S Bagsvaerd, Denmark) were purchased from Novozyme Korea Co. Raw and de-alginated S. japonica were hydrolyzed at a hydrolase loading of 15 FPU and 30 FPU per g-glucan for cellulase and 70 pNPGU/g-glucan for β-glucosidase. Raw and de-alginated S. japonica were suspended in 50 mL of 100 mM sodium citrate buffer (pH 4.8), and tetracycline (80 μg/mL) and cycloheximide (60 μg/mL) were supplied to inhibit the microbial growth. Enzymatic digestibility was investigated during 48 h of hydrolysis. The hydrolysates were boiled to deactivate the enzyme and filtered through a membrane filter (0.2-μm nylon membrane filter). Hydrolysis reactions were conducted in triplicate at 50 °C and 120 rpm.
Compositional Analysis of Raw and De-Alginated S. japonica
The glucan; arabinan, mannan, and galactan (AMG); and mannitol contents in the raw and de-alginated S. japonica were analyzed according to the analytical method for cellulosic biomass, NREL/TP-510-42618 [28]. Triplicate samples were hydrolyzed using a two-step acid hydrolysis (72 wt% sulfuric acid, room temperature for 1 h, and then 4 wt% sulfuric acid, 100 °C, for 1 h). The resulting supernatant was filtered (Gelman, 0.2-μm pore size), and the glucose content was determined by HPLC. The conversion factor for the dehydration on polymerization to glucan was 162/180 for glucose.
A HPLC system (Waters Co., Milford, MA, USA) equipped with a RI detector (Waters 2414, Waters, USA) was used for the glucan measurement. A Bio-Rad Aminex HPX-87H (300 × 7.8 mm2) column and cation H+ micro-guard cartridge (30 × 4.6 mm2; Bio-Rad Laboratories Inc., Hercules, CA) were used. The flow rate of the mobile phase (5 mM H2SO4) was 0.5 mL/min, and the temperatures of the column and detector were set at 65 and 50 °C, respectively.
Protein in the S. japonica was estimated using an appropriate nitrogen factor (NF, 6.25), and the nitrogen content of the S. japonica was measured by Kjeldahl methods [29]. The ash content of the samples was determined by complete combustion in a muffle furnace (DMF-12, Romax Co., Korea) equipped with a temperature controller running a temperature ramp program according to the NREL/TP-510-42622 method [30]. The remaining residue in the crucible was taken as the ash content.
Results and Discussion
Chemical Composition of Raw S. japonica
The chemical compositions of S. japonica selected in this study are presented in oven-dried biomass (ODB) weight percentages. Based on the solid analysis of more than 20 replicates, the average percentages and standard deviations of its constituents are listed in Table 2. The initial composition of S. japonica was determined as 7.0 ± 0.5% glucan, <1% the total sum of three oligomeric sugars arabinan, mannan, and galactan, 39.2 ± 0.2% alginate, 14.4 ± 1.1% mannitol, 8.7 ± 0.9% crude protein, 24.1 ± 1.2% ash, and ∼5.6% others on ODB. The analysis of the S. japonica shows that carbohydrate accounted for >60.0% ODB.
Optimization of Acidifying Conditions for Subsequent De-Algination
The acid concentration for the acidified raw S. japonica was determined depending on the alginate yields, obtained by the alkaline extraction of the acidified S. japonica under the following conditions: reaction temperature, 70 °C; reaction time, 100 min; and Na2CO3 concentration, 2.4 wt%. The alginate yield is defined as the amount of alginate in the liquid fraction over the complete alginate content of the raw S. japonica and is represented by percentage. The percentage changes in the alginate yield after the alkaline extraction process for 2 h soaking at room temperature and with various acid (H2SO4) concentrations are shown in Fig. 1. The alginate yield increased proportionally as the acid concentration increased from 51.3% at 0.5 wt% to 77.4% at 4.0 wt% acid concentration. However, the solid remaining after the alkaline extraction decreased rapidly with increasing acid concentration. Specifically, ∼70% of solid remaining was maintained at a low acid concentration (0.5–1.0 wt% of H2SO4), but it decreased to 60.4 and 42.3% at 2.0 and 4.0 wt%, respectively, at high acid concentrations. The decrease in the solid remaining was due to the degradation of solid components by highly acidic circumstances. Thereby, the substantial independent variable was required to optimize the acidifying condition and is defined as the total alginate yield represented by the product of alginate yield and solid remaining.
As shown in Fig. 1, the total alginate yields maintained at ∼60%, and slightly significant differences at various acid concentrations were observed. Only when S. japonica was acidified with 0.5 wt% H2SO4, the total alginate yield was 67.1%. These conditions for acidifying were in agreement with Honya’s result reported more than 20 years ago [15]. Consequently, every S. japonica used for alkaline extraction in this study was acidified with 0.5 wt% H2SO4 at room temperature for 2 h.
Selection the Alkaline Solution for De-Algination
Acidified S. japonica, obtained under the abovementioned conditions, was directly used for subsequent experiments to select appropriate alkaline catalyst increasing the de-algination yield. De-algination experiments were conducted under the following conditions: reaction temperature, 70 °C, and reaction time, 120 min. The changes in the alginate yield after the de-algination of the acidified S. japonica with three alkaline catalysts (NaOH, Na2CO3, and NH4OH) with various concentrations were investigated. As shown in Fig. 2, the alginate yields increased as the concentration of alkaline catalysts increased. With the exception of 0.1% of alkaline catalyst concentration, the de-algination performance of Na2CO3 was much higher than those of other alkaline solutions in all the concentration ranges. The de-algination yield with Na2CO3 maintained at ∼60%, when the catalyst concentration was >2%, whereas the maximum de-algination yield was 41.6 and 32.8% in the case of NaOH and NH4OH, respectively. According to the de-algination yield, when Na2CO3 was used, only slight variations were observed for >2% concentration; therefore, Na2CO3 was deemed a suitable alkaline catalyst for the de-algination of S. japonica.
Optimization of the Reaction Conditions for De-Algination of Acidified S. japonica
The acidified S. japonica obtained under the conditions determined by the acidifying experiments conducted before was directly used for de-algination experiments by varying one of the following three operating parameters: reaction temperature, reaction time, and Na2CO3 concentration. The following experimental sets were investigated: (1) six different Na2CO3 concentrations (0.5, 1.0, 1.5, 2.0, 2.5, and 3.0 wt%), (2) three different reaction times (60, 90, and 120 min), and (3) three different reaction temperatures (40, 60, and 80 °C).
Figure 3 shows the effects of operating conditions on the de-algination yield. At a low reaction temperature (40 °C), the extension of reaction time increased the de-algination yield. However, at a high reaction temperature (80 °C), the de-algination yield adversely decreased with increasing reaction time. Meanwhile, the hasher reaction conditions, i.e., at high Na2CO3 concentration, the higher de-algination yields were observed at a low reaction temperature of 40 °C. At a high reaction temperature of 80 °C, however, de-algination yield decreased with further increase in Na2CO3 concentration. According to this tendency, the optimized de-algination reaction conditions could be found at the experimental sets fixed at a medium reaction temperature of 60 °C. The de-algination yields generally increased with increasing Na2CO3 concentration. In contrast, at Na2CO3 concentration >1.0%, the de-algination yield decreased with increasing reaction time. These results indicate that at a low reaction temperature, the reaction time was a major factor and significantly increased the de-algination yield. Nevertheless, at a high reaction temperature, a shorter reaction time was more capable of improving the de-algination yield (Fig. 3). The maximum de-algination yield of 67.9% was obtained under the following operating conditions: Na2CO3 concentration, 3.0 wt%; reaction time, 60 min; and reaction temperature, 60 °C. Although these results could not be directly comparable to other results in the previous reports, de-algination yield obtained from this work (20–40%) was turned out to be the highest among the referred study using the Laminaria sp. [15, 16, 20]. It was speculated that the acidifying condition in the first step was appropriately selected for making alginate solubilizing more effectively. Under the harsh de-algination conditions, i.e., increased reaction temperature, Na2CO3 concentration, and reaction time, the de-algination yield decreased, attributed to the monomerization of alginate (correctly, Na-alginate) into uronic acid, i.e., β-d-mannuronic acid and α-l-guluronic acid under the harsher reaction conditions. Because the main goal of de-algination is the separation with conserving the alginate in the polymeric form from S. japonica, the degradation of alginate into monomeric form is the major cause not only of the alginate loss but also of the reduction in the selectivity of the alginate solution, requiring an additional separation process cost.
The results from all the experimental sets were taken for the statistical analysis (data are not shown). According to the statistical analysis, the response surface model built from the combined reaction conditions and their corresponding significant coefficient (“probability > F” less than 0.05) for the de-algination yield based on the ANOVA analysis. Through this statistical analysis, it was easy to understand the individual and interaction effects of the reaction conditions (i.e., reaction temperature, reaction time, and Na2CO3 concentration) on the de-algination yield and predict the highest de-algination yield (67.6%) under the optimized reaction conditions (reaction temperature; 69.3 °C reaction time, 120 min; and sodium carbonate concentration, 2.4%). Substantially, 70.1% de-algination yield was obtained under the following optimized reaction conditions: 70 °C, 100 min; and 2.4 wt% Na2CO3.
Enzymatic Digestibility of De-Alginated S. japonica
Figure 4 illustrates the results of the enzymatic hydrolysis experiments carried out with washed and unwashed de-alginated S. japonica, and raw S. japonica was used as the control prepared by suspending in 100 mM sodium citrate buffer (pH 4.8). The data from the mean of triplicate experiments exhibit good reproducibility within the standard deviation of 0.75. The digestibility of the de-alginated S. japonica significantly improved over the control (raw S. japonica). The enzymatic hydrolysis of the de-alginated and washed S. japonica almost completed in 9 h, showing the enzymatic digestibility in the range 97.8–98.9% with increasing hydrolysis time. Meanwhile, very low range of digestibility, from 36.6% at 9 h to 56.5% at 48 h, with de-alginated S. japonica without washing was observed throughout the hydrolysis time. The enhanced digestibility with the de-alginated S. japonica without washing showed only 29.9% over that (43.8%) of the raw S. japonica. This low enhancement of enzymatic digestibility of the de-alginated S. japonica without washing is attributed to the residual alginate hindering the enzyme accessibility to glucan in the de-alginate solid by the adsorption of enzyme onto the alginate, rendering noneffective for glucan hydrolysis.
The other purpose of de-algination was to maximize the glucose production from the de-alginated S. japonica by enzymatic hydrolysis by lowering the hydrolase loadings. Although high glucose yields can be obtained by high hydrolase loadings, 96.8 and 98.7% yields were obtained after 24 h at a loading of 15 FPU and 30 FPU per g-glucan, respectively. The effect of high hydrolase loadings was not significant. The hydrolysis yield and rate (96.8% after 24-h hydrolysis with hydrolase loading of 15 FPU) in this study were higher than those of our previous work [25]. When the de-algination was applied by extremely low acid (<0.1% wt. H2SO4), 83.9% of enzymatic hydrolysis yield was obtained after 48-h hydrolysis. Combined de-alginate process conducted in this study, therefore, could be more effective not only for recovering the alginate but also for enhancing the hydrolysis rate.
Overall Mass Balance of Alginate and Glucose
To estimate the amount of alginate and glucose recovered and lost in both the de-alginated solid residue and the liquid hydrolyzate obtained after the de-algination followed by the enzymatic hydrolysis of S. japonica, a detailed mass balance for each step was established and shown in Fig. 5. The de-algination of 100 g of acidified S. japonica carried out with 2.4 wt% Na2CO3 at 70 °C for 100 min afforded 51.3 g of S. japonica solubilized and 27.7 g of Na-alginate in the liquid phase, corresponding to 70.1% yield of de-algination. After the de-algination, the glucan content in the de-alginated S. japonica increased to 9.1% (without washing) and 38.0% (with washing), approximately fivefold higher than that of the raw S. japonica. A total of 5.2 g of glucose was produced at a loading of 14.2 g of de-alginated S. japonica, with a corresponding glucose yield of 96.8%, indicating that 68.4% of the glucan in the raw S. japonica was hydrolyzed into glucose.
Conclusions
The fractionation process, de-algination and subsequent enzymatic hydrolysis, was developed using S. japonica. Through the de-algination process, the glucan-rich solid residue was obtained and presumed to have increased the hydrolase effectiveness due to the removal of alginate. Moreover, the resulting alginate-rich liquid fraction can be used for various applications. This strongly suggests that a biorefinery concept is required prior to a six-carbon fermentation process and as a valued utilization of alginate, for increasing the overall valorization in the brown algal biorefining industry.
References
Horn, S. J., Aasen, I. M., & Østgaard, K. (2000). Ethanol production from seaweed extract. Journal of Industrial Microbiology and Biotechnology, 25(5), 249–254.
Percival, E., & McDowell, R. H. (1967). Chemistry and enzymology of marine algal polysaccharides (pp. 53–55). London and New York: Academic Press.
Qureshi, N., Saha, B. C., Dien, B., Hector, H. E., & Cotta, M. A. (2010). Production of butanol (a biofuel) from agricultural residues: part I—use of barley straw hydrolysate. Biomass and Bioenergy, 34(4), 559–565.
Huesemann, M. H., Kuo, L. J., Urquhart, L., Gill, G. A., & Roesijadi, G. (2012). Acetone-butanol fermentation of marine macroalgae. Bioresource Technology, 108, 305–309.
Sanchez, O. J., & Cardona, C. A. (2008). Trends in biotechnological production of fuel ethanol from different feedstocks. Bioresource Technology, 99(13), 5270–5295.
Naik, S. N., Goud, V. V., Rout, P. K., & Dalai, A. K. (2010). Production of first and second generation biofules: a comprehensive review. Renewable and Sustainable Energy Reviews, 14(2), 578–597.
Lee, J. Y., Ryu, H. J., & Oh, K. K. (2013). Acid-catalyzed hydrothermal severity on the fractionation of agricultural residues for xylose-rich hydrolyzates. Bioresource Technology, 132, 84–90.
Demirbas, A. (2010). Use of algae as biofuel sources. Energy Conversion and Management, 51(12), 2738–2749.
Goh, C. S., & Lee, K. T. (2010). A visionary and conceptual marcoalgae-based third-generation bioethanol(TGB) biorefinery in Sabah, Malaysia as an underlay for renewable and sustainable development. Renewable and Sustainable Energy Reviews, 14(2), 842–848.
Suzuki, S., Furuya, K., & Takeuchi, I. (2006). Growth and annual production of the brown alga Laminaria japonica (Phaeophyta, Laminariales) introduced into the Uwa Sea in sourthern Japan. Journal of Experimental Marine Biology and Ecology, 339, 15–29.
Kumar, P., Barrett, D., Delwiche, M., & Stroeve, P. (2009). Methods for pretreatment of lignocellulosic biomass for efficient hydrolysis and biofuel production. Industrial and Engineering Chemistry Research, 48(8), 3713–3729.
Kim, D. H., Wang, D., Yun, E. J., Kim, S., Kim, S. R., & Kim, K. H. (2016). Validation of the metabolic pathway of the alginate-derived monomer in Saccharophagus degradans 2-40 T by gas chromatography–mass spectrometry. Process Biochemistry, 51(10), 1374–1379.
Painter, T. J. (1983). Algal polysaccharides. In G. O. Aspinall (Ed.), The polysaccharide (pp. 195–285). New York: Academic Press.
Bertagnolli, C., Uhart, A., Dupin, J. C., Carlos da Silva, M. G., Guibal, E., & Desbrieres, J. (2014). Biosorption of chromium by alginate extraction products from Sargassum filipendula: investigation of adsorption mechanisms using X-ray photoelectron spectroscopy analysis. Bioresource Technology, 164, 264–269.
Honya, M., Kinoshita, T., Ishikawa, M., Mori, H., & Nisizawa, K. (1993). Monthly determination of alginate, M/G ratio, mannitol, and minerals in cultivated Laminaria japonica. Nippon Suisan Gakkaishi, 59(2), 295–299.
Vauchel, P., Leroux, K., Kaas, R., Arhaliass, A., Baron, R., & Legrand, J. (2009). Kinetics modeling of alginate alkaline extraction from Laminaria digitate. Bioresource Technology, 100(3), 1291–1296.
Nishide, E., Anzai, H., & Uchida, N. (1987). A comparative investigation on the water-soluble and the alkali-soluble alginates from various Japanese brown algae. Nippon Suisan Gakkaishi, 53(7), 1215–1219.
Hernández-Carmona, G., McHugh, D. J., & López-Gutiérrez, F. (1999). Pilot plant scale extraction of alginates from Macrocystis pyrifera: studies on extraction conditions and methods of separating the alkaline-insoluble residue. Journal of Applied Phycology, 11(6), 493–502.
Jork, A., Thürmer, F., Cramer, H., Zimmermann, G., Gessner, P., Hämel, K., Hofmann, G., Kuttler, B., Hahn, H. J., Josimovic-Alasevic, O., Fritsch, K. G., & Zimmermann, U. (2000). Biocompatible alginate from freshly collected Laminaria pallida for implantation. Applied Microbiology and Biotechnology, 53(2), 224–229.
Vauchel, P., Kaas, R., Arhaliass, A., Baron, R., & Legrand, J. (2008). A new process for extracting alginates from Laminaria digitata: reactive extrusion. Food and Bioprocess Technology, 1(3), 297–300.
Andriamanantoanina, H., & Rinaudo, M. (2010). Characterization of the alginates from five madagascan brown algae. Carbohydrate Polymers, 82(3), 555–560.
Sanaa, A., Boulila, A., Boussaid, M., & Fadhel, N. B. (2013). Alginic acid and derivatives, new polymers from the endangered Pancratium maritimum L. Industrial Crops and Products, 44, 290–293.
Yuan, Y., & Macquarrie, D. J. (2015). Microwave assisted step-by-step process for the production of fucoidan, alginate sodium, sugars and biochar from Ascophyllum nodosum through a biorefinery concept. Bioresource Technology, 198, 819–827.
Lorbeer, A. J., Lahnstein, J., Bulone, V., Nguyen, T., & Zhang, W. (2015). Multiple-response optimization of the acidic treatment of the brown alga Ecklonia radiata for the sequential extraction of fucoidan and alginate. Bioresource Technology, 197, 302–309.
Lee, J. Y., Li, P., Lee, J., Ryu, H. J., & Oh, K. K. (2013). Ethanol production from Saccharina japonica using an optimized extremely low acid pretreatment followed by simultaneous saccharification and fermentation. Bioresource Technology, 127, 119–125.
Kennedy, J. F., & Bradshaw, I. J. (1987). The rapid quantitative determination of alginates by poly (hexamethylenebiguanidinium chloride) complexation in industrial liquors extracted from brown seaweed. Carbohydrate Polymers, 7(1), 35–50.
Selig, M., Weiss, N., & Ji, Y. (2008). Enzymatic saccharification of lignocellulosic biomass . Golden: National Renewable Energy Laboratory.Report No. TP-510-42629
Sluiter, A., Hames, B., Ruiz, R., Scarlata, C., Sluiter, J., Templeton, & Crocker, D. (2011). Determination of structural carbohydrates and lignin in biomass . Golden: National renewable Energy Laboratory.Report No. NREL/TP-510-42618
Hames, B., Scarlata, C., & Sluiter, A. (2008). Determination of protein content in biomass . Golden: National renewable Energy Laboratory.Report No. NREL/TP-510-42625
Sluiter, A., Hames, B., Ruiz, R., Scarlata, C., Sluiter, J., & Templeton, D. (2008). Determination of ash in biomass . Golden: National renewable Energy Laboratory.Report No. NREL/TP-510-42622
Acknowledgements
The present research was conducted by the research fund of Dankook University in 2014.
Author information
Authors and Affiliations
Corresponding author
Rights and permissions
About this article
Cite this article
Ryu, H.J., Oh, K.K. Combined De-Algination Process as a Fractionation Strategy for Valorization of Brown Macroalga Saccharina japonica . Appl Biochem Biotechnol 182, 238–249 (2017). https://doi.org/10.1007/s12010-016-2323-1
Received:
Accepted:
Published:
Issue Date:
DOI: https://doi.org/10.1007/s12010-016-2323-1