Abstract
Most currently applied systematic methods use post-groundplan character states to reconstruct phylogenies in modern higher Insecta/Arthropoda taxa. But, this approach is unable to separate synapomorphies from frequently occurring homoplasies. Conflicting, unresolved and unrealistic higher-level phylogenies result. The reasons are analyzed. A contrasting “groundplan” method, long used in Vertebrata and found to be superior in resolving higher-level phylogenies, is described. This method, as used for insects, uses a highly diversified morphological organ system (such as limb/wing), identifies its homologues in all subphyla and classes, records the full history of its character transformation series in all lineages from the shared Paleozoic ancestor to modern times, pursues the full homologization of its character states in all modern orders, and verifies these data with evidence from other fields of biology. Only such an extremely broad dataset provides the complex information needed to identify and homologize the groundplan character states in modern orders and other higher taxa in the insect/arthropod fauna. After this is accomplished, the gate to recognizing higher-level synapomorphies is open. Only groundplan-level character states include distinct synapomorphies, since homoplasies are either absent or easily detectable. Examples are given. The interpretations of higher phylogenies and evolutionary processes in Hexapoda, based on the unpredictable and often misleading post-groundplan character states found in extant, Tertiary and Mesozoic fauna, are critically compared with those based on the evolution of organ systems, by using the groundplan method.
Similar content being viewed by others
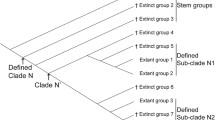
Avoid common mistakes on your manuscript.
Introduction
In the second half of the previous century, the practice of insect systematics was improved by a series of remarkable advances. Hennig (1969, 1981) differentiated types of characters used in phylogenetic evaluation, defined clades and monophyletic groups by deriving their character states from a single ancestor, distinguished them from polyphyletic and paraphyletic clusters, clarified the role of groundplans and full homologization, and introduced cladistic analysis. The Hennigian approach firmly established that the phylogenetically informative character states used in analyses of the modern insect fauna must fulfill three requirements. They must be (i) at the basal (=groundplan) level in all taxa involved (to avoid interference from later uninformative adaptations), (ii) fully homologized, and (iii) recognizable in (at least some) modern insects (to exclude related extinct taxa). Hennig offered convincing arguments that synapomorphies shared by sister groups are part of their groundplan character states, which are present at their divergence. Thus, at the groundplan levels of all extant higher taxa, all synapomorphies are distinctive (not obscured), while homoplasies are rare or absent, and easily recognized. These special conditions offer an invaluable window of opportunity for separating synapomorphies from homoplasies. In post-groundplan subtaxa, convergent adaptations to a similar habitat in unrelated orders frequently generate homoplasies, while most groundplan synapomorphies become unrecognizable or obscured. This leads to perennially unresolved phylogenies (for many examples, see Kristensen 1991, 1995, 1998 and before, and Grimaldi and Engel 2005). Examples are also given later.
Most systematic problems in entomology concern the species and genera (=lower taxa) and families (=intermediate taxa). They are usually successfully resolved by computerized systematic methods in which a matrix of characters and their transformed states is processed in a computer program, which links all analyzed taxa together. In each order, the character states in lower and middle taxa come mainly from the extant insect fauna, and some also from its Tertiary and Mesozoic subtaxa. These are all morphologically close enough to secure a correct intra-ordinal homologization. In addition, most character states in species and genera are naturally at their groundplan level, or close to it. Thus, they fulfill the requirements outlined by Hennig (1969, 1981). As a result, the methods are able to separate groundplan-level synapomorphies from interfering post-groundplan reversals, and are instrumental and successful in objectively reconstructed repeatable phylogenies.
However, in modern higher taxa (suborders, orders, lineages, divisions, classes, and subphyla), the conditions are completely different. The sister groups diverged deep in the Paleozoic and almost all species with unmodified groundplan features perished during the Great end-Permian Extinction. In a few surviving species, the groundplan character states underwent intense adaptations, which modified them at the family, genus, and species level. Hence, in the Mesozoic, Tertiary and Quaternary fauna, the ancient groundplan characters are very often obscured by post-Paleozoic adaptations (usually varied reductions and fusions). Since adaptations to similar environment and function often produced in unrelated taxa convergent transformations, so called “false synapomorphies” abound. These conditions make objective inter-ordinal homologization of many higher character states extremely difficult. As shown now in publications for almost two decades, without homologization down to the Arthropoda level and an ability to identify, in sister groups, the synapomorphies at their groundplan level the relationships of modern higher taxa are quite impossible to resolve. There are a number of well-known “living fossils” which have helped to show relationships of higher-level clades. Examples are Machilus in Archaeognatha, Mastotermes in Isoptera, Notiothauma in Mecoptera, and Agathiphaga in Lepidoptera. However, the main bulk of evolutionary data is in reconstructing, and then comparing, the groundplans of all modern higher taxa. This is based mainly on a study of character transformation series in all insect lineages, from Paleozoic to modern times, and on complementary data from genetics, ontogeny, etc. If this approach sounds like an enormous task, it truly is; but, there may not be other option.
Much of the contemporary argument about higher systematics seems to miss a set of simple facts. Order-level cladogenetic events were ancient in their time of occurrence. It is only ancient character states, which will document these events. Of course, modern faunas retain little evidence of the origins of these clades, which makes modern species quite inadequate as a sole source of phylogenetic information. As a fact documented by Hennig (1969, 1981) only the groundplan characters include synapomorphies in their original, clearly recognizable and unobscured state. Instead, the currently used systematic methods habitually limit their data intake to the derived, post-groundplan character states, without paying enough (or most frequently any) attention to their taxonomic level and perfect homologization. This omission mixes into higher phylogenies too many superficially similar but unrelated character states, which antagonize the fundamental systematic requirement for fully homologized character states at their groundplan level. Subjectively reconstructed, conflicting phylogenies result and are then endlessly argued, with no solution in sight.
As demonstrated here (Figs. 1–21), the groundplan characters in the morphological organ systems of modern insect higher taxa can be identified by recognizing their origin and attributes, finding their homologues in other Arthropoda and then tracing their evolution from the Paleozoic insect ancestor to modern insect species. This “groundplan method” has been successfully used in vertebrates and some invertebrates for most of the past century, and it is equally applicable also in arthropods. However, quite unfortunately, the groundplan states of the extant higher taxa often are not at all evident or even visible, in most living insect species! Instead, they are retained in only a few species in basal families and must be identified by research.
Arthropod polyramous limb groundplan. Segmented limb-derived appendages on the head, thorax, and abdomen are serially homologous in all body segments and can be derived from a single polyramous limb model through reductions, fusions, subsegmentations and other modifications. The outer and inner rami (branches), the exites and endites, respectively, are along the shaft of each limb. In Arthropoda, the epicoxa always forms a pleuron serving as an articulation site. In Insecta the epicoxal pleuron in the head is fused to cranial terga; in the thorax it is articulated to terga (in Archaeognatha), articulated and fragmented into wing pteralia (in Pterygota), or fused to terga or reduced; in the abdomen it is fused to terga as a narrow sidelobe, with or later without, a suture, and alone or combined with the wing. In the head all limb appendages of the mouthparts articulate with the subcoxa; in the thorax the subcoxa is cylindrical or flattened into the subcoxal pleuron; in the abdomen the subcoxa, coxa and trochanter form the abdominal pleuron. Exites (outer rami) are lobes which evaginate from the membrane between the podites, then often shift into the proximal podite and are enclosed in it; plesiomorphic podites are conical, annulated, articulated, mobile lobes inserted by muscles from the proximal and distal podite; endites (inner rami) are lobes similar to exites but they evaginate and are musculated from within only one podite. Dotted rami are those reduced in insects. Reduction and fusion of rami to podites is very frequent. Limbs in all Arthropoda were homologized by E. L. Smith; limbs in Paleozoic insects were researched by JKP. Original, JKP. Kristensen (1998) and before interprets the insect limb to have only 6–7 podites; the exites and endites as secondary lobes; the origin of mouthparts, wings, pleura and genitalia as uncertain
Differences between generalized Paleozoic and modern pterygote nymphs. Fossils show the limb organ to be present on all body segments and serially homologous (=homonomous) in podites and rami (exites, endites). Up to 14 pairs of limbs occur; all bear climbing tarsi with once subsegmented ET and curved double claws. Abdominal ventral vesicles (formed from endites) are serially homologous with genitalia. All wings and winglets (=flattened epicoxal exites) are originally serial, fully articulated and mobile. Epicoxal pleuron is fused to cranium in the head, articulated to tergum and fragmented into wing pteralia in the thorax, and fused (with a suture) to terga in the abdomen (abdominal winglets are originally articulated, later fused, or reduced). In most modern nymphs the winglets plus their articulation were secondarily fused to terga and resemble lateral tergal extensions; limbs are much more dissimilar and appear to be not serially homologous. Schematic, after Kukalová-Peck (1991), updated. Kristensen (1998) and before interprets wings as autapomorphic in Pterygota and there is no appendage homologue in other Arthropoda; limb-derived appendages in mouthparts, thorax and abdomen are uniquely formed, not derived from a monophyletic all-arthropod limb ancestor; chewing lobes in mouthparts, vesicles, gonapophyses, penes and valvulae are of uncertain origin, not derived from all-arthropod endites and not serially homologous. If these were true, these profound morphological differences would remove insects from Arthropoda
Differences between Paleozoic and modern thoracic limbs in winged insects. (1) Insect (and arthropod) limbs show a maximum of 11 muscled, articulated segments. First limb podite is flattened into epipleuron (ECX), articulated to tergum and enclosing the flattened epicoxal wing exite. This is followed by a subcoxal pleuron (SCX) supporting the pleural wall. Free limb is Z-shaped and contains nine cylindrical articulated podites starting with the coxa. Strongly modified podites occur at bends and include: the patella (fused to tibia with a suture), basitarsus (strongly slanted, muscled), eutarsus (once subdivided in all telopodites), and pretarsus with double claws (in all telopodites). Insects retained a maximum of four annulated exites on proximal podites. (2) Modern limbs have obscured the podites near their bends and mostly show only 6–7 segments: the epipleuron plus wing may look like a tergal extension with a fragmented base; trochanter (TR), prefemur (PFE), and patella (PAT) are fused to other podites; basitarsus (BT) and subsegmented eutarsus (ET) may look like a single “tarsal” segment (TA) subdivided into several similar articles; outer rami are mostly lost. This impoverished leg in Hexapoda (Atelocerata) thus appears to be “non-homologous” with the limb of other Arthropoda. Schematic. Original JKP, based on material published in 1983, 1991, 1992, and 1998. Kristensen (1998) and before interprets the insect thoracic leg to lack PFE, PAT, BT, and to bears no exites. This is an error because articulated TR and PFE are retained in extant Odonata; PAT is shown as separated by a deep suture in living Paleoptera; BT as cilindrical and muscled (=definition of a podite) occurs in most Pterygota; all-arthropod endites form working parts of mouthparts and genitalia; and up to four exites are retained in modern Archaeognatha
Comparison of maxilla and leg in the most primitive Pterygota (†Diaphanopterodea). Higher-level character states in maxilla: epipleuron fused to cranium (Hexapoda groundplan); subcoxa articulated at both ends (Arthropoda groundplan); coxa (CX) + trochanter (TR) fused (an autapomorphy of Dicondylia); coxendite + trochendite fused (an autapomorphy of Paleoptera); patella (PAT) fully articulated (Arthropoda groundplan); eutarsus (ET) subdivided into two articles and pretarsus (PT) bearing double claws (two autapomorphies of Hexapoda). In the thoracic leg: all subcoxae (SCX) flattened as pleuron (an autapomorphy of Dicondylia); prefemur (PFE) fused to femur (FE) (probably an autapomorphy of †Diaphanopterodea); patella (PAT) fused to tibia (TI) with a suture (an autapomorphy of Hexapoda and a plesiomorphy of Paleoptera; suture lost, an autapomorphy of Neoptera); eutarsus (ET) once subdivided, occurring in all unreduced palps and fossil abdominal leglets, and also in thoracic legs of basal fossil orders (an autapomorphy of Hexapoda). Schematic. Original JKP based on material published in 1992, 1998. Kristensen (1998) and before interprets podites ECX, PFE, PAT, BT to be absent in insect thoracic legs. This is an error: they are obscured in most but distinct in some extant insects: see evidence here. Also wrong is that plesiomorphic Parainsecta do not have a flexible tarsus, and hence the tarsal joints are neoformations added in Insecta. But, tarsal fusions are always derived (muscled original podites never occur de novo, and their loss is irreversible, see text); arthropods living on loose substrates routinely develop straight tarsi by fusing tarsomeres together, and with the tibia, see evidence of this process in †Monura and Archaeognatha, Figs. 7 and 8, here
The most primitive maxilla, but not leg, in modern Neoptera (Hymenoptera: Pleroneura). Plesiomorphic maxilla: epipleuron fused to cranium; podites and claws exactly as in Paleozoic Pterygota (Fig. 4); coxal and trochanteral endites remain separate (a plesiomorphy of Arthropoda and Neoptera). Derived leg: patella fused without a suture (an autapomorphy of Neoptera); eutarsus (ET) subdivided into four articles (an autapomorphy of Hymenoptera, tarsal adaptation for better climbing, occurring in Pterygota only in thoracic legs). After JKP (1998), altered. Kristensen (1998) and before thought that additional limb segments and double claws in head palps are absent in modern insects, which invalidates their alleged occurrence in fossils. This is a simple error and oversight: All 11 articulated podites of the Precambrian arthropod groundplan limb (Fig. 1) were retained in modern Archaeognatha; double claws (of the Hexapoda groundplan) are retained in the maxillary palps, of modern sawflies and of Raphidioptera—in their “walking pupa” (E. L. Smith, personal communication)
Generalized insect head scheme with clues from Monocondylia: †Monura. Acron is a frontal flap with sensory organs, ocelli and eyes, but is not a segment. It bears up to four sulci. All cranial terga (TE) are horseshoe-shaped and surrounding the acron (which shifted upwards and became enclosed), their ends fused with epicoxal pleura (E). Tergum 1 + epipleuron 1 (TE + E1) and coxopodites 1 meeting anteriorly under acron and laterally fused to each other; coxopodites 1 forming clypeo-labrum. TE + E2 bears antenna (not illustrated) articulated with muscled subcoxa and coxa. TE + E3 dorsal and ventral to eye, invaginates and bears hypopharynx (homologous to antennulae (antennae 2) in Crustacea and to chelicerae in Chelicerata). Triangular lobe distal from eye possibly represents Tömösvary organ (see Fig. 7). All head appendages articulate to TE + E with their subcoxae. Limb parts in hypopharynx are not yet fully homologized. Mandibular palp reduced and lost in Hexapoda. Serial homology in coxopodites, telopodites and endites is very distinct in fossils, but much less visible or obscured in most (not all!) extant insects. All Arthropoda (including Insecta) always eat with their coxopodite (=3 podites plus 2 endites), never with the coxa alone! Head limbs in arthropods and modern insects were homologized by E. L. Smith; ancestral features found in †Monura and Paleozoic Dicondylia, were included by Kukalová-Peck (1987, 1991, 1992, 1998). Combined figure by JKP. Kristensen (1998) interpreted the insect mandible to be a single piece (not a coxopodite); the so-called endites in the head as secondary, non-serial lobes; the maxilla as not more primitive than the thoracic leg. But, these are errors since sutures between podites left behind after their fusion are retained in mandibular coxopodites of some trilobites, and are quite distinctive in living Archaeognatha (Figs. 11, 12); the thoracic leg, Z-shaped, suspended from two flattened pleura and bearing a wing as well as climbing tarsus, is adapted to lift the insect body up and to serve equally well in walking, running over uneven substrates, climbing, jumping, holding, scratching, and flying, and is a marvel of multiple functionality even among arthropod appendages
The most primitive insect order †Monura (Carboniferous—Permian). This is the Paleozoic sister group of modern Archaeognatha (Monocondylia). Head: acron and all terga + epipleura are delimited by sutures; eyes semi-circular, wedged deeply into TE + E3 at a triangular Tömösvary organ; TE + E3 under eye crescent-shaped, ventrally invaginated; TE + E2 meets TE + E4 ventrally from TE + E3; clypeolabrum (shown here as falsely overlying antenna and maxillary palp) with laterally fused true limb segments, ending with fused annulated trochanteral endites; antennae with five large basal podites (possibly all muscled?) followed by a narrower flagellum. Thorax: large, darkly pigmented epicoxal pleura articulated to terga and to subcoxae. Subcoxa (SCX) flattened into pleuron only in prothorax, but subcoxa remained cylindrical in meso- and metathorax; basitarsus (BT) slanted, anteriorly disconnected, scale-like; ET1 and ET2 flexed on contact, close to their ancestral position adapted to climbing. Claws missing, (they very probably broke off as the matrix split). Dasyleptus brongniarti Sharov, 1957. After Kukalová-Peck 1998, altered. Kristensen (1998) and before concluded that the origin of thoracic pleura is uncertain; tarsus originated from a single, straight podite, which became later subdivided; existence of ECX pleuron was not proved. However, both in †Monura and living Archaeognatha, ECX pleuron forms a large plate articulated dorsally to tergum and ventrally to SCX; SCX is a cylindrical podite in meso- and metathorax but a flat pleural plate in prothorax—what other, or stronger, proof is needed that ECX exists and that the thoracic pleuron in insects is formed by a flattened subcoxal podite? In †Monura ET1 is at an angle to ET2, while in their sister group, the modern Archaeognatha, ET1 + ET2 are straightened up and fused: (see Fig. 8) the only logical conclusion available is that the modern tarsus is derived. A similar secondary adaptation to moving on unconsolidated substrates occurred independently and in parallel in Parainsecta and other arthropods
The most primitive modern insect order Archaeognatha (Devonian-Recent). (1) Maxilla: Epicoxal pleuron fused to cranium. Coxopodite with three segments and two endites, all fully articulated (a plesiomorphy, as in the Arthropoda groundplan). Coxopodite articulates to cranium with conical subcoxa (SCX). Telopodite (palp) 7-segmented, with once subdivided eutarsus (ET); exites on PFE and FE shifted upward and fused to their podites. Pretarsal claws reduced (an autapomorphy of Monocondylia). (2) Prothoracic limb: Epicoxal pleuron entire, articulated at both ends (a plesiomorphy, as in the Arthropoda groundplan); subcoxa (SCX) flattened into pleuron; trochanter & prefemur (TR + PFE), and patella + tibia (PAT + TI) fused; basitarsus (BT) slanted, anteriorly disconnected (four autapomorphies of Monocondylia); ET1 + ET2 fused and perpendicularly oriented (an autapomorphy of Archaeognatha). (3) Mesothoracic limb: Epicoxal pleuron desclerotized; subcoxa (SCX) cylindrical, articulated at both ends (a plesiomorphy, as in the Arthropoda groundplan); coxal exite annulated (a plesiomorphy), shifted upward into coxa (CX), enclosed, immobilized, and used as tactile appendage (three autapomorphies of Archaeognatha). After Kukalová-Peck (1998), altered. Kristensen (1998) and before maintained that coxal lobe is not an exite because it is positioned in the middle of coxa. This is an error because some arthropod exites shift upward (as in Fig. 12 here), from the membrane into the proximal podite, into which they eventually become enclosed (E. L. Smith, communication during cooperation with JKP)
Archaeognatha. Epicoxal pleuron and subcoxal pleuron in prothoracic leg. Both pleura are fully articulated and positioned exactly as in †Monura (Fig. 7) and Pterygota (Figs. 15–19), but in the latter, ECX was fragmented around muscle attachments into articular sclerites. SEM photograph, JKP. Kristensen (1998) and before stated that the occurrence of the epicoxal and subcoxal pleuron in Insecta is disproved by their absence in modern insects. This is an error and oversight because as shown here they are present in insects, either entire or fragmented in sclerites, at exactly the same serially homologous level in all tagmatic segments
Evolution of the endites in insect maxilla. (1) Monocondylia: In Archaeognatha, subcoxa (SCX), coxa (CX) and trochanter (TR) are fully articulated; coxal endite (lacinia) and trochanteral endite (galea) are individually articulated to their podites. (2–5) Dicondylia: CX + TR are fused (an autapomorphy); (2–4) Paleoptera: CX + TR endites are also fused, into lacinio-galea (an autapomorphy). (5) Neoptera: CX + TR endites remain individually articulated, as in Archeaognatha (a plesiomorphy at Arthropoda groundplan level, Fig. 1). Hence, the fusion of maxillary endites in Paleoptera is unique and monophyletic. After Kukalová-Peck (1998), altered. Kristensen (1998) and before believed that the maxillary lobes are not informative phylogenetically. However, like all other ancient morphological features, endites become informative only after they are correctly identified and fully homologized at the Arthropoda level! Then, their unique fusion in all Paleoptera stands out as a strong synapomorphy, because the same endites are separate in Neoptera and other Arthropoda
Evolution of the anterior articulation in insect mandibles. (1) Monocondylia: In Archaeognatha, the mandible is narrow, slanted, with anterior socket absent, engaged mainly in milling movement, and rarely pressing forward to mimic condyle; SCX, CX, TR and two endites of mandibular coxopodite are separated by sutures, and CX is subdivided. (2–6) Dicondylia: Mandible is without sutures, broadly triangular, extended anteriorly to articulate by a socket with a projection on clypeo-tentorium. (2) Zygentoma (silverfish): mandible bears two sockets, outer and inner, articulated to a yoke. (3–6) Pterygota: mandible bears one (outer) socket only; early articulation (as in modern mayfly nymphs) was secured by voluntarily pressing the mandible forward. (3–4) †Palaeodictyoptera (with rostrum, adapted to suck the contents of fructifications), and Ephemeroptera (aquatic nymphs eating soft food) bear a mandible with an ancestral elongate socket (=slider) sliding against a clypeo-labral track. (5, 6) Odonatoptera (predators chewing insect exoskeletons) and Neoptera (chewing tough terrestrial vegetation) bear a mandible with a shorter socket, tightly fitting around a ball on clypeo-tentorium; such mandibles can open laterally on two condyles like a door on hinges for a strong shearing action (convergent adaptation for shearing, a homoplasy). Original, JKP. Kristensen (1998) and before thought that the so-called slider occurs only in Ephemeroptera as an autapomorphy, separating them from all another Pterygota. This is a simple oversight: as has been long known, it is present also in other Paleoptera: Palaeodictyopterida, representing over 50% of Paleozoic global entomofauna. Paleoptera also differs from Neoptera in 65 wing-related characters (Haas and JKP 2001)
Evidence for the 3-segmented mandible and polyramy. In modern Archaeognatha: (1) mandible (coxopodite), showing three limb podites (SCX, CX, TR) and two endites (mola, incisor) still separated by sutures. (2) Maxilla, the prefemoral outer ramus (exite) is shifted upward into prefemur, fused and enclosed. (3) Maxilla, the femoral exite still residing in its original, shallow membranous embayment at the base of femur (FE), secondarily immobilized. (4) In Pterygota: Paleoptera: †Palaeodictyopterida (Early Permian): thoracic leg with prefemoral outer ramus (exite) still retaining plesiomorphic annulation. Extant Insecta and Crustacea bear up to four outer rami, often secondarily immobilized. Exceptions are wings (in Insecta), and swimming “legs” and aquatic gills (in Crustacea). By shared occurrence and monophyly, Hexapoda and Crustacea are both ancestrally polyramous. Presenting these taxa as “uniramous” and “biramous” shows an oversight (or ignorance) of some 20 years of scientific contributions. (Photographs, by JKP 1998). Kristensen (1998) and before interpreted the mandible as one piece, which is a simple error: mandibular sutures, replacing articulation after limb podites become fused, show all mandibles in arthropods as homologous and as coxopodites. He also thought that limb exites in fossils do not exist. This is also a simple oversight: they do exist and their photographs were published by JKP, in 1983. Similarly he thought that coxal lobe in modern jumping bristletails is too high to qualify as an exite. This is a simple error: the arthropod exite starts from membrane (3), but often shifts upward (2), and the upper podite may close underneath it (Fig. 8.3)
The most primitive dicondylous insect order †Cercopoda (Carboniferous). This order was adapted to a cryptic lifestyle, when Dicondylia still bore abdominal rope muscles and used them in vertical jumps (a synapomorphy of Mandibulata, expressed also in Crustacea and Monocondylia). †Cercopoda bore 15 pairs of relatively long limbs (including limb-like cercopods), with all except palps equipped by double claws (Hexapoda groundplan); thoracic and abdominal epipleura plus protowings were fused with sutures to terga as sidelobes. (2) †Cercopoda are all fossilized in an arched position, which is evidence for post-mortem contraction of the rope muscles. (3) †Cercopoda bear a robust sclerotized ovipositor with the gonangulum (SCX fused to acrotergites), subcoxal pleura present on all thoracic segments, and a broad, triangular, dicondylous mandible (three synapomorphies of Dicondylia = Pterygota + Zygentoma). (1, 3) A cumulative reconstruction: after Kukalová-Peck (1987, 1998), updated. (2) Undescribed species, Mazon Creek, Illinois. Original photograph, JKP
Paleozoic nymphs with articulated, mobile wings. A young nymph of Palaeodictyoptera (Late Carboniferous, Siberia) from a basal family, showing a small fore winglet fully articulated as in the adults. Note that in some Carboniferous young nymphs the secondary fusion between the winglets and the terga has already started. After Kukalová-Peck (1983), revised. Kristensen (1998) thought that insect nymphs only bear immobile wing pads, and that the subimago with mobile wings is unique to Ephemeroptera and separates them phylogenetically from Odonatoptera + Neoptera. This is a simple oversight. The fact that most Paleozoic pterygote orders bore plesiomorphic nymphs with articulated, mobile wings and had several subimaginal instars has been documented by different authors in a dozen papers. Such winglets are found fossilized separate from the bodies (by the hundreds in Permian mayflies from Elmo, Kansas), showing that the wing base was articulated at a zone of structural weakness, not a zone of strength and fusion to the tergum
Pterygota, limb homology in the abdomen. In most Paleozoic insects, the abdominal epicoxal pleuron is fused to the tergum with a deep suture as a narrow sidelobe. Articulated epicoxal winglets (flattened exites mobilized by the same coxal muscles as adult wings) were retained only in the (early-secondarily) aquatic pterygote nymphs of Ephemeroptera (in other Pterygota they probably fused with and were absorbed by ECX). Subcoxa, coxa, and trochanter flattened into the pleura and reinforced the pleural membrane. Leg shaft (telopodite, PFE to PT) bears subdivided ET and double claws (Hexapoda groundplan). Coxal endites (vesicles or male penes) and trochanteral endites (vesicles, ovipositor valves and male gonapophyses) flank the triangular sternum. In male genitalia, the outer trochanteral endites become superimposed over the inner coxal endites. In females, coxal endites are reduced. Vesicles are present in silverfish and present (modified) in Neoptera (a symplesiomorphy), and are reduced in Paleoptera (an autapomorphy). Original JKP. Kristensen (1998) and before thought that vesicles are secondary lobes, and not serial with the endites in mouthparts and genitalia. (But, compare Figs. 2, 6, 13, 15, 20, 21)
Carboniferous dragonfly with three pairs of wings. Prothoracic winglets in the most primitive dragonfly order †Geroptera from Argentina retained the ancestral protowing veinal system as the shared groundplan venation of all Pterygota. This is dissimilar to the typically odonatan flying wings, but very similar to prothoracic winglets present in the (only distantly related) Carboniferous order Palaeodictyoptera (see Fig. 17.1). Never used in aerial forward flapping flight, the prothoracic winglets retained the original venation of the pre-flight ancestral protowings, which originally may have formed a continuous homonomous series on all segments of the thorax and abdomen. After JKP in: Wootton and Kukalová-Peck (2000)
Prothoracic wings and ancestral protowing. (1) Prothoracic wing of †Palaeodictyoptera (Late Carboniferous, France). (2) Prothoracic wing of Odonatoptera: †Geroptera (Late Carboniferous, Argentina). (3) Ancestral protowing model (1983) based on long term research of wing transformations in all Pterygote lineages, from the Paleozoic to modern times. All three sources independently show eight veins, each vein composed of two basally separate and dichotomously branching veinal sectors. This ancestral wing model indicates that all veinal stems, fusions and braces near the wing base in higher taxa are derived. When added at the groundplan level in higher taxa (i.e., no longer subject to reversals) these fusions and braces have good potential to offer synapomorphies. After Kukalová-Peck and Lawrence (2004). Kristensen (1998) and before thought that wings do not offer reliable synapomorphies for higher-level phylogenies; but just the opposite is true: Neoptera and Paleoptera differ in a remarkable 65 (!!) wing characters (Haas and JKP 2001); and Neoptera divide into two groups of sister lineages with different types of wing articulation, veinal fusions and braces, configuration of folds and flexion lines in fore- and hind wing anojugal lobes, etc. These synapomorphies habitually cascade from divisions to lineages and down to orders, while offering several synapomorphies in every taxonomic level in their descent (JKP and Lawrence 2004)
Paleozoic wing articulation close to pterygote protowing model. (1) Basal pterygote order †Diaphanopterodea, bearing up to 14 pairs of serially homologous limb appendages with a once subdivided eutarsus and also often claws (Hexapoda groundplan). (2) Their wing articulation was arranged in eight horizontal rows of fully articulated sclerites, aligned with eight wing veins (marked by colors), and in three perpendicular columns (marked with different patterns). (3) Diagram of the same pattern, showing eight rows and three columns of sclerites: proxalar (PR), axalar (AX), and fulcalar (F). Fulcalar column articulates with basivenalia (B) (=sclerotized veinal bases of paired veinal sectors). Fusions between precostal and costal row of sclerites are derived (an autapomorphy of the lineage Palaeodictyopterida). However, the remaining overall pattern is identical with the protowing model (1983) based by this author on all unfused articular sclerites recorded in all lineages of Pterygota and presented in one model (compare to Fig. 19). (1, 2) After Kukalová-Peck (1983), revised; (3) original JKP. Kristensen (1998) and before thought that the composition of wing veins from two veinal sectors cannot be proved, especially since precosta does not have two sectors. However, two sectors of Sc, R, M, Cu, A, J start independently from basivenale in many fossils and in some modern insects, as repeatedly published by this authors and co-authors. The precosta starting as two sectors from precostal basivenale basivenale was discovered only in 2006, in modern mayflies by T. Soldán, in JKP laboratory in Ottawa
Neoptera, complex wing articulation derived from pterygote protowing. (1) In Neoptera, the wing articulation contains three irregular clusters of protowing sclerites: first axillary (1Ax), second axillary (2Ax), and third axillary (3Ax); small circles denote muscle insertions. Single sclerites are articulated, hinged, or fused with (or later without) sutures. (2) Diagram showing how irregular neopterous clusters are composed of sclerites from different rows and columns. Compare Fig. 18.3. (1) After Kukalová-Peck (1991, 1998) and JKP in Haas and Kukalova-Peck (2001). (2) Original JKP. Kristensen (1998) and before thought that wing articulation is of little use in phylogenetic considerations. But, actually, a fully homologized articulation evaluated with reference to a common protowing ancestor (Fig. 17) has become informative in recognizing insect divisions, lineages and orders. Haas and JKP (2001) and JKP and Lawrence (2004) described a cornucopia of articular character states shared by the higher taxa
Pterygote ovipositor, homologization and basal diversification. Paleoptera: (1–2), †Diaphanopterodea (Permian), with three pleural plates and clawed leglets on segments 7 and 8; trochanteral pleuron 8 bears an articulated TR endite forming the 1st valvula, which sends out a slim dorsal projection articulated to the gonangulum. Gonocoxite 9 (coxa & trochanter fused) dorsally pointed and moved by a muscle, slides and rocks against SCX in gonangulum 9. This back and forth movement is transferred to TR endite 9 (2nd valvula), which slides against endite 8 (1st valvula) and cuts slits (probably into vegetation). The third valvula (gonoplac), guiding movements of 1st and 2nd valvulae, is barely noticeable. Neoptera: (3, 4). In ancestral Pleconeoptera + Orthoneoptera, 3rd valvula is derived from leglet 9 (gonostylus). (5) In Blattoneoptera + Hemineoptera + Endoneoptera, 3rd valvula is derived from the elongated gonocoxite (CX + TR). The same split in lineages occurs in two different types of fusions of the medial sectors, as well as in reductions and folds of anojugal lobes in hind wings. Figs. 1 and 2, after Kukalová-Peck (1992), altered; Figs. 3–5, after Sharov (1966), redrawn. Kristensen (1998) and before thought that the morphology of the insect ovipositor is uncertain, and thus not reliable enough to indicate definitive relationships in higher taxa. This is a simple oversight. When accurately homologized, and researched for evolutionary trends and transformations, insect ovipositors show the basal split of Neoptera into two superlineages. Note that this basal split is repeated again twice, in two sets of wing characters (fusions of medial veinal sectors and states of veinal sectors, folds and flexion lines in hind wing anojugal lobes)
Male genitalia close to pterygote groundplan. In Paleoptera: †Diaphanopterodea (Permian) male genitalia are closely similar to those in fossil and modern mayflies (siphlonurids), in the following characters: Gonocoxites with SCX, CX, TR, completely fused, projecting dorsally to fuse medially and form a bridge (mesocoxite). Gonostyli (telopodites) articulated by PFE (bearing an annulated exite) are serialy homologous and homodynamous with palps, abdominal leglets and the cercal flagellum. Endites articulate under the mesocoxite so that ducted TR endites (gonapophyses) are superimposed on CX endites (penes) (TR ducts in mayflies run on their inner sides). After Kukalová-Peck (1992), updated. Kristensen (1998) and before thought, in summary, that almost all features in (modern) insect limb-derived appendages formed probably de novo, and that all-arthropod homologization is too adventurous to be useful. Therefore, the evolution of limb/wing organ system was summarily rejected (often superficially, as simple error or oversight), or ignored, and not followed. But, unfortunately for higher systematics this highly conservative approach is not harmless. As a fact, which is more apparent every year, it cripples progress in full homologization and a potentially objective, evolution-based character evaluation, on which higher systematics stands (Hennig 1969, 1981). As amply shown by the course of events in the last two decades, it supports phenetics over phylogenetic systematics and evolution. By doing this, it contributes significantly to the ongoing crisis in reconstructing relationships between insect higher taxa and the realistic interpretation of evolutionary events and processes
The limb/wing organ synthesized here is a very broadly diversified organ system in the phylum Arthropoda, which appears to be the best suited for phylogenetic purposes in the higher taxa (Figs. 1–21). This account offers practical examples of different approaches, and of crosschecking with data from other biological fields. The groundplan method applied here was previously tested by this author in all modern insect orders, and used to identify the obscured synapomorphies shared by the modern higher taxa. It was found to be repeatable, good for independent use and for updating, and thus objective enough to offer hope in the present crisis in reconstructing higher phylogenies.
Material
The study of modern wings was focused mainly on basal families, under the auspices of foremost modern specialists. Orthopteroids were studied with D. C. F. Rentz (Australia), D. Otte (USA), and D. Nickel (USA); Coleoptera, with J. F. Lawrence (Australia); Plecoptera, with P. Zwick (Germany); Embioptera, with E. Ross (USA); Ephemeroptera, with W. L. & J. G. Peters (USA) and T. Soldán (Czech Republic); Dermaptera and other blattoids, with F. Haas (Germany); Hymenoptera, with M. J. Sharkey and G. A. P. Gibson (Canada); Neuropterida, with K. J. Lambkin (Australia); and Trichoptera/Mecoptera, with O. S. Flint, JR (USA) (see “Acknowledgements”). Morphology of the limbs in Arthropoda was studied in cooperation with E. L. Smith (USA). This gathering of data spanned over two decades and together with the data from Paleozoic insects provided the objective knowledge of concrete steps in which the character transformations in limb/wing organ system, advanced in pterygote lineages from the Paleozoic to modern times.
Only those genera are listed which are documented by detailed interpretative figures of morphological details, wings and pteralia currently in my files. Many additional specimens have been studied and figured. All modern taxa were compared with numerous fossil specimens from their particular lineage and the differences in character states were recorded and evaluated.
Extant Insects Examined
NEOPTERA
Pleconeoptera: Plecoptera: Arctoperlaria: Pteronarcyidae: Pteronarcella, Pteronarcys; Perlodidae: Dictyogenus, Isoperla, Megarcys, Perlodes. Perlidae: Dinocras, Doroneuria, Oyamia, Paragnetina. Chloroperlidae: Siphonoperla. Taeniopterygidae: Brachyptera, Doddsia, Taenionema, Taeniopteryx. Nemouridae: Amphinemura, Nemura, Nemurella, Protonemura. Leuctridae: Leuctra, Pachyleuctra, Perlomyia. Capnyidae: Capnia, Utacapnia. Antarctoperlaria: Eustheniidae: Cosmioptera, Eusthenia, Stenoperla, Thaumatoperla. Diamphipnoidae: Diamphipnopsis. Grypopterygidae: Dinotoperla, Trinotoperla. Austroperlidae: Austroheptura. Embioptera: Clothoda, Dicrocercembia, Spathembia, Archembia, Tylembia.
Orthoneoptera: Caelifera: Acrididae: Ailopus, Austroicetes, Caloptenopsis, Gastrimargus, Gesonula, Goniaea, Heteropternis, Hippiscus, Choristocetes, Locusta, Pseudaiolopus, Pycnodictya, Pycnostictus, Qualetta, Schistocerca, Sphingonotus, Valanga. Eumastacidae: Biroella, Erucius, Oedaleus, Paramastax, Taeniopoda, Tytthotyle, Trimerotrophis. Pampagidae: Hoplolopha, Eremopeza, Loboscelia. Pneumoridae: Physemacris. Pyrgomorphidae: Desmoptera, Desmopterella, Petasida, Phymateus. Romaleidae: Eutropidacris. Tetrigidae: Scelimena. Tridactylidae: Rhipipteryx. Trigonopterygidae: Trigonopteryx. Ensifera: Haglidae: Cyphoderria. Gryllidae: Acheta, Brachytrupes, Gryllus, Teleogryllus. Schizodactylidae: Schizodactylus. Gryllotalpidae: Gryllotalpa, Scapteriscus. Tettigoniidae: Anabrus, Banza, Capnobotes, Clonia, Metrioptera, Neobarrettia, Scudderia, Tympanophora. Stenopelmatidae: Gryllotaurus, Genus? (Heniciinae), Schizodactylus. Gryllacrididae: Bothriogryllacris, Gryllacris, Hadrogryllacris, Xanthogryllacris. Cooloolidae: Cooloola. Phasmatodea. Phylliidae: Chitoniscus, Phyllium. Phasmatidae: Acontiometriotes, Acrophylla, Cotylosoma, Eurycnema, Palophus, Prisopus. Pseudophasmatidae: Stratocleus.
Blattoneoptera: Dermaptera: Diplatyidae: Diplatys, Haplodiplatys. Pygidicranidae: Pyragra, Echinosoma, Tagalina. Anisolabididae: Carcinophora. Labiduridae: Apachyus. Spongiophoridae: Labia, Marava, Sparatta, Spongiophora, Vostox. Forficulidae: Allodahlia, Ancistrogaster, Forficula. Chelisochidae: Chelisoches, Chelisochella. Mantodea. Mantoididae: Mantoida. Choeradolidae: Choeradodis. Mantidae: Hierodula, Rhombodera, Mantis, Macromantis. Hymenopodidae: Pseudocreobotra, Genus? Empusidae: Empusa. Chaeteessidae: Chaeteessa. Isoptera. Mastotermitidae: Mastotermes. Termopsidae: Porotermes, Stolotermes, Zootermopsis. Hodotermitidae: Hodotermes, Microhodotermes. Kalotermitidae: Neotermes. Blattodea: Blattidae: Eurycotis, Periplaneta. Polyphagidae: Polyphaga. Blatellidae: Neotemnopteryx. Blaberidae: Calolampra, Leucophaea, Panesthia.
Hemineoptera: Cicadomorpha: Tettigarctidae: Tettigarcta. Cercopidae: Leptotaspis? Mahanarwa, Tomaspis. Fulgoromorpha: Eubrachidae: Eubrachys, Platybrachys. Fulgoridae: Enchophora. Copidocephalidae: Copidocephala. Cixiidae: Paranagnia Lophidae: Genus? Flatidae: Ityraea. Ricaniidae: Ricania. Achilidae: Achilus. Heteroptera: Belostomatidae: Lethocerus. Ochteridae: Ochterus. Notonectidae: Notonecta. Reduviidae: Heza. Tingidae.Nabidae: Nabis. Coreidae: Anas. Scuterellidae.
Endoneoptera: Hymenoptera: Xyelidae: Pleroneura, Macroxyela, Xyela. Tenthrediniidae: Trimes. Pamphiliidae: Pamphilius. Pergidae: Perga, Philomastix. Sphecidae: Genus? Argidae: Runaria. Megalodontidae: Tristactoides. Braconidae: HelcionNeuroptera: Ithonidae: Varnia, Ithone, Megathone. Rapismatidae: Rapisma. Dilaridae: Nallachius. Coniopterygidae: Neosemidalis,Spiloconis. Berothidae: Proberotha, Protobiella, Spermophorella. Mantispidae: Ditaxis, Campion. Sisyridae: Sisyra. Neurorthidae: Austroneurorthus. Psychopsidae: Psychopsis, Megapsychops. Polystoechotidae: Polystoechotes, Fontecilla. Osmylidae: Oedosmylus, Eidoporismus. Hemerobiidae: Drepanacra, Notherobius, Psychobiella, Zachobiella. Chrysopidae: Ankylopteryx, Dictyochrysa, Italochrysa, Oligochrysa, Triplochrysa. Nyphidae: Myiodactylus, Norfolius, Nymphes. Nemopteridae: Chasmatoptera. Myrmeleontidae: Distoleon, Palpares, Periclystus, Weeleus. Megaloptera: Corydaliidae: Archichauliodes, Chauliodes, Neohermes, Neoneuromus, Neurhermes, Nigronis, Protochauliodes, Protohermes. Sialidae: Sialis, Stenosialis, Austrocialis. Raphidioptera. Raphidiidae: Agulla. Inoceliidae: Parainocellia, Inocellia. Mecoptera. Notiothaumidae: Notiothauma. Nannochoristidae: Nannochorista. Panorpidae: Panorpa. Choristidae: Chirustus, Taeniochorista. Diptera. Tipulidae: Holorusia. Tabanidae: Tabanus. Asilidae: Diogmites. Trichoptera: Philopotamida: Dolophilodes. Polycentropodidae: Phryganea. Hydropsychidae: Baliomorpha, Hydropsyche. Calamoceratidae: Anisocentropus. Lepidoptera. Hepialidae: Fraus. Neopseusticae: Neopseustis. Agaristidae: Hecatesia. Strepsiptera: Mangelnillidae: Mengenilla. Corioxenidae: Triozocera. Halictophagidae: Coriophagus. Mermecolacidae: Caenocholax, Lychnocolax. Coleoptera. Archostemata: 3 families, 9 genera. Myxophaga: 4 families, 8 genera. Adephaga: 9 families, 37 genera. Polyphaga: 60 families, 150 genera.
PALEOPTERA
Ephemeroptera: one to eight species from: Siphloriscus group: Nesameletus, Siphloriscus, Baetidae. Siphlonurus group: Siphlonurus, Metreletus, Ameletus. Heptagenia. Siphlonisca, Oniscigaster. Mirawara. Coloburiscus group: Heptageniidae, Coloburiscidae, Oligoneuridae, ?Baetiscidae. Ephemerella group: Neoephemeridae. Ephemerellidae. Tricorythidae. ?Baetidae. Leptophlebia group: Neoleptophlebiidae. Palingenia group: Palingeniidae, Ephemeridae, Potamantidae, Euthyplocidae, Polymitarcidae, Behningiidae.
Odonatoptera: one to three genera from these families: Anisoptera: Gomphidae. Aeshniidae. Petaluridae. Cordulidae. Libellulidae. Zygoptera: Coenagrionidae. Lestidae. Calopterygidae.
Fossil Insects Examined
Due to their scarce occurrence and incomplete preservation, the systematics of Paleozoic insects are largely unsettled, disputed and often unresolved. Nevertheless, the character states in the limb/wing organ system usually show quite clearly important similarities and differences between the modern order and its Paleozoic relatives. These indicate character transformation series, in which the individual character states evolved, in one direction since reversals in modern higher taxa are always absent (Hennig 1969, 1981; Figs. 1–21 here). Such irreversible series may reveal which extant species of the particular taxon bear the least derived characters states, which in turn identify the groundplan character states.
The following list includes the institutions, which harbour the Carboniferous, Permian and Mesozoic fossil insect, which were studied by this author. Approximately 2,000 detailed figures documenting mainly Paleozoic data were prepared and used in this study. They include the indirect and direct ancestors in the following lineages: Paleoptera: †Palaeodictyopterida (†Diaphanopterodea, †Palaeodictyoptera, †Megasecoptera, †Permothemistida), Ephemeroptera, Odonatoptera; and Neoptera: Pleconeoptera, Orthoneoptera, Blattoneoptera, Hemineoptera, and Endoneoptera.
Paleozoic and some Mesozoic fossil insects in the following institutions were studied and about 80% figured (ca 1,000 figures and photographs): National Museum, Prague; Charles University, Prague; Museum d’Histoire Naturelle, Paris; Museum of Natural History, London; Humboldt University, Berlin; Paleontological Institut, Russian Academy of Sciences, Moscow; Museum of Comparative Zoology, Harvard University, Cambridge; Peabody Museum, Yale University, New Haven; Field Museum, Chicago; Smithsonian Institution, Washington, D.C.; The Australian Museum, Sydney; Natal Museum, Pietermaritzburg, South Africa; museums in China (Beijing and Inner Mongolia). About 200 figures of Carboniferous insects from private collections in the Chicago area are on file. Early Permian insects were excavated for 12 years at Obora, Moravia, Czech Republic.
Terms, Abbreviations, Graphic Symbols, and Color-Coding
AX. Axalare (axalaria); second column of wing sclerites. Wing articulation contains 8 axalaria.
1, 2, 3Ax. First, second, third axillary sclerite; composite, irregular clusters of sclerites occurring in Neoptera (Fig. 19).
A, AA, AP. Anal vein, anal anterior sector, anal posterior sector.
AWP. Anterior wing process, composed of subcostal and radial proxalare, which are often both secondarily fused to tergum and to each other.
B. Basivenale (basivenalia), sclerotized veinal blood sinus at the veinal base; fourth column of sclerites; wing contains 8 basivenalia, each giving rise to two veinal sectors, anterior A (convex, +) and posterior P (concave, −).
BAS. Basalare. Composite sclerite under the wing.
BT. Basitarsus, a slanted muscled limb podite following tibia; BT is anteriorly disconnected in Archaeogatha and †Monura.
C, CA, CP. Costal vein, costa anterior sector, costa posterior sector. CP is present in most fossils and prominent in some Coleoptera and Hemineoptera, but reduced in most other extant Pterygota.
CX. Coxa, a podite. Coxal outer ramus (CX exite) is retained in the thoracic legs of Archaeognatha.
Coxopodite. Subcoxa (SCX) + coxa (CX) + trochanter (TR) + CX endite + TR endite, articulated or fused. Coxopodites in the head are used in eating (clypeolabrum, mandible, maxilla, labium); in the abdomen in copulating (SCX (articulation in males or upper part of gonangulum in females) + gonocoxite (CX + TR) + TR and CX endites (as valvulae or gonapophyses and penes)). Arthropod limb is divided into coxopodite (upper limb) and telopodite (the leg shaft, palp, leglet, gonostylus).
E, ECX. Epicoxal pleuron, epipleuron. In all Arthropoda, first limb segment is flattened into a pleuron, articulated to the tergum and serving as an articlar site to 10 cylindrical limb segments, each bearing an exite and an endite. Articulated, flattened outer branch of ECX (epicoxal exite) evolved into thoracic wings and abdominal winglets. ECX in the head is fused with capital terga (some ECX extended into sidelobes and fused under the head in Diplura, and are jutting into sides in some Zygentoma); in the thorax, ECX is articulated to tergum (entire in †Monura and Archaeognatha, but fragmented into wing sclerites in Pterygota); in the abdomen of most Paleozoic insects ECX (single or with a fused winglet) is secondarily fused to tergum with a suture. Later, suture is lost and ECX looks like extended tergum.
ET. Eutarsus. Hexapoda in their groundplan contain once subdivided (climbing, flexible) ET (present on 15 limb pairs in †Cercopoda, and on up to 14 in other Paleozoic insects). Only in the thoracic legs of Pterygota, ET may bear up to four subsegments sharing the same main muscle.
F. Fulcalare (fulcalaria); third column of wing articular sclerites, containing 8 fulcalaria.
FE. Femur. Femoral outer ramus (FE exite) is retained in maxillary palps of extant Archaeognatha.
Gonocoxite. In pteygote male genitalia, SCX + CX + TR 9 fused. In female genitalia, SCX forms upper part of gonangulum, which articulates to CX + TR 9 fused.
Gonostylus. Telopodite 9, in genitalia.
HP. Humeral plate, precostal fulcalare & basivenale, fused together with costal fulcalare & basivenale. Sutures are almost always lost. HP occurs only in Neoptera.
J, JA, JP. Jugal vein, jugal anterior sector, jugal posterior sector.
M, MA, MP. Media, medial vein (also, the stem of media, MA + MP fused), media anterior sector, media posterior sector.
Median plate. Medial fulcalare and cubital fulcalare next to each other, articulated or fused.
Ocelli. Median and two lateral sensory organs on the border of acron.
PAT. Patella, a podite. In the maxillary palp, labial palp, abdominal leglet, gonostylus, PAT articulates at both ends; in the leg, PAT fuses to tibia: in Paleoptera with a suture, in Neoptera without a suture.
PC, PCA, PCP, PC strip. Plesiomorphic precosta anterior (PCA) and precosta posterior (PCP) are basally separate in some modern Ephemerida (T. Soldán, unpublished). In other Pterygota, precosta forms a serrated (in Paleoptera) or a smooth (in Neoptera) precostal strip fused to costa (C).
PFE. Prefemur, a podite; prefemoral outer ramus (PFE exite) is retained in the maxillary palp of Archaeognatha; in thoracic legs, PFE is usually fused either to TR or to FE, but TR and PFE are free in Odonata (a plesiomorphy and a proof that PFE exists also in thorax).
PR. Proxalare (proxalaria). The first column of wing sclerites. Insect wing contains 8 proxalaria.
PT. Pretarsus, last arthropod limb podite. In Hexapoda, PT bears curved double ungues (claws) on up to 15 pairs of climbing limbs; the loss of claws is derived.
PWP. Posterior wing process includes anal and jugal proxalare. They often become secondarily fused with the tergum.
R, RA, RP. Radius, radial vein, the stem of radius (RA + RP fused), radius anterior sector, radius posterior sector.
Sc, ScA, ScP. Subcosta, subcostal vein, subcosta anterior sector, subcosta posterior sector. ScA was present in Paleozoic wings. Later, ScA was largely reduced but in the fore wings, it forms a strong, recurrent brace of extant Ephemerida and Odonata, and a long ScA replaces C in Orthoptera with saddle-like tergum.
SCX. Subcoxa, a podite; in the head, all limb appendages articulate with SCX to ECX, which is fused to cranial terga; in the thorax of Monocondylia (Archaeognatha, †Monura), SCX forms pleuron only in on prothorax, but in Didondylia (ΨCercopoda, Zygentoma, Pterygota) it form pleuron in all thoracic segments; in the abdomen of Insecta, SCX forms pleuron together with CX and TR. In female genitalia, SCX foms the outer part of the gonangulum.
Stem (veinal stem). Veinal sectors can fuse into a veinal stem only in three central veins: radius, media, cubitus (R, M, Cu). Veinal stems once formed, are irreversible in higher taxa; they are often longer in derived taxa.
SUB. Subalare, a composite wing sclerite ventrally under the wing.
TA. Tarsus. In Hexapoda, TA includes basitarsus (BT) and eutarsus (ET) once subdivided into ET1, ET2. Under Pterygota, tarsus in thoracic legs may further subsegment into 4–5 articles: BT and ET1, ET2, ET3, ET4.
TEG. Tegula, a glandular organ on top of precostal & costal axalaria in Neoptera.
TI. Tibia. In Hexapoda groundplan, head palps, abdominal leglets, gonostyli and cercopods TI is articulated at both ends. In thoracic legs, it is always slanted and fused to patella, in Paleoptera with a suture, in Neoptera without a suture.
Telopodite. In Archaeognatha, 7-segmented limb shaft (PFE to PT) following coxopodite.
TR. Trochanter, a podite; trochanteral outer ramus (exite) is retained in some Paleozoic insects.
Veinal brace. Two principal veins come together, fuse for a short distance, then separate again.
Veinal sectors. Each wing vein is composed of two veinal sectors, anterior A (convex, +) and posterior P (concave, −): PCA+, PCP−; CA+, CP−; ScA+, ScP−; RA+, RP−; MA+, MP−; CuA+, CP−; AA+, AP−; JA+, JP−. Only in three central veins, R, M, Cu, can veinal sectors fuse basally into veinal stems.
VWP. Ventral wing process; dorsal projection on subcoxal pleuron on which the wing rests. In Neoptera, VWP is usually placed under the second axillary sclerite (2Ax).
Color Code for Morphological Features
Purple. Precostal strip; precostal row of sclerites.
Orange. Costa C, CA, CP; jugal J, JA, JP; costal and jugal rows of sclerites; acron; cranial tergum 6; telopodite; PFE, FE, TI, BT, ET, PT, exites, endites.
Yellow. Subcosta, Sc, ScA, ScP; anal A, AA, AP; subcostal and anal rows of sclerites; subcoxa, SCX pleuron; cranial tergum 3.
Red. Media M, MA, MP. Medial row of sclerites. Cranial tergum 4; epipharynx, incisor, lacinia, glossa; patella.
Green or light blue. Cubitus Cu, CuA, CuP. Cubital row of sclerites. Cranial tergum 1, 5; Trochanter; TR endite; gonostylus; galea, paraglossa; cranial tergum 1 and 2.
Purple. Radius R, RA, RP. Cranial tergum 2; E, or ECX; radial row of sclerites; TR, TR exite, galea, paraglossa.
Light Purple. CX, CX1, CX2, coxal endite.
Brown. Subalare (SUB), basalare (BAS).
Concepts and Terms Used in the Evolution of Organ Systems
To avoid misunderstanding, the interpretation of some Hennigian and other terms and concepts used in the following text is briefly outlined below.
Groundplan
The set of character states of the last common stem-species of a monophyletic group, including all its plesiomorphies and apomorphies. This cannot be observed, unless one travels back in time. Therefore, a groundplan can only be reconstructed in insect lineages based on character transformation series from the Paleozoic to modern times, and on other concrete biological data indicating the course of evolution. Groundplan-level character states define all lower, intermediate and higher taxa and determine their relationships. In a modern higher taxon, they are present in all of its constituent living species; however, they are frequently obscured by post-groundplan autapomorphies added at the family, genus and species level, and may be difficult to recognize. Note that these autapomorphies never replace or otherwise change the basal groundplan states, but are always added on top of them. As an example, in a modern order all families share exactly the same ordinal characters, which are quite often variously obscured by familiar autapomorphies but even then the order holds together. Were these groundplan characters reversed or truly replaced by younger autapomorphies, the order would collapse.
Irreversibility
Hennig (1981) maintained that the groundplan character states of modern higher taxa are irreversible. Therefore, (1) they are not subject to reversals (i.e., they cannot suddenly change into a different state) and, (2) they are always present in their constituent species, either visibly or obscured by various post-groundplan apomorphies. All post-groundplan changes are irrelevant to phylogenetic relationships.
Irreversibility Rule
The present author and co-authors (1983–2004) observed and documented the fact that in a modern higher taxon, all reductions and fusions in limb podites, wing articular sclerites and principal wing veins and branches, which are part of the groundplan, stay reduced and fused in all of its constituent species. The validity of this irreversibility rule in modern higher taxa is easily verified. Examples: In Hexapoda (without any exception) the thoracic leg patella is fused to the tibia (Figs. 3–5). All Pterygota bear a maxilla with coxa + trochanter fused together (Figs. 6, 10). In all hind wings of blattoids + hemipteroids + endopterygotes, the anojugal lobe starts at the anal fold and the AA veinal sector is reduced; in all hind wings of plecopteroids + orthopteroids, the anojugal lobe starts at the claval flexion line, and the AA veinal sector is well developed and branched (Kukalová-Peck and Lawrence 2004). The irreversibility of groundplan characters was never falsified.
Sister Groups in Higher Taxa
These diverged at their ancient (Paleozoic) groundplan level. Their groundplan character states are composed of plesiomorphies, autapomorphies and synapomorphies, but only synapomorphies reveal relationships. In the extant fauna, these ancient synapomorphies are often hopelessly hidden among other similarities, convergencies and parallelisms. Since taxonomic character states evolve from one groundplan to another (rather than by synapomorphies alone), the only possible way to “pin them down” is to research the entire evolution of an organ in all insect lineages, starting with its homologue at the Arthropoda level. This is a monumental task, which may take decades, but there is probably no other way, in the morphological system, to recognize synapomorphies shared by extant higher taxa.
Ancestor, Ancestral Model
The shared ancestor of an organ system in a monophyletic higher taxon is synonymous with its groundplan and groundplan-level character states. As used here in high-ranking taxa, the terms “groundplan”, “ancestor”, “ancestral model”, or “evolutionary model” (such as arthropod protolimb, pterygote protowing) are interchangeable. In an organ system, the shared ancestor serves as a monophyletic base from which all younger character states are derived, and by their distance from which they are judged and evaluated. Ancestral models are of necessity reconstructed, since the real ancestral (usually Paleozoic) stem-species cannot be presented (Raff 1996). They are based on concrete character states recorded in the character transformation series of insect lineages, which were studied and in which plesiomorphic states were identified by using the irreversibility rule. As an example, in the limb/wing organ, they are the least reduced and least fused features found in all constituent species of a modern higher taxon. After the groundplans in all modern lineages down to their orders are researched simple comparisons instantly reveal the synapomorphies shared by the higher sister groups at the moment of their divergence. At this level, the interference from homoplasies is minimal, since they are mostly a post-groundplan phenomenon.
Serial Homology (Homonymy)
Serially homologous organs share a common genetic origin and similar construction, which is underlining all subsequently added modifications. In the limb/wing organ system, these often include synapomorphies shared by the extant higher taxa (Figs. 1–21).
Parallelism
This term refers to apomorphic similarities that have independently evolved in closely related taxa. They may be caused by a common genetic predisposition for an evolution of this character state. Parallelisms are called trends or tendencies when they evolved repeatedly within a monophyletic group.
Homoplasy
This is the general term for non-homologous similarities. It is used both for a secondary presence of a similar state (convergence), or for its secondary absence (reduction or reversal).
Reversal
This is a secondary character state occurring in lower taxa (quite often a reduction), which mimics a “primitive” character state: a derived condition, which superficially looks like a plesiomorphy. Reversals may also involve the reappearance of ancient character states caused by reactivation of a previously suppressed genetic information. However, a return to characters older than 10 million years is very rare as well as uncertain, and 100 million year old or older (Paleozoic) reversals in the groundplan character states of modern higher taxa have not been found and are presumed non-existant (Marshall et al. 1994; Raff 1996).
Systematic Methods used in Reconstructing Phylogenies
Phenetics, or Numerical Taxonomy
This is a non-phylogenetic approach to biological classification based on overall similarity. All character states (plesiomorphies, synapomorphies, apomorphies, and even convergencies) are used as group-defining similarities, assembled in large numbers and processed in a computerized statistical analysis. For criticism and discussion, see Wägele (1996).
Molecular “Systematics”
This rather frequently used term is incorrect and should be dropped. There is obviously only one, biological systematics, which is using different methods and sources of evidence. Molecular data represent the genome, the basic entity of evolution, and are very numerous and relatively easy to define. As discussed in the present synthesis, molecular analyses are increasingly helpful in deciding phylogenies of the lower taxa. Nevertheless, in higher taxa they generate an inordinate amount of inacceptable and even absurd relationships and criteria how to eliminate or at least diminish this flood of false phylogenies is wanting.
Phylogenetic Systematics
This is a method for reconstructing phylogenetic trees and identifying monophyletic groups by the use of homologous, shared derived character states (synapomorphies). In higher taxa, these are identified by first studying the entire character transformation series in their organ systems, and then selecting plesiomorphic character states (un-modified, least fused and reduced) at all taxonomic levels: phylum, subphyla, classes, divisions, lineages, and orders. These plesiomorphies serve in reconstructing their groundplans, which contain distinctive synapomorphies shared by the sister groups. Phylogenetic systematics strives to support all branching points by strong characters based on clearly defined criteria, which are open to criticism, independent use and improvement (see many examples below). The principle of parsimony is used sparingly and only in cases of conflicting evidence.
Computer Cladistics and Parsimony
This method constructs computer-generated cladograms with the principle of parsimony, without previously polarizing and weighing the character states. Out of all possible cladograms those which should be preferred are ones that minimize the number and/or the weight of necessary assumptions of homoplasies. Note that the term “cladistics” is often considered synonymous with “phylogenetic systematics”. But, the absence of character evaluations before they are used in cladograms, as practiced in the mainstream cladism, is a profoundly different approach, characteristic for a different systematic method. As discussed below, this attribute also makes computer cladistics unsuitable for analyzing the relationships of higher taxa.
Mainstream computerized cladistic methods have significantly improved the objectivity of species- and genus-level phylogenies and are presently widely used in entomology. Different approaches exist. As an example, the “exemplar” method generates character states by choosing an extant member of each group to represent the taxon as a whole. The “democratic” method evaluates the most frequently occurring character states as being ancestral (Yeates 1995; Bininda-Edmonds et al. 1998; Prendini 2001). The “ancestral” method attempts to discover the groundplan of each monophyletic supraspecific taxon as the basis for reconstructing phylogeny. This is supplanted by use of exemplars, and a virtually random choice of an outgroup taxon is used as the basis for generating clades (Bininda-Edmonds et al. 1998).
Computer Cladistics and Relationships of the Modern Higher Taxa
As abundantly documented in systematic papers for more than a decade, the mainstream computerized cladistic methods successful in lower taxa (species, genera), consistently fail to deliver convincing phylogenies in the higher taxa (order, lineages, divisions, classes, subphyla). Various reasons and remedies have been proposed. The conviction of some modern systematists that only the computerized cladistic method has the capability to objectively reconstruct any part of the hexapod phylogenetic tree has become so overwhelming and uncritical that they now blame morphology itself (i.e., the essence and raison d’ être of morphological systematics itself!) for being “unable” to deliver synapomorphies. They naively hope that molecular characters will eventually provide better evidence, sometime in the future. But, this will not happen, for reasons discussed below. A peculiar situation has developed in which massive advancements in computerized methods are very useful in lower taxa (species and genera), but which have a mixed effect in intermediate taxa (families), and have strongly regressive influence in higher taxa (suborders and upward taxa). Note, that to save space, all currently used systematic methods limited to exploring higher-level phylogenies by using only the character states of living, Tertiary and Mesozoic insects, are here collectively referred to as the post-groundplan methods.
The synthesis offered here lists multiple reasons, which have caused or contributed to the present critical situation in insect higher systematics. The “groundplan method” for pursuing evolution of the richly diverse limb/wing organ system, and honoring the systematic rules outlined by Hennig (1969, 1981) is offered below as a way to better understand and, hopefully, improve the reconstruction of phylogenies.
Groundplan Method Used in Phylogenies of the Extant Higher Taxa
Hennig (1969, 1981) maintained that the groundplan characters of all extant higher taxa are (1) irreversible and (2) plesiomorphic within the taxon. Such characters can be recognized in the constituent living species of the higher taxon by two criteria: (1) their shortest distance from the shared, single, common ancestor (=monophyletic groundplan), and (2) their irreversibility within their entire higher taxon. The systematic method used in this process by this author and co-authors, here called the “groundplan method” differs from all other currently used systematic methods by using all-arthropod evolutionary perspective and evidence from other biological fields to homologize and evaluate the character states before they are phylogenetically analyzed. This broad dataset is also used for crosschecking identifications, and to interpret evolutionary processes.
The groundplan systematic method is used by phylogenetic systematics to reconstruct higher phylogenies by researching the evolutionary history of organ systems. It explicitly infers the shared monophyletic ancestor of the organ system corresponding to the taxon (here, the groundplan of the arthropod and hexapod limb (Fig. 1) and the pterygote wing venation and articulation (Figs. 17–19)). This ancestor is instrumental to a full homologization and judging of the character states in orders and lineages. It is based on concrete character states recognized as plesiomorphic in modern species, by using the character transformation series from Paleozoic to modern times as recorded in all modern lineages, and with the irreversibility rule. The homologue of the hexapod limb and wing in other arthropods is researched, together with its expression and compatibility in other biological fields: ontogeny, embryology, genetics, developmental genetics, experiments in transplants, and others. Assembling the basal data is a broad and complex process, which may take decades and which remains permanently open to improvements by newly discovered evidence. Thus, phylogenies delivered by the groundplan method are an ongoing process, presenting the most informed results according to the presently available evidence. The groundplan method has been long used in reconstructing phylogenies of vertebrates and marine invertebrates, and is widely practiced in paleontology. As shown here (Figs. 1–21), it is equally capable of finding higher synapomorhies and reconstructing higher phylogenies in arthropods.
Pioneering Use of the All-Arthropod Limb Organ System by A. G. Sharov
A. G. Sharov (in his ground breaking 1966 book) pioneered the use of a broad arthropod context and Paleozoic fossils in insect higher phylogeny. His results were extensively discussed and further developed by Hennig (1969, 1981), Boudreaux (1979), E. L. Smith and other leading arthropodologists, but not surprisingly, they were severely but unjustly criticized by the users of only extant characters and advocates of “uniramous” hexapod appendages, sensu S. Manton (1977). Sharov set phylogenetics of Hexapoda on a modern, sounder, all-arthropod evolutionary base. A new, broad and significant role for Paleozoic insects was recognized, superior to alpha taxonomies using figures in which all “uncertain” (read: ancestral and thus unfamiliar, difficult to interpret) states were mercilessly left out not to jeopardize “objectivity” (read: interpretation would take too much time). For Sharov, Paleozoic fossils offered invaluable and direct evidence of the plesiomorphic states in the evolution of organ systems. The new approach, highly demanding on preparatory skills, patience, observation techniques, comparative knowledge of arthropod structures and of supporting evidence in other biological fields instigated up to date many surprising interpretations of evolutionary processes and higher phylogenies, which would otherwise remain unknown.
New Limb Homologization by E. L. Smith and the Present Author
During the two decades following Sharov’s premature and tragical death, the ancestral limb model was updated, completed and subsequently broadly verified in all modern arthropod subphyla, by E. L. Smith. The detailed evidence in fossil palps, antennae, legs, leglets and cerci was provided, applied and crosschecked in other biology fields by the present author. The same ancestral model of pterygote wings was actually independently reconstructed twice: the first published in 1983 was based on a long-term gathering of the character transformation series in all insect lineages. The second was found (in 1994) to be actually present in the prothoracic winglets of two different superorders of Carboniferous insects! (See discussion below). In 1983, this author proposed that insect wings originated from the flattened exite of the epicoxal pleuron, present in all Arthropoda. The insect veinal system and wing articulation was also homologized in 1983, and several interpretive improvements were offered later. The updated groundplans in all Neoptera lineages were proposed but JKP in two co-authored publications (Haas and Kukalová-Peck 2001; Kukalová-Peck and Lawrence 2004), and in Paleoptera lineages (in co-authorship with J. Peters and T. Soldán) they have been recently updated and are ready for publication.
When E. L. Smith and I started in 1978 to research the limb transformation series in the arthropod/insect higher taxa, we soon noticed the absence of reversals in their characteristic character states, which was documented previously by Hennig (1969). Distally from the first epicoxal podite, which was flattened into the epipleuron (= archipleuron) (Kukalová-Peck 1998), there were 10 plesiomorphic, cylindrical and muscled podites articulated at both ends, which bore two articulated mobile rami (Fig. 1). The outer ramus (exite), which evaginates from the membrane between two podites, was conical, articulated, mobilized by muscles from both podites (note that exites tend to migrate upwards and into the proximal podite, and often become immobilized or fused) (Figs. 8, 10, 12). The inner ramus (endite) evaginates from one podite, is conical, articulated and mobilized by muscle from the podite (endites may also fuse with their podites, e.g., in the mandible (Fig. 6). The highest number of rami occurs in trilobites, the lowest in Chelicerata. Paleozoic winged insects retained up to four short, and already very weak exites (Figs. 3, 12), which completely disappeared in extant pterygotes (Figs. 3, 4), but remain quite distinctly expressed in living Archaeognatha (Figs. 8–10, 12). Epicoxal exites (functioning as gills) are retained in some living Crustacea, e.g., in branchiopods and syncarids (Averof and Cohen 1997).
Ultimately, five fully articulated, muscled podites, four exites and two endites, retained in fossil as well as some modern insects (Figs. 3–21), were found and added to the classical Snodgrass limb model (1935). The most completely retained ancestral insect limb is the maxilla in modern Archaeognatha, bearing the complete set of 10 cylindrical articulated and muscled podites, subdivided into the original coxopodite and telopodite. Modern Archaeognatha also harbour relictual limb exites (Figs. 8.1, 10.1, 12.2, 12.3) and offer a very well-retained archaic epipleuron, which is still fully articulated at both ends to the tergum and subcoxa (rather than secondarily fused) to either of them (Figs. 7–9). The correct interpretation of the insect thoracic pleuron is also very clearly revealed in Archaeognatha (Figs. 8–12), in which the subcoxa forms a pleuron in the prothorax, but still remains cylindrical in the meso- and metathorax (Kukalová-Peck 1998, Figs. 19.3e, 19.5a, b). This shows that the thoracic pleura in Pterygota are formed by the flattened subcoxa. In the extinct subphylum Trilobita, some limb podites bore an outer and most an inner ramus (an exite and endite) (Kukalová-Peck 1991, Fig. 6.1A), but in post-Paleozoic Arthropoda, all non-functional rami became reduced. Exites and endites that developed a special function were not reduced but adapted and modified. Examples: The flying epicoxal exites in Pterygota (wings, Fig. 17), tactile coxal exites in Archaeognatha (Figs. 8, 10, 12), locomotory coxal exites in aquatic Crustacea (“swimming legs”), and flattened epicoxal, coxal and subcoxal respiratory exites in some basal aquatic Crustacea (Syncarida, Anaspides, Tasmanian shrimp; Branchiopoda, Artemia, etc., serving as gills. Retained endites in arthropod mouthparts are used for chewing (Fig. 6), in the abdomen as abdominal vesicles for absorbing moisture (Figs. 2, 15), and in genitalia as gonapophyses, penes, and ovipositor valves for copulating nad laying eggs (Figs. 2, 4, 15, 20, 21). Coxal endites articulate coxae to the thoracic sternum in Endopterygota.
Abdominal pleural membranes were found by us to be supported by flattened podites. Three separate pleura (subcoxal, coxal, and trochanteral) were distinctly delimited by sutures in the abdomens of fossil Diaphanopterodea (Fig. 20). These, and abdominal structures in modern Ephemeroptera and extinct and extant Zygentoma, were instrumental in reconstructing the ancestor (groundplan) of the abdomen in Dicondylia (Fig. 15), which also shows the position of abdominal endites as in Fig. 1. In Pterygota, Paleoptera (Palaeodictyopterida + (Odonatoptera + Ephemeroptera)) lost the pregenital abdominal endites (a synapomorphy at the division level).
“Absence” of Certain Limb Podites and Rami in Extant Entomofauna
Plesiomorphic Limb Features (Fig. 1)
These are much better expressed and visually more evident in Paleozoic than in Mesozoic-to-extant arthropods. Progressive simplification, loss and “disappearance” of plesiomorphic character states are a common evolutionary transformation. The adage “evolution is reduction and tinkering with the rest” is widely considered valid. In arthropod appendages, there is enormously varied reduction/fusion in the number of limb podites, rami and wing veins in the modern entomofauna (Figs. 1–21). Trilobites were also much more complex in the Cambrian than they were in the Permian (Webster 2007). Countless other examples exist. Post-groundplan reductions, fusions, parallelism and homoplasies especially target and confuse the serial homology of limb-derived appendages, and superficially defy their provenance from a single all-arthropod ancestral model. The post-groundplan modifications are quite irrelevant to higher-level relationships. Nevertheless, they turned some systematists practicing computerized methods using parsimony against the groundplan concept of Hennig. Quite erroneously, this is now seen by some as one of several available systematic methods. Instead, judging characters at their groundplan level is the fundamental prerogative of systematics at all taxonomic levels. Since lower taxa are naturally at or close to their groundplan level, there is no problem and computerized cladistic methods are able to remove the interfering reversals (which are not part of the groundplan!). However, higher taxa have Paleozoic groundplans, which are too obscured by later autapomorphies to be obvious, and must be morphologically researched. Computerized methods, mainstream cladistics using parsimony, methods using statistics and molecular analysis, are not equipped to research ancient groundplans of modern higher taxa, hence they are powerless to distinguish synapomorphies and to deliver, well-reasoned and repeatable phylogenies based on objectively defined character states. See examples below.
Interpretive Problems with Reductions and Fusions
As evident from Figs. 1–21, the muscled and articulated limb podites of appendages, articulated exites and endites, pleura delimited by sutures, abdominal leglets and winglets, wing vein sectors and articular sclerites, were all much more plentiful, and more distinctly expressed, articulated, mobile, regular, larger and more conspicuously serially arranged and visually noticeable in the Paleozoic, than in Mesozoic, Tertiary, and Quaternary insects. Serial homology of limb/wing organs was much more evident (Fig. 18). While the ancestor was rich in character states, these almost always became partially fused and/or reduced in the descendants. The catch is that these changes happened in each lineage differently and to different characters. The resulting gaggle of states has provided almost as many interpretive possibilities as there are systematists. This is shown especially well in the pterygote wing articulation, which looks utterly different in Paleoptera and Neoptera, yet monophyly of wings is not in doubt. Attempts to homologize pterygote wing sclerites based on their state in modern insects were an exercise in creative engineering and “supported” three phylogenies: (Paleoptera + Neoptera); (Ephemeroptera + (Odonatoptera + Neoptera)); and (Odonatoptera + (Ephemeroptera + Neoptera)) (Kukalová-Peck 1983, 1998; see below). In contrast, clues from fossils showed that ancestral sclerites were quite small, numerous, and arranged in regular rows protecting blood channels (Figs. 2, 14, 18.). In Neoptera, they were assembled into three irregular, oblique clusters (the first, second, and third axillary), while in Paleoptera all fusions followed an arrangement into rows (Figs. 14, 17–19). Axillaria and articular plates in some modern insects still bear weak sutures delimiting the original, small sclerites. But, without clues from fossils, these weak, diminutive sutures would have been very probably overlooked and homology in pterygote articulation never would be resolved. Homologized articulation offers dozens of higher-level character states, which would remain unknown (Kukalová-Peck 1983, 1998; Haas and Kukalová-Peck 2001; Kukalová-Peck and Lawrence 2004).
In spite of their distinct expression in Cambrian trilobites, Cambrian-to-extant Crustacea and Devonian-to-extant Insecta, the existence of arthropod groundplan rami (exites, endites) in Hexapoda is strongly and repeatedly rejected by some insect systematists (e.g., by Kristensen and by Grimaldi and Engel 2005 who consider them to be “secondary lobes”). The most severe criticism of the contributions by Sharov, Smith and the present author have concerned the groundplan limb features, present in fossils but reduced in most (but not all!!) modern insects: TR, PFE, PAT, BT in thoracic legs, abdominal pleura delimited by sutures, abdominal articulated winglets and segmented leglets, segmented gonostyli and cerci, and especially ancient exites and endites serially present in mouthparts, vesicles and genitalia (Figs. 1, 2, 13, 20, 21). The renewed mistrust of polyramy and serial limb homology by some established systematists is hard to understand, especially since the general rejection of “Uniramia” (an erroneous “uniramous” phylum combining Myriapoda + Hexapoda + Onychophora) took place less than 20 years ago!! The “Swiss army knife” multifunctionality of rami in arthropod limbs has been known to biologists for about two centuries. Up to four large leg exites engaged in respiration and swimming, are present in many living Crustacea (e.g., in Branchiopoda and Syncarida). In modern basal Insecta, jumping bristletails Archaeognatha bear exites on up to three different podites (Figs. 8, 10, 12), and the wings and abdominal winglets of mayfly nymphs in Pterygota are in the position of and homologous to the epicoxal exites (Figs. 15, 17). Archaeognatha retained plesiomorphic, fully articulated, Arthropoda-level epicoxal pleuron (Fig. 9), and their maxilla represents the 10-segmented fully articulated limb of Cambrian Arthropoda, subdivided into the classical coxopodite and telopodite and still retaining two exites and two endites! (Compare with Figs. 1 and 8). Therefore, rejecting the presence of rami in Insecta is a simple (albeit far reaching) error, for anybody who accepts Arthropoda as a natural phylum. It also stunts and destroys higher phylogenetics.
Lower and Higher Taxa Have Different Systematic Problems
Systematic Rules Defining Informative Character States
The character states in all taxa are systematically informative only when they are (1) fully homologized, and (2) at the groundplan level of the respective taxon (Hennig 1969, 1981). Interfering homoplasies, reversals, convergencies, parallelisms, etc. are almost always post-groundplan phenomena, which were added only later. Hence, the groundplan level of all taxa also contains the largest number of distinctive synapomorphies, which are not yet obscured by post-groundplan adaptations.
Insects and the Great End-Permian Extinction
Modern higher taxa, subphyla, classes, divisions, lineages and almost all modern insect orders diverged during the Paleozoic. Therefore, they have pre-Triassic groundplans and synapomorphies, which often include ancient features. These features are largely suppressed or modified in the extant fauna (especially exites and endites, Figs. 1–6). About 252 million years ago, at the end of the Permian, the global ecosystem collapsed, gradually and over a long period of time. According to a relatively new hypothesis based on a large set of geological data, a huge extra-terrestrial body collided with Earth near Antarctica. Strong shock waves travelled on the global surface as well as through the core to the opposite side of the globe causing extremely powerful antipital focusing. This opened the Siberian traps, an immense area of basaltic volcanos, which poured sulphur-containing lava into the ocean and instigated a massive increase of sulphurous bacteria. These produced huge quantities of hydrogen sulphide, a poisonous compound that removed oxygen from the seawater, killed 98% of marine species and eventually also lowered the amount of oxygen in the air. Whatever the cause, an estimated 90% of all animal species went extinct (compared to about 65% at the Cretaceous-Tertiary boundary), and the terrestrial ecosystem probably did not fully recover for at least 5 million years (Erwin 1993; Benton 2003).
According to Hennig (1981, p. 345) the insect fauna suffered a massive reduction and repopulated dry land from as little as 40–50 stem-species (!) Most of the plesiomorphic Paleozoic groups feeding on Paleozoic plants, representing about 85% of Carboniferous entomofauna, including the lineage of haustellate Palaeodictyopterida and almost all ancestral hemipteroids, became extinct except for several relics (a probable reason why in modern fauna hemipteroids are so unevenly represented and disjunct). The crown of the insect phylogenetic tree was cut off as by a dull scythe except for a few mostly-derived branches. Most insects bearing plesiomorphic features were wiped out, and with it “missing links” documenting evolution in insect organ systems. When Triassic insects diversified from the few surviving “stem species” and adapted to changed terrestrial habitats, almost every aspect of this entomofauna was remarkably different from its Paleozoic ancestors: in morphological diversity, taxonomic composition, variability, ecology, ethology, and very probably also in ontogenetic development (the numbers of instars were probably dramatically lowered and a metamorphic instar was added independently in every pterygote lineage). As a well-known fact, Mesozoic insects as a whole are much closer to the Tertiary and Quaternary fauna, than they are to their close Paleozoic neighbors.
The Great end-Permian Extinction had an enormous impact on insect evolution, phylogeny and systematics. While in modern orders most of their Mesozoic, Tertiary and extant families, genera and species bear limb appendages with similar fusions and reductions, the groundplans of orders, lineages, divisions and classes often include unfamiliar character states, visibly retained in only a few modern basal species (see good examples in Coleoptera, by Kukalová-Peck and Lawrence 2004). These are often part of the ancestral groundplan and harbour varied clues to higher-level synapomorphies. Therefore, very old groundplans of modern higher taxa cannot be reliably identified based only on the living fauna. After many failed attempts, this idea seems to be gradually accepted by mainstream entomology.
Problems in Lower Taxa
Modern insect species and genera have Quaternary, Tertiary, and occasionally Mesozoic groundplan character states, which are close morphologicaly and can be fully homologized without much trouble. But, there are considerable problems with their stability because they are often mixed together with reversals. Many other problems, e.g., with cryptic species, sibling species, ring species, inter-specific hybrids, etc., are solved with help from molecular analysis, which may provide missing clues as to which are the genuine groundplan character states in lower taxa. In contrast, molecular analysis is highly unpredictable in higher phylogenies, and often delivers unrealistic results.
Under these circumstances, the post-groundplan methods applied in lower-level phylogenies work (1) with fully homologized character states, which are at or near the groundplan level, and (2) with significant sample sizes of living species, which hold the informative character states. Hence, they are in agreement with two basal rules of phylogenetic systematics as formulated by Hennig (1969, 1981) (using fully homologized character states, at their groundplan level).
Problems in Intermediate Taxa
Almost all modern families originated during the Mesozoic and Tertiary and therefore, some of their synapomorphies can be found by currently used systematic methods. The groundplan method is useful in recognizing the ordinal and higher character states, which are shared by the constituent families as symplesiomorphies.
Problems in Higher Taxa
Almost all modern insect higher taxa (suborders, orders, lineages, divisions, and classes) have Paleozoic groundplan character states, in which they are related, and which include reliable synapomorphies. The problem is that the currently used post-groundplan methods cannot find groundplan states, and thus cannot separate the immensely old synapomorphies shared by extant higher-level sister groups from various “false synapomorphies”, homoplasies and parallelisms added in their intermediate and lower taxa. The Great end-Permian Extinction disrupted the gradual character transformation series of organ systems and made them seemingly incomprehensible. Therefore, they must be researched first.
Many Paleozoic ancestral features survived until modern times, but remain unidentified. To determine how many living species actually carry the unchanged subordinal groundplan character states in the veinal system, Kukalová-Peck and Lawrence (2004) inspected the hind wings of beetles in all basal families. The least derived venational characters in suborders judged with respect to protowing, were retained in <5% of modern species, scattered over several basal families. Detecting them took time, but once available, they instantly revealed several previously unknown synapomorphies showing subordinal relationships under Coleoptera with reference to their distance from the shared ancestor.
Homologization of organ systems between insect orders and higher taxa presents yet another major problem. The nomenclature is often tailored to suit the needs of a single order, while postulated homologues in other orders are often only intuitive. The post-groundplan approach typically uses (1) character states at an unknown distance from the common single ancestor, (2) states which are often incompletely or wrongly homologized and, (3) which include an unknown number of homoplasies and parallelisms masquerading as synapomorphies and impossible to verify. Typically, several “possible” solutions to phylogenies of modern higher taxa are found and pondered (e.g., Kristensen 1991, 1995, 1998; Beutel and Pohl 2006). The problem is that arthropod evolutionary morphology is still little known. Across all arthropod orders, so far, only the insect wing and most of the limb structure have been fully homologized (but the limb derived insect hypopharynx and some derived insect genitalia are still inadequately understood) (Figs. 1–21) (Kukalová-Peck 1991, 1992, 1998; Haas and Kukalová-Peck 2001; Kukalová-Peck and Lawrence 2004).
Limb/Wing Structure: Its Use in Higher Phylogenetics by the Groundplan Method
Most extant winged insect orders and/or their sister groups (except Lepidoptera and some small or parasitic orders), were recorded in the Carboniferous or Permian. This includes the following orders: Ephemeroptera, Odonatoptera, Plecoptera, Embioptera, Orthoptera, Blattodea, Coleoptera, Megaloptera, Neuroptera, Mecoptera, Trichoptera, Diptera (CSIRO 1991; Carpenter 1992). The limb/wing appendages in each higher taxon bear a typical irreversible pattern of fusions, reductions and modifications. Since they are irreversible (=never revert to the ancestral or other state), the plesiomorphic states of the characters in limb/wing organ (found in constituent modern species and identified by referring them to the ancestral model) are at the same time the groundplan character states of the analyzed higher taxa.
Also, because of their irreversibility, the groundplan-level adaptations in the limb/wing organ system such as fusions, reductions, and braces (in the limb podites, exites, endites; wing articular sclerites; principal wing veins) move in a predictable direction. Fusions can only be added but never un-fused, and reductions can only increase but reduced features can never come back, in all representatives under each modern higher taxon. Thus, in an informative sample of constituent species of an extant order, the least reduced and fused states found in limb podites, wing venation, and wing articulation are also the groundplan-level character states of their respective order (or other higher taxon). This predictability is the Rosetta stone of higher phylogenetics. It provides an objective, independent, and repeatable technique to identify the higher groundplan character states in living species (which in turn inable precise homologization and reveal synapomorphies).
Search for the Shared Ancestral Limb Model
It should be underlined that morphological ancestral models have taken decades to reconstruct. They must be based exclusively and only on concrete character states found scattered in all Arthropoda, Hexapoda, Insecta, and Pterygota. Thus, a missing parts cannot be “filled in”, as they are in scientific life-like reconstructions of fossil animals, but must be found in actual specimens. Before being presented, an evolutionary model must be repeatedly tested in all lineages. It must also be found fully compatible with all available data in ontogeny, genetics, embryology, etc. Insect limb models (Figs. 1, 6, 15) took almost 20 years to reconstruct and crosscheck, and the pterygote wing model (Figs. 17–19) took 30 years. This labor-intensive background is mentioned here not to promote this author’s interpretations, but to show in real numbers how long it takes to make sure that all character states are at their plesiomorphic level. A fully documented ancestral model consumes a great amount of time. Yet, how can anyone expect to reconstruct a credible higher phylogeny without ever knowing how the common ancestor looked like, i.e., which character states are plesiomorphic and which are derived, and how much they became transformed to be derived, in modern higher taxa?!?
The arthropod limb model (Fig. 1) revealed a number of features, which were previously unknown or misinterpreted: the presence of the epicoxal pleuron shared by all Arthropoda; the identity of insect clypeolabrum; the composition of mandible; maxilla; and labium in the head (Fig. 6), subcoxal pleuron in the thorax (Figs. 7–9), and subcoxal + coxal + trochanteral pleuron in the abdomen (Figs. 15, 20). In the thorax, epicoxal pleuron and its exite were identified as the homologue of the wing articulation and flattened wing ramus (Figs. 7–9). In the abdomen, it showed serial pregenital leglets (starting at prefemur (PFE) and primitively bearing double claws) as homologues of the previously enigmatic abdominal “styli”, vesicles and gonostyli; gonocoxites in male and female genitalia were recognized as coxopodites, and genital appendages as endites (Figs. 15, 19, 20) (Kukalová-Peck and Richardson 1983; Kukalová-Peck 1983, 1985, 1987, 1991, 1992, 1998; Riek and Kukalová-Peck 1984; Haas and Kukalová-Peck 2001; Kukalová-Peck and Lawrence 2004).
It is most regrettable that the Atlas of Arthropods by E. L. Smith, the most broadly documented, comprehensive, exquisitely detailed, accurate and fully illustrated arthropod comparative-morphological analysis that ever existed, has not found enough support to be published. As shown below in several examples, the broadly documented evolution of organ systems falsifies in arthropods and insects almost all previous interpretations of evolutionary processes and most of the higher phylogenies based recently on the limited and often misleading post-groundplan evidence. Understandably, this has raised resentment in some systematists, who spent their careers trying to resolve insect phylogenies by using modern, sophisticated and well-tested systematic method generally known to be very helpful in lower taxa. Alas, it also inspired some imaginative and determined counter-measures and witch-hunt in entomo-politics, including pretending that we, or our contributions to insect evolution, or even both, never existed. The term of choice evaluating our results is “controversial”, while reasoned criticism is superficial or not offered. An exception is the open criticism offered by Kristensen and a few other authors, which is discussed and rebutted below. As a result, most unfortunately, only a relatively small part of E. L. Smith’s monumental comparative morphological atlas of arthropods was published, which was done in cooperation with the present author (Kukalová-Peck 1983, 1991). This author researched the supporting evidence to the limb-derived appendages, available in fossil and in other biological fields. JKP alone is responsible for interpreting the origin of insect wings and wing articulation, and the diversification of the wing organ into divisions, lineages, and some orders. As evident from the “List of genera examined”, an extensive file of large, detailed figures exists and can be offered in further considerations. About 25% of character analysis done in cooperation with specialists in extant groups, and only about 5% of the figures, is published.
Search for the Shared Ancestral Wing Model
Pterygota are the largest, most diverse, and most successful group in the Animal Kingdom, to a large extent because they have wings. Insect wings contain veinal systems and articulations, which offer many easily observed synapomorphies shared by higher taxa, such as characteristic and sometimes unique veinal fusions, braces and reductions, modifications of the anojugal lobe, fusions and desclerotizations in articular sclerites, etc. I first noticed the potential for using veinal stems and braces in reconstructing higher phylogenies during my work with Paleozoic insects. Since about 1970, I started recording the character transformation series under separate fossil and extant lineages. Pterygota are considered as a monophyletic taxon, hence insect wings evolved from a single ancestral protowing veinal system and a single protoarticulation. The search for a concrete character transformation series, in which the veins and articulation change between the Paleozoic and present times, was done by recording concrete states in all eight principal veins and in all eight rows of articular sclerites. Typical transformations always present in divisions, lineages, and orders were documented in detail in many figures (see “Material” here).
Early in the project (before 1983), the shared ancestral protowing model was hypothesized based on all available evidence, and then repeatedly cross-examined against additional samples of living species from all extant lineages. In a useful ancestral model, all evolutionary components must evolve consistently with each other plus with all pertinent biological evidence. The “Eureka” moment came in 1983, when all existing wing characters in all Pterygota could be at last flawlessly derived from a single protowing pattern by always using exactly the same irreversibility rule: at the groundplan level of a higher taxon, fusions between veinal sectors do not unfuse, and reduced features do not re-appear (see examples in the following paragraph). In systematic practice it means that, in higher taxa, (1) wings with more fused and more reduced characters are always more derived—and vice versa; and (2) the least fused and reduced character states found in the constituent species of an order or other higher taxon, are the ordinal (lineageal, divisional) groundplan characters, which of course contain reliable higher synapomorphies. In insect wings, which are adapted to their complex type of flight without any known reversals, these rules follow a basic assumption of phylogeny: “Complex structures are never regenerated in the same form” (Ross 1974). See Raff (1996) for genetic background and further evidence.
The protowing model, based on a huge dataset, served four purposes. (1) It confirmed beyond reasonable doubt that the fusions, braces and reductions, in principal veins and sclerites characteristic for each lineage and orders are indeed irreversible (=they can be obscured by reduction or enhanced, but they cannot become “undone”). Examples of irreversible synapomorphies: Paleoptera share as synapomorphies two veinal stems, of M and of Cu; in Neoptera these stems are absent (in plesiomorphic wings only); Odonatoptera and Ephemeroptera share a unique, composite, protruding anal brace, which supports the anal area, a unique anterior articular plate formed by identical sclerites, and a set of five braces in veins near wing base; in Orthoneoptera and Pleconeoptera, MA basally fuses to MP in the veinal stem of M; in Blattoneoptera + Hemineoptera + Endoneoptera, MA basally fuses with R (=the stem of M is absent), etc. (2) Protowing model pooled together all plesiomorphies in all lineages into a single reference scheme, which shows at a glance which veinal and articulation character states are derived and shared. (3) It revealed the exact combinations of groundplan fusions, reductions and modifications, which were typical for each one of the modern orders, lineages and divisions. (4) Last but not least, the groundplan—level characters included “fresh” synapomorphies (i.e., numerous, unmodified, not yet overwhelmed by homoplasies), which provided the defendable evidence for modern higher reconstructed phylogenies, and can be easily crosschecked or improved.
The Question of Irreversibility of Wing Character States in Pterygote Higher Taxa
The systematic rule determining that inside every higher pterygote taxon, significant veinal fusions and reductions can be only added and never removed are documented here in Figs. 1–21, and in many publications (Haas and Kukalová-Peck 2001; Kukalová-Peck 1969, 1970, 1978, 1983, 1985, 1987, 1991, 1992, 1998; Kukalová-Peck and Brauckmann 1990, 1992; Kukalová-Peck and Lawrence 2004; Kukalová-Peck and Richardson 1983; Riek and Kukalová-Peck 1984; Sharov 1957, 1966; Smith 1970, 1988). The irreversibility rule plays an important role in higher-level phylogenies. Example: in the protowing ancestral model for Pterygota (Kukalová-Peck 1983) all veins were found to be composed of two veinal sectors (note that two precostal veinal sectors were discovered recently in modern mayflies (T. Soldán, personal communication)). In the pterygote protowing, two veinal sectors of the medial vein and the cubital vein are basally separate (two plesiomorphies). Modern Paleoptera (Ephemeroptera + Odonatoptera) share a unique anal brace composed of three veinal sections aligned in a ridge (AA & AA1 + 2 & AA1 & cross-vein brace to cubitus); this becomes longer, shorter, partly superimposed, extended or variously modified in different families, but is always present (a Paleozoic, groundplan-level synapomorphy). In Neoptera fore wings, the anal basivenale forms a protruding anal bar, branch AA1 + 2 is distant from branch AA3 + 4, and AA3 + 4 is always basally fused with AP veinal sector. These and other distinctive higher-level synapomorphies are easily recognized, verified, and judged by their distance from the all-pterygote protowing (containing plesiomorphies) (Kukalová-Peck 1998; Kukalová-Peck and Lawrence 2004).
As a fact, the presence of irreversible wing characters in higher taxa can be easily verified in living insects (e.g., the stem of M (MA + MP fused) is present in all Orthoptera and Plecoptera, and a simple observation or cut near wing base shows that in all Blattodea, Hemiptera and Endopterygota, MA basally fuses to R instead), Others are obscured by later adaptations (e.g., jugal area in the wing of fossil Paleoptera is strongly suppressed, and in extant Paleoptera is non-existant (a synapomorphy of paleopterous subtaxa). The evidence for irreversibility is overwhelming and cannot be disproved by experiments in Drosophila, as suggested by some entomologists. These experiments are done under induced, sometimes exotic conditions, which include homeotic mutations and other phenomena mimicking reversibility, and tend to produce lethal mutations. They can hardly falsify the reality of easily tested groundplan characters, such as that all blattoids have anojugal lobe in the hind wing starting at the anal fold, while in orthopteroids and plecopteroids it starts always at the claval fold; all Neoptera have three identically constructed axillary sclerites, etc. Examples of the irreversibility of groundplans are countless (see Figs. 1–21). Raff (1996), Marshall et al. (1994) and Raff (1996) have analyzed genetic attributes and evolutionary constraints versus irreversibility of the old Paleozoic groundplans.
On the opposite side of the genetics-based argument proposing many limitations, is the radical application of the post-groundplan approach, which stimulated the resurrection of the “cowboy” concept that “everything is possible” and “the sky is the limit” in evolution. This confused line of thinking is presently spouting eminently forgettable higher phylogenies and wasting loads of precious paper.
Paleozoic Insects, Their Character States Retained in Living Insects
Many Paleozoic fossils are difficult to interpret. Many are squashed in a dark, grainy matrix, and riddled with artifactual fractures masquerading as sutures. Many also contain unfamiliar features, which may take years to resolve. Weakly sclerotized, minute features, such as exites, tarsal articles, claws, bristles, abdominal telopodites, clypeo-labral and cranial sutures, ocelli, ommatidia, endites in the mouthparts and genitalia, etc., are almost invisible on an uneven, reflective matrix. Knowledge of arthropod structure, experience in drawing a crushed fossil by repeatedly changing light angles and position, employing enhancing observation techniques (ammonium chlorite, alcohol, and glycerine), good vision, and last but not least a talent and patience for sorting out deformed criss-crossed shapes, are quite necessary prerequisites for recognizing important but often minute, ancestral character states. Untrained observers often can see an ancestral feature only after it is placed in the middle of the field and enhanced by a low angle light effective only in a single position! Photographs are difficult to make and, once published, difficult to interpret. Some ancestral features are best shown personally, or else they are not recognized by most observers. These well-known problems with Paleozoic fossil insects are not lost on the advocates of the post-groundplan approach and they have been used as the main reason to ignore important evidence without ever checking published photographs (!) or the presence of homologues in modern insects (!!) and other arthropods (!!!).
Evolution and parsimony gives a very different interpretation. Paleozoic-based features most frequently ignored by the advocates of post-groundplan methods are exites, endites, sutures denoting fusions between “disappeared” podites, abdominal pleural plates, abdominal vesicles, telopodite-like palps, styli and gonostyli, and any other feature showing serial homology of the limb-derived appendages (compare Figs. 1–20). These critics often cite the experiments in Drosophila, suppression and desuppression, and claim that they “disprove” the irrreversibility of ordinal character states. But, geneticists have other explanations for these phenomena and give their support for irreversibility of the old groundplans (Raff et al. 1991; Marshall et al. 1994; Raff 1996). In nature, features do not change when the environment does not change, or when they are useful for some important function. Example: The arch-conservative order Archaeognatha (jumping bristletails) has a cryptic life style and lives in crevasses and litter. From the Devonian to modern times, it has kept its unreduced, telopodite-like palps, which are used in climbing and for balance (Fig. 10.1), articulated epipleuron (Figs. 8, 9), up to three exites (Figs. 8–12) and other Paleozoic character states. Some Hymenoptera (sawflies, Fig. 5) use plesiomorphic multi-segmented palps for raking pollen and retained not only unreduced, Hexapoda-level maxillary telopodites, but also curved double claws (which some Paleozoic insects bore on up to 15 pairs of limb appendages, Fig. 13). Before the Great end-Permian Extinction, most insects probably bore double claws in their palps and abdominal leglets (when present) but these are minute and rarely visible clearly enough in grainy matrix to provide a definitive proof of occurrence.
The supporting evidence for the 11-segmented, serially homologous arthropod ancestral limb (Fig. 1) found in Paleozoic arthropod fossils, is steadily increasing. Developmental genetics made remarkable progress in experiments with HOX genes, homeoboxes, etc., which confirm seriality seen in Paleozoic insects. These paleontological and now also genetic data were ignored in the recent book by Grimaldi and Engel (2005) that strongly advocates the use of the post-groundplan method in interpreting higher-level phylogenies. Those authors explicitly reject two decades in limb/wing contributions: all-arthropod ancestral polyramous limb (Fig. 1), the seriality of mouthparts and endites (Fig. 6), mandible and maxilla formed from a three-segmented coxopodite (rather than from “coxa”, but see Figs. 6, 11, 12), origin of genital appendages and vesicles from endites (see Figs. 15, 20, 21), homology of wings and the limb rami in Archaeognatha from exites (see Figs. 2, 10, 12), and of mandibles and maxillae in Hexapoda from coxopodites (see Fig. 6), etc. Instead, they see all exites and endites as occasionally developing secondary lobes, mandibles and maxilla as a single segment (coxa), etc., exactly as Manton (1977) in “Uniramia”. However, these antiquitated, long rejected—but lately resurrected interpretations without and beyond any reason (except, that they cannot be observed in most (!) living species), are on a collision course with arthropod monophyly, genetics, ontogeny and fossil record. By monophyly, as an iron rule, all arthropod limbs and limb-derived appendages must be derivable from a single, shared ancestor!
The fact remains that, different approaches and turf squabbles notwithstanding, Paleozoic fossil insects are the only concrete witnesses of how the ancestral organ systems really looked like. Clearly, we have no other choice but to cope with the vagaries of fossilization and to make the best of them by seeking confirmation in other fields. The alternative practice of ignoring data in Paleozoic fossils, genetics, ontogeny, and other arthropods, and of looking for evolutionary answers in derived, much younger features, will never bring defendable solutions, now or in the future.
Systematists using the post-groundplan method in higher taxa (e.g., Kristensen 1991, 1995, 1998; Grimaldi and Engel 2005; others) maintain that Paleozoic ancestral states “cannot be observed” in (most) post-groundplan insects and thus there is no positive proof of their existence. This argument is quantitative, mechanistic and invalid. In all Animalia, ancestral characters were reduced in most, but often still occur in some modern species, the same way as in Insecta, Crustacea and Chelicerata. In evolutionary morphology, their published un-reduced occurrences are considered the definitive proof of their existence, and a verifiable plesiomorphy which must not be ignored (see Figs. 5, 6, 8–10, 12, 19–21, and text). The equally important complementary evidence in modern ontogeny, embryology, and genetics published by various authors (see references cited by Kukalová-Peck 1978, 1983, 1998 and Kukalová-Peck and Lawrence 2004) also must not be ignored, because it provides all-important cross-checking and additional invaluable information. As a fact, a fossil ancestral features is considered verified at the very moment it is found still retained in some modern insects, no matter how many or how few the occurrences are (for comparison, pineal eye in vertebrates was retained only in Tuatara!)
Relictual plesiomorphies found in modern insects (Figs. 2–21, text), homologous structures in other arthropods, and compatible data from other biological fields, embryology, ontogeny, genetics, developmental genetics, and experiments with transplants, provide the necessary verification of the oldest, often-unfamiliar fossil character states (see examples below). Using many sources is also highly effective in detecting and rectifying possible interpretive errors, in paleontological material and elsewhere. The groundplan method pools all data for a maximally broad interpretation and broad evolutionary patterns.
Arthropod Limbs: Concepts by the Post-Groundplan and Groundplan Method
“Arthropods Are All Legs”
The groundplan method claims that each arthropod body segment bears a pair of polyramous limbs, used in either walking, jumping, climbing, digging, predation, swimming, respiration, flying, eating, copulation, laying eggs, absorbing moisture, maintaining balance, parachuting, etc. (Fig. 1). Diversification of limbs into all these functions generated a treasure trove of higher groundplan character states, which include many higher synapomorphies. Paleontology and developmental genetics agree that the limb pairs are serially homologous, i.e., that they all evolved from a single, ancestral, polyramous arthropod limb model (Smith 1970, 1988; Kukalová-Peck 1983, 1987, 1991, 1992, 1998; Marshall et al. 1994, Williams and Carroll 1993; Raff et al. 1991; Raff 1996). Such a generalized model is of fundamental importance for higher phylogenetics, because it homologizes the limb-derived appendages in the entire arthropod phylogenetic tree and evaluates them against a monophyletic ancestor. All character states in all appendages are recognized, compared and judged by their distance from this single, shared ancestral model.
The limb/wing model had a deep influence on our understanding of arthropod evolution. The popular limb model of Snodgrass (1935), still used by some entomologists, was based on the highly derived walking/climbing thoracic leg of modern grasshoppers; this has five podites obscured by modifications (Fig. 3.2). Grasshopper leg has only 6–7 visible podites and no outer and inner rami (exites and endites), compared to 11 podites bearing exites and endites in the all-arthropod limb ancestor (Fig. 1). Where did the missing podites go? Epicoxal and subcoxal podites were flattened into pleural plates, which entered the pleural membrane; exites and endites, except thoracic wings, served no purpose and became reduced; since thoracic leg is Z-shaped, podites near leg bends were reduced or fused; tarsus developed small, equal articles for flexibility in climbing, which disguised two podites, basitarsus and (subdivided) eutarsus. Thus impoverished and transformed, grasshopper leg shows only a distant resemblance to the much better preserved maxilla, and none to the mandible, flagellated antennae and cerci, and reduced abdominal styli and gonostyli (Figs. 2, 6, 15, 20). The Snodgrass model also shows no clues to either the origin of “teeth” in the mouthparts, working parts of genitalia, abdominal plate gills, vesicles or styli, or of the wings (Figs. 1–6, 10, 11), and it show all these appendages as “secondary” (and, quite useless for higher classification!!). It is because of this concept, which excludes a shared ancestry, that insect limb-derived appendages, exites and endites are seen as secondary lobes, i.e. not serially homologous but instead uniquely built in all tagmata (rather than derived from a single all-arthropod model and serially homologous). This erroneous concept also does not contribute to hexapod higher phylogeny, since an incomplete ancestor is useless in homologizing and judging character states. Not only this: it also shows the limbs of Hexapoda, Crustacea, Chelicerata and Trilobita as un-homologous, which leads inevitably to the only possible logical conclusion: Arthropoda are a “polyphyletic” phylum as supposed by Manton (1977) (see Kukalová-Peck 1992 as a rebuttal).
Introduction of the Phylum “Uniramia”
When Manton (1977) correctly observed that arthropods eat with their limb base, she used the grasshopper leg (Fig. 3.2) as an arthropod limb model, and interpreted the arthropod mandible incorrectly as a “single” coxal podite (but, it is composed of three podites: Fig. 6). After that she correctly noticed that the most primitive modern insects (Archaeognatha: jumping bristletails) bear mandibles dissected by sutures marking their composition from several limb podites (Figs. 11.1, 12.1). This incorrectly convinced her that unlike Arthropoda, the insects eat with the “tip of their legs” in the same way as Onychophora. Consequently, Manton disassembled the monophyletic phylum Arthropoda, and referred Atelocerata (=Myriapoda + Hexapoda/Insecta) plus Onychophora to a new (but polyphyletic) “uniramous” phylum “Uniramia”. In reality, all arthropods are polyramous and eat with the limb base (coxopodite), which is composed of three limb podites and two endites (Figs. 1, 6); however, only Archaeognatha retained the sutures that separate these three podites (plus a coxal subdivision) and two endites (Figs. 11.1, 12.1). Errors coming from a well-known scientist can have a long half-life. It took 17 years to rectify this massive misconception (Kukalová-Peck 1983, 1992, 1998; Wägele 1993).
Now, by ignoring the need for a monophyletic all-arthropod limb ancestor and multiple evidence for its serial expression, the post-groundplan method is again bringing back the problem with limb homology, in the contributions of Kristensen (1995, 1998), Willmann (1997), and Grimaldi and Engel (2005) (see examples and discussions below). In the book of the last authors, the limbs in Hexapoda are once again defined as “uniramous” and in Crustacea as “biramous”, rather than both being polyramous (Figs. 8, 12.1–4, 15). All rami-based appendages are “secondary lobes”, and the concept of serial homology of limbs, repeatedly confirmed in paleontology, developmental genetics, and classical genetics, is abandoned to a great loss of synapomorphies shared by the higher arthropod taxa. But, the post-groundplan approach, with its emphasis on numbers, brought one bizzar novelty to systematics: Arthropoda are now considered by some as monophyletic in spite of bearing “differently evolved”, “un-homologous” limb appendages in subphyla, classes and orders, because a sufficiently large number of character states in several different character sets shows them as monophyletic (just as if they were artificial, assembled robots!). This reasoning is mechanistic, quite divorced from evolutionary concepts and is faulty to the core. The fact remains that in a morphological system, a monophyletic phylum can never include any organs with non-homologizable character states. In this respect, the dismantling of Arthropoda by Manton (1977) was incorrect but logical, and the recent support for Arthropoda by advocates of the post-groundplan methods is correct but completly illogical!
Mandible and Maxilla: Can They Show Two Different Basal Splits of Pterygota?
Winged insects (Pterygota) are by far the most diversified group of Animalia. For over 60 years of the past century, their basal division was into Paleoptera + Neoptera (Martynov 1923, 1924, 1931; Riek and Kukalová-Peck 1984; Kukalová-Peck 1983, 1985, 1991, 1998; CSIRO 1991; Carpenter 1992; Bechly et al. 2001). Modern Paleoptera include two lineages with wings permanently fixed in an outstretched position, the sister groups mayflies (Ephemeroptera) + dragonflies (Odonatoptera) (Fig. 16). Modern Neoptera include the remaining lineages, which flex their wings backwards at rest (Fig. 19). The groundplan method recorded in the limb/wing structure an unusually large number of differences (65), which confirmed convincingly this basal split of Pterygota into (Paleoptera + Neoptera) (Haas and Kukalová-Peck 2001).
In the post-groundplan approach, this fundamental split of Pterygota was rejected based on an erroneous and outdated belief that mandible and maxilla are composed of a single podite “coxa”, bearing two “secondary” lobes (mola and incisor in mandible, lacinia and galea in maxilla) (but, see Figs. 6, 8.1, 11, 12). This faulty model has many erroneous repercussions; among others it suggests a different phylogeny when applied to the mandible, than when applied to a maxilla.
Kristensen (1991, 1995) compared the mandible of Odonatoptera and Neoptera and found the following five “synapomorphies”: massive, broad mandible; tight ball and socket anterior articulation; sideways shearing movement; an extra strong muscle performing shearing; remaining mandibular musculature reduced; and hypopharynx in posterior position. By plain number of their similarities, these show Pterygota as dividing into (Ephemeroptera + (Odonatoptera + Neoptera)). However, it was long known, that in the next head segment, the maxilla in Odonatoptera bears a fused lacinio-galea, which occurs also in Ephemeroptera and in †Palaeodictyopterida, while the lower Insecta and Neoptera bear individually articulated, separate lacinia and galea (Figs. 6, 10). This shows modern Pterygota as Paleoptera + Neoptera, and modern Paleoptera as Odonatoptera + Ephemeroptera.
With the post-groundplan approach (relying on numbers), Kristensen (1991 and before) found five mandibular characters more convincing that a single fusion in the “possibly secondary” maxillary lobes. Consequently, division Paleoptera was rejected as polyphyletic and Pterygota were newly supported as (Ephemeroptera + (Odonatoptera + Neoptera)). Some systematists (most recently, Grimaldi and Engel 2005) accepted this emendation, in spite of limited evidence. It was also confirmed by some molecular analyses (Wheeler et al. 2000), while other analyses supported Pterygota = (Paleoptera + Neoptera) (Hovmöller et al. 2002) or (Odonatoptera + (Ephemeroptera + Neoptera)) (Kjer 2004). Unfortunately, the new division of Pterygota has found its way into some important entomology textbooks, presumably because of using a “better” systematic method!! However, the most significant repercussion concerned genetics: Is it likely, at all, that different parts of the mouthparts (or other co-evolved organs) could retain an allegiance to two different higher taxa? If confirmed, the implication would seriously affect many evolutionary concepts. The groundplan method, which uses evolution, offers the following answers.
Mandible (Fig. 11)
Insect mandibles show progressive early adaptation toward a more forceful bite. In primitive Monocondylia: Archaeognatha, the mandible is a narrow, slanted plate with a single permanent posterior articulation, mainly used for milling soft food (Kukalová-Peck 1987, 1998, Fig. 19.1b–d). In early Dicondylia, the mandible becomes broader and stronger by extending anteriorly and creating a secondary anterior condyle with the clypeo-tentorium. This condyle is shaped like an oval socket in †Cercopoda (a personal observation by JKP); in silverfish, like a paired sockets; and, it can be elongated to a sliding groove (Fig. 11). The early mandible pressed and slid against a shaft on the clypeo-tentorium (Figs. 6, 11). The contact could be disconnected at will. A long mandibular slide was present in †Palaeodictyopterida (Fig. 11.3) and a short slide is present in modern Ephemeroptera juveniles (Fig. 11.4) and in fossil juveniles and adults (Kukalová-Peck 1985, Fig. 34a, b). A permanent anterior condyle, based on a single tightly fitting socket, is a derived condition occurring twice, in Neoptera and again in Odonata (Fig. 11.5, 6) (Smith 1988; Kukalová-Peck 1985, 1987, 1991, 1992, 1998, Fig. 19.1e).
Basal Neoptera very probably fed on plants, which were hard to chew and required a more forceful bite. Hence, the dicondylous jaw became adapted as follows: the mandibular anterior articulation became tight and permanent; the mandible became broader and more massive; the mandibles opened widely sidewise on two tight pivots like a door, for a shearing function; they were manipulated by a single, strong muscle; the remaining non-shearing muscles became reduced; and the shearing mandible pushed the hypopharynx into a new, more posterior position (five autapomorphies). This adaptation possibly took place very early, for the first time already in the Ordovician or Silurian when Pterygota split into two divisions: Paleoptera + Neoptera. It happened for the second time probably in the Late Silurian or Devonian when paleopterous Odonatoptera became adapted for predation, by adding exactly the same adaptations to their mandibles. Predation also requires a more forceful bite and the adaptations probably happened back-to-back.
The above scenario shows the occurrence of powerful shearing jaws in Neoptera as a primary adaptation of the mouthpart organ system, and in Odonatoptera as a convergent adaptation derived from the same or very similar ancestral state.
Maxilla (Fig. 10)
The groundplan method brought evidence that the insect mandible and maxilla are both coxopodites, i.e., they are composed not of one, but of three originally independent, articulated podites, and of two independent, articulated endites (Figs. 6, 10). Endites, or inner rami, are ancient articulated and muscled lobes present in Precambrian arthropod ancestors and shared by all subphyla (Fig. 1). Thus, they are older than the higher taxa Hexapoda and Insecta, which probably arose some time between the Early Cambrian and Early Ordovician. Two decades of crosschecking confirmed that groundplan-level fusions of rami in arthropod higher taxa do not unfuse. In most primitive modern insects (Archaeognatha), the maxilla bears two separate, articulated endites, the lacinia and galea (a plesiomorphy) (Fig. 8) (Kukalová-Peck 1998, Fig. 19.1b–d). In Neoptera, the lacinia and galea are also separate (a plesiomorphy) (Fig. 10). However, all Paleoptera (Odonatoptera + Ephemeroptera + †Palaeodictyopterida) share a fused lacinio-galea (a synapomorphy) (Fig. 10). This shows the lacinio-galea as a high-ranking, unique synapomorphy supporting the basal split of Pterygota into Paleoptera + Neoptera.
Genetic Interpretation
Genetically, hexapod mouthparts have functioned as a tightly co-evolved organ for about 500 million years. Therefore, they can be neither considered, nor treated as an assemblage of independently evolving appendages! In no way can a mandible be involved in a different phylogeny than a maxilla (Marshall et al. 1994; Raff et al. 1991; Raff 1996). Therefore, the mandible can never support Odonata + Neoptera, and the maxilla Odonatoptera + Ephemeroptera, as anticipated by Kristensen (1991, 1995).
Raw Numbers of Similar Character States in Higher Systematics
In modern species and genera, higher numbers (frequency) of similarities occurring in several different character sets are diligently pursued, ad they often denote true synapomorphies. However, in orders, lineages, divisions and classes, raw numbers of post-groundplan similarities are irrelevant, have no phylogenetic meaning and are even suspicious for not representing informative states. This is because homoplasies (=false synapomorphies) and parallelisms are much more frequent than apparent synapomorphies. In any case, the perilous “frequency rule” does not apply and its following produces falsely reconstructed phylogenies.
Presence or Absence of Subimagos in Ancestral Pterygotes?
In the post-groundplan approach, the subimago is supposed to occur “only” in Ephemeroptera and “not” in Odonatoptera + Neoptera, or in †Palaeodictyopterida (Kristensen 1991, 1995; Wheeler et al. 2000; Grimaldi and Engel 2005). This erroneous character interpretation has been repeatedly falsified in at least 12 publications: standard textbooks (CSIRO 1991), Treatise on fossil insects by Carpenter (1992), Sharov (1966), Carpenter and Richardson (1968, 1971), Kukalová-Peck (1974, 1978, 1983, 1985, 1991), and Shear and Kukalová-Peck (1990). All these studies show that Paleozoic ancestral pterygotes initially had several subimaginal instars, recorded so far in Ephemeroptera, †Palaeodictyoptera, †Megasecoptera, fossil Odonatoptera, and in the †ancestral plecopteroids and hemipteroids (see Kukalová-Peck 1991 for a review). This indicates that pterygote development was initially completely gradual as in their extant sister group Zygentoma (silverfish). Therefore, the metamorphic instar, which is now present in all modern lineages, originated repeatedly several times in parallel, possibly mainly during the Great end-Permian Extinction, as a response to a very harsh long-term environment. The presence of a subimago in living Ephemeroptera is definitely not an autapomorphy but a remnant of a once more common development.
Hexapod Climbing Tarsus, Judged by the Post-Groundplan and Groundplan Method
The hexapod climbing tarsus (Figs. 1–7, 9, 10, 13, 16) is one of several pivotal adaptations that turned insects into the most successful animal group. Willmann (1997) rejected the homologized hexapod limb podites (Kukalová-Peck 1983, 1991) (Fig. 1) and emended interpretation of the tarsus as follows: hexapod tarsus is primitively “one- segmented” and its subdivision in insects into up to five articles is derived. (But, this change disrupts homologization of BT and ET, present in palps, leglets, gonostyli and in the plesiomorphic limbs of other arthropods!!). The evidence for Willmann’s emendation was that the most primitive extant insects Archaeognatha (jumping bristletails) share with extant Crustacea a “one-segmented” tarsus (but, see Figs. 7, 8) as a symplesiomorphy, hence, all tarsal articles in Hexapoda and Insecta must be secondary subdivisions.
This emendation based solely on derived states turns tarsal interpretations up side down. It would interpret the original three-jointed tarsi in Paleozoic ancestral nymphs and adults as derived, and the one-segmented fused tarsi in (only) some modern aquatic insect nymphs as plesiomorphic. The arthropod groundplan level two-segmented tarsi in Trilobita and fossil Crustacea would also be derived, while the one-segmented fused tarsi in many modern Crustacea would be plesiomorphic, etc. The groundplan method broadly documents an evolutionary scenario on fossils, and offers more reliable data for phylogenetic reconstructions than those based on derived modern characters. Before all, primitive extant groups definitely offer no guarantees what-so-ever that all of their character states must be plesiomorphic!! (Hennig, 1969, 1981).
Varied Numbers of Arthropod Tarsomeres
In Paleozoic Trilobita, Cambrian Crustacea and Chelicerata, the tibia is followed by three fully articulated and muscled podites: basitarsus, eutarsus, pretarsus (Fig. 1) (Kukalová-Peck 1983, 1987, 1991, 1998; Maas et al. 2003). In the Hexapoda groundplan, eutarsus became once subdivided in the limbs on all tagmata, so that the tibia is followed by: basitarsus (BT), eutarsus 1 (ET1), eutarsus 2 (ET2), and pretarsus (PT). The resulting tarsal flexibillity is the groundplan adaptation of Hexapoda for climbing, living, and actively escaping from predators on vegetation (fusion of tarsal joints is presumed derived) (Figs. 1, 2, 3.1, 4–8, 15, 18.1, 20). Evidence in fossils: Flexible, once subsegmented climbing tarsus plus double-clawed pretarsus occurs on up to 15 limb pairs of Paleozoic insects: in two pairs of palps, three pairs of thoracic legs, eight pairs of abdominal leglets, one pair of gonostyli, and one pair of cercopods (the latter, only in †Cercopoda, Fig. 13) (Kukalová-Peck 1983, 1987, 1998) (Figs. 1–21). Evidence in extant insects: Hexapod climbing tarsus without claws is retained in the maxillary palps of modern Archaeognatha (Figs. 7, 8); with claws, it occurs in Hymenoptera, in the sawfly Pleroneura sp. (Fig. 5; the fused-immobile claws in palps rake pollen), and in the “walking pupa” of Raphidioptera (E. L. Smith, personal communication). The serial occurrence of ET1 and ET2 in all oldest telopodites strongly indicates that all hexapodan limb telopodites originally had once subdivided eutarsus (=3 tarsal joints). Further eutarsal subdivisions and their fluctuations are limited only to the thoracic legs involved in climbing and walking (Figs. 2–5). Outside the thorax, tarsi in the stem-species that survived the Great end-Permian Extinction are almost always reduced, many times independently and in parallel. Many Paleozoic insects show the presence of 3 tarsal joints (tarsomeres).
Secondary Fusions Between Tarsomeres
These occur in legs of Arthropoda walking on unconsolidated substrates (soil, litter, or the bottom of water bodies). In these, the tarsal podites become aligned, perpendicularly oriented, and secondarily fused, in order to sink and then push laterally against soft sediment as a lever (Figs. 8, 13). This type of fusion took place repeatedly and independently many times, in Crustacea, Chelicerata, the (secondarily) aquatic nymphs of most Ephemerida, and in the cryptic, litter inhabiting insects, †Monura (Fig. 7) and Archaeognatha (jumping bristletails) (Fig. 8). These two most primitive insect orders (Monocondylia) diverged very early from the free-living insect stock by adapting to a cryptic lifestyle, probably at the bottom of low-growing, Silurian plants rooted in mud and water, or even earlier, in algae on shores of Ordovician water bodies. As an adaptation to confined spaces and soft substrates, the early protowing appendages became lost and the tarsal articles gradually aligned (compare Figs. 7 and 8); at the same time, the largely predator-free environment helped to sustain many early insect plesiomorphies. Evidence: In Permian †Monura (Fig. 7) (=the sister order to Archaeognatha) as well as in Carboniferous Zygentoma (Kukalová-Peck 1987, Figs. 10, 15) the thoracic legs still bear articulated and somewhat mutually flexible ET1 and ET2, as in the basal hexapod limb (Kukalová-Peck 1998, Fig. 19.5d). But, in extant Archaeognatha (Fig. 8), the same eutarsal subsegments are fused and perpendicularly oriented (i.e., derived, an autapomorphy), while the basitarsus (BT) is anteriorly disconnected and scale-like (like in †Monura only longer; a synapomorphy).
The evidence above shows that in Hexapoda, the flexible climbing tarsus is plesiomorphic, tarsal fusions and perpendicular position are derived, and further subsegmentations of eutarsus occurring only in thoracic legs, are also derived.
Twin Protowings: The First One Reconstructed—The Second One Found in Fossils
Insect wings in Pterygota are their most diversified organ system, distinctive enough to be used to identify pterygote divisions, lineages, orders and even many families in both extant and extinct representatives (Carpenter 1992; Wootton et al. 1998; Wootton and Kukalová-Peck 2000). Therefore, a flawless protowing model is crucial for anchoring most pterygote higher phylogenies. It serves to identify higher groundplan character states in modern species and in the process, to open access to objectively identified synapomorphies shared by pterygote sister groups in suborders, orders, lineages and divisions (Figs. 7, 8, 15–19).
In order to document the ancestral pterygote protowing, this author studied and figured all Paleozoic, some Triassic and some Jurassic insects in the museums of Europe, Russia, America, Australia, S. Africa, and China. A large collection of Early Permian insects was excavated for 12 years at Obora, Moravia, Czech Republic. The study of modern wings was focused mainly on basal families, under the auspices of foremost modern specialists. An extensive morphological collection of living insects was assembled, mainly in Central and South America, South Africa and North Australia (see “Material” and “Acknowledgements” sections).
This gathering of data spanned over two decades and provided the objective knowledge of concrete steps in which the transformations of wing veinal sectors advanced in pterygote lineages from the Paleozoic to modern times. It also showed which particular combinations of irreversible fusions, braces and reductions define each modern higher taxon (divisions, lineages, superorders, orders) in their living representatives. Valid systematic rules were discovered, showing that in the groundplan characters of all higher taxa, the fusions and reductions always advanced in one predictable direction: from separate to fused, from unbraced to braced, and from unreduced to reduced.
This author reconstituted the ancestral protowing model in 1983 by recording all the least fused, braced and reduced wing character states occurring in pterygote lineages, in extinct and extant species. Reconstructed protowing (Fig. 17) contained eight veins, each vein composed of two dichotomously branched veinal sectors starting independently from a single blood sinus (basivenale). Fusions of sectors into veinal stems, braces and fusion braces were completely absent (an important plesiomorphy). Three previously unknown veinal sectors were discovered and added to the veinal model: PC-, CP-, and ScA+. These identified the “extra” “un-homologous” veins near the anterior wing margin, occurring in modern Ephemeroptera, Hemineoptera, and Orthoneoptera, as plesiomorphic. The ancestral model of Paleoptera and Neoptera articulation was first offered in 1983, and again in 1997. Neoptera were updated by JKP in the paper by Haas and Kukalová-Peck (2001). Anojugal area in wings was further developed in the paper by Kukalová-Peck and Lawrence (2004). Wing base in Paleoptera, involving all extant families of Ephemeroptera (co-authored by J. G. Peters and T. Soldán) was recently upgraded and homologized in every detail with the sister group Odonatoptera, but the results are not published.
Against all expectation, 12 years after publishing (in 1983) the pterygote protowing model based on broadly researched character transformation series, I recognized the same protowing veinal system as present in the prothoracic winglets of †Geroptera, the most primitive Carboniferous dragonflies (Figs. 16, 17.2) (Wootton et al. 1998; Wootton and Kukalová-Peck 2000). This similarity was a maximally encouraging bonus showing the objectivity of broadly documented evolutionary models. Plesiomorphic protowing—like prothoracic winglets were attached to the prothorax in front of two pairs of highly derived, dragonfly-like flying wings. Alerted to this opportunity, I found yet another protowing veinal system in the prothoracic winglets of †Palaeodictyoptera (Late Carboniferous of Commentry, France, Fig. 17.1), in front of two pairs of very differently derived flying wings! These I published twice before (in 1970 and 1978) in a routine description and never suspected of drawing a protowing venational model. It took an additional 20 years of comparative experience to recognize this veinal pattern as an extremely rare relic from a very distant past, not expected to be ever found. The fossiliferous Early Paleozoic freshwater deposits have been lost worldwide to erosion. This loss makes prothoracic “protowings” of Carboniferous insects’ even more remarkable monument to evolution, available only in fossil record.
Thus, the protowing venation based on indirect but extremely broad evidence published more than a decade ago (in 1983) turned out to be identical to the actual protowings retained in two distantly related Carboniferous lineages with very different flying wings. This is about the most objective proof one can get showing that an ancestor of a morphological organ system can indeed be reliably reconstructed based on a very broad comparative study of character transformation series shown in fossil and modern specimens. It also gives the previously unavailable direct testimony against charges (from post-groundplan side) that the ancestral models composed of the groundplan—level character states are by definition “subjective” and thus “inferior” to post-Hennigian reconstructed phylogenies, based exclusively on “directly observed” post-groundplan states. But, just the very opposite is true: it is the exclusive use of post-groundplan character states, which proved to be inferior and un-informative, and which caused the present crisis of morphological systematics in insect (and arthropod) higher taxa.
Ancestral Protowing: Its Use in Reconstructing Pterygote Higher-Level Phylogenies
One of the advantages in the groundplan method is that the search for groundplans with distinct synapomorphies can be done independently and with different insect collections; yet at the end, researchers will agree which character states are at the groundplan level and which represent synapomorphies. This is because all characters are judged by their distance from the same protowing model and are evaluated by the same irreversibility rule (see “Groundplan Method” above). This author and co-authors worked on different continents, yet obtained exactly the same synapomorphies and sister groups!!.
Cladistic and Molecular Analysis
After the higher groundplan characters are identified in living species (but not before), they can be subjected to the cladistic analysis and processed by computerized methods (see analysis offered by Kukalová-Peck and Lawrence 2004). This step is arbitrary, and it does not change the final outcome. Unlike in lower taxa, groundplan method judges characters before they are analyzed, hence the higher sister groups stand out clearly before their character states are cladistically analysed and processed. But, cladistic analysis is offered anyway by this author and co-authors, for its crisp and logical arrangement of character states. In contrast, “molecular analysis is not cladistic analysis” (Schwartz and Maresca 2006). Evolutionary morphological analysis of the organ systems and molecular analysis are two independent biological fields with their own evidence, problems and solutions. The results from biological fields greatly benefit from being compared, crosschecked, and mutually verified, complemented, and then engaged in one comprehensive interpretive concept (see below). However, to reach this benefit, their character states must never be thrown together into one accidental heap and then judged according to larger numbers of superficial similarities!! Such mechanistic, non-evolutionary approach guarantees in higher taxa production of unrealistic, conflicting, and not provable higher phylogenies.
Phylogenies Reconstructed by the Post-Groundplan Method
The post-groundplan method typically produces two or more reconstructed phylogenies of the higher taxa, which are left unresolved (e.g., as offered in contributions by Kristensen 1991, 1995, 1998). In the last decade, sometimes in combination with molecular analysis, this method offered the following “possible” phylogenies: In Pterygota, three basal splits, into (i) (Ephemeroptera + (Odonatoptera + Neoptera)); (ii) (Odonatoptera + (Ephemeroptera + Neoptera)); (iii) (Paleoptera + Neoptera). In Neoptera lineages, three possible combinations, (i) [(plecopteroids) + ((orthopteroids + blattoids) + (hemipteroids + endopterygotes))], (ii) [(plecopteroids + orthopteroids + blattoids) unresolved + (hemipteroids + endopterygotes)], (iii) (plecopteroids + (orthopteroids + (blattoids + (hemipteroids + endopterygotes)))). In Endopterygota, two possible combinations: (i) [(Coleoptera + Neuropterida) + (Mecopterida + Hymenoptera)], (ii) (Coleoptera + (Neuropterida + (Mecopterida + Hymenoptera))). In modern literature, unresolved or multiple phylogenies between orders is a norm. Examples: Zoraptera and Strepsiptera are referred simultaneously to up to three different superorders or lineages (Kristensen 1991, 1995, 1998; Beutel and Pohl 2006; Grimaldi and Engel 2005).
Phylogenies Reconstructed by the Groundplan Method
The groundplan method (applied to the limb/wing organ system, see examples in Figs. 1–21) uses only one ancestor and one set of irreversibility rules for all higher taxa in Arthropoda. Therefore, it can produce only one higher phylogeny (right or wrong). For winged insects it is: Pterygota = Paleoptera + Neoptera; Paleoptera = (Ephemeroptera + Odonatoptera); Neoptera = (Pleconeoptera + Orthoneoptera) + (Blattoneoptera + (Hemineoptera + Endoneoptera)); Endoneoptera = (Hymenoptera) + (Coleopterida + (Neuropterida + Mecopterida)). (But, note that Paleoptera and Neoptera differ in an unprecedented 65 wing-based character states). Zoraptera share seven synapomorphies with other Blattoneoptera. Strepsiptera and their sister Coleoptera (under the superorder Coleopterida) share nine unique, highly complex wing synapomorphies, which do not occur elsewhere in Pterygota (see character analysis by Haas and Kukalová-Peck 2001; Kukalová-Peck 1991, 1992, 1998; Kukalová-Peck and Lawrence 2004). Unfortunately, documentation of the evolutionary background is way too voluminous to review here. Interested readers are recommended to see the above references.
Origin of Insect Wings: Different Views
Insect wings are by far the most richly diversified organ system in insect higher and intermediate taxa. They also include easily detectable synapomorphies clearly identifying pterygote sister groups. Yet, exploitation of these desirable attributes in higher phylogenetic reconstructions requires knowledge of their evolutionary history. This includes: the homologue of wing and wing articulation in other Arthropoda and its basal attributes; serial homologues of wings and wing articulation in head, thorax and abdomen; reconstruction of the shared ancestral protowing model of veinal system and the wing articulation; the record of concrete wing character transformation series in all pterygote lineages, from the Paleozoic to modern times; a feasible hypothesis of the origin of insect flight; and, the evolution of the form and function of wing pairs, wing coupling, fusions between basivenalia and wing sclerites, and other modifications in response to different roles of wings in flight.
The Post-Groundplan Method
In limb/wing structure, this approach based on modern, diversely derived structures revived the long invalidated paranotal theory of wing origin! (e.g., by Grimaldi and Engel 2005). This theory derives insect wings from the lateral extensions of the terga and interprets wing articular sclerites (pteralia) as tergal fragments along the base of these extensions. The paranotal theory was based on the facts that: (1) the wing pads of modern pterygote nymphs are fused to terga; (2) pterygote sister group silverfish (Zygentoma) bears thoracic sidelobes which also resemble tergal extensions; and (3) in human experiments, short, solid tergal extensions enabled gliding through the air, considered a “primitive” kind of flight. Paranotal theory of wing origin was widely accepted earlier in the past century, but was later disproved by multiple contradicting evidence found in organ transplants, genetics, developmental genetics, embryology, ontogeny, and paleontology (see a full review below). It inevitably projects strong phylogenetic implications, which were followed by Grimaldi and Engel (2005). Therefore, these authors, again, interpreted the permanently outstretched wings in modern paleopterous insects, mayflies and dragonflies (superficially resembling tergal extensions) as “plesiomorphic for Pterygota”, and their wing articulation including large plates as the “plesiomorphic type of wing articulation”. Hence, the wings of Neoptera, which can be flexed backwards, were considered as secondarily capable of this movement, and derived. Under this long rejected concept, the authors were able to justify the basal split of Pterygota as: (Ephemeroptera) + (Odonatoptera + Neoptera) preferred by Kristensen (1991 and before) and based on the post-groundplan method. However, the authors were unable to explain why the acknowledged most primitive order of Paleoptera and Pterygota, the †Diaphanopterodea was able to flex their wings backward, by using a paleopterous (i.e., plesiomorphic in their emended sense) wing articulation (Fig. 18). The authors explained this unreconcillable conflict in evidence by giving up and coining this ancient, richly represented order as “enigmatic”. As expected, resurrected but erroneous paranotal theory once again generated undefendable basal divergencies of Pterygota, discussed here in the previous section.
The Groundplan Method
This offers the following evidence: Paleontology: In the plesiomorphic Paleozoic juveniles of Paleoptera and Neoptera, the winglets are fully mobile and articulated in a functional (lateral) position, as in miniature adults (Figs. 2, 14). Several thousands of detached nymphal winglets of Ephemeroptera from the Early Permian from Elmo, Kansas, are deposited at the Museum of Comparative Zoology, Harvard University, Cambridge (modern nymphal wing pads stay always fused to terga). About 200 Paleozoic juveniles with fully articulated, mobile wings are recorded (Fig. 14). Note that fusions of the winglets to terga first started in young instars, and already in the Carboniferous, while older instars still retained articulated wings (most noticeably in some Palaeodictyoptera). The wings increased in size very gradually, with several subimagos preceding the imago. A metamorphic instar was not found. In Paleoptera, the most primitive order †Diaphanopterodea (Fig. 18) retained the near-plesiomorphic state of the pterygote articulation, which is almost identical to the one reconstructed for the all-pterygote shared protowing model (compare Figs. 18 and 19) (Kukalová-Peck 1978, 1983, 1991, 1992; Kukalová-Peck and Brauckmann 1990; Carpenter 1992). This was later variously modified in the rest of Paleoptera, and again but quite differently in Neoptera (Fig. 19).
Genetics and Ontogeny
In modern insect larvae, the wing disc separates from the limb Anlage just above and slightly posteriorly of the spiracle. A small wing appendage evaginates from the Anlage and becomes larger and broader in each subsequent instar. The tergum gradually expands to meet the wing appendage. Their eventual fusion takes place in some older instar. This shows that fusion between the wing pad and the tergum is secondary (Tower 1903; Garcia-Beillido 1975; Kingsolver and Koehl 1994; Paganiban et al. 1995; Popadic et al. 1998; Raff 1996; Abouheif and Wray 2002).
Ontogeny
In modern libellulid Odonata, evaginated wing appendages is free until as late as in the 8th instar (!), when a secondary fusion occurs between the wing pad and the tergum (Bocharova-Messner 1959; for figures and results in this important but difficult to find paper see JKP 1978).
Wing Anlage Transplants
The wing articulation is part of the wing. A transplanted wing Anlage develops into the wing bud plus all pteralia including wing processes (!) This provides a very important confirmation that none of the wing articular sclerite separated from the tergum, not even so called “tergal” processes (renamed “wing processes” by this author) (Stenzhorn 1974).
Developmental Genetics and Paleontology
As shown in experiments, insect wings in Crustacea are homologous to the articulated upper gills of the fairy shrimp Artemia (=epicoxal exites) (Averof and Cohen 1997) (Fig. 1).
Interpretation of Wing Origin
All arthropod/insect limb appendages, including wings, are serially homologous in the head, thorax and abdomen; they evolved from a single, shared limb model (Sharov 1957, 1966; Norling 1982; Kukalová-Peck 1983, 1985, 1991, 1998; Brodsky 1994; Kingsolver and Koehl 1994; Shubin et al. 1997).
Interpretation of Wing Ontogeny
In modern insect juveniles, the wing separates from the limb Anlage in the pleural membrane, far from the tergum. It evaginates looks and behaves like an appendage. It’s flattening and fusion to the tergum occurs only later in development, which shows it as secondary. All wing articular sclerites are part of the limb/wing organ system, since none of them separates from the tergum. In body segments, they are at exactly the same position and level as the epicoxal pleuron still retained in plesiomorphic †Monura (Fig. 7) and extant related Archaeognatha (Figs. 8, 9) (i.e., articulated between the tergum and the subcoxal pleuron) (Kukalová-Peck 1998, Figs. 19.3e, 19.5a). In the Hexapoda head, the epicoxal pleuron is fused to the tergum, perhaps originally as small sidelobes (still present above maxilla in some Zygentoma, silverfish: Kukalová-Peck 1987, Fig. 17); in Diplura (the sister of Insecta), two epicoxal, double-walled sidelobes (of mandibles and maxillae) are projecting from the head capsule and fused ventrally with the labium, thus partly enclosing the mouthparts (Kukalová-Peck 1987, p. 2336). In almost all Paleozoic insects, abdominal epicoxal pleura are separated from the terga by sutures (Fig. 15). They form narrow sidelobes, either alone (in Ephemeroptera) or combined with abdominal winglets (in Palaeodictyopteroida and possibly in all Neoptera). In the polyramous limbs of Trilobita (Kukalová-Peck 1991, Fig. 6.1A), the epicoxal pleuron is a plate articulated to the tergum, which serves as an articulation site for 10 articulated, cylindrical limb podites starting with subcoxa (Fig. 1). In Pterygota, thoracic epicoxal pleuron is also articulated to the tergum (at its both sides, as in Archaeognatha and Pterygota), but in Pterygota it became fragmented around muscular attachments and the fragments function as wing articular sclerites manipulating the wing (Fig. 17).
Insect wings are homologous with the outer rami (=exites) of arthropod epicoxal pleura (Fig. 17.1–2). The flattened protowing exite travelled dorsally (upward) on its epicoxal pleuron, which fused completely underneath it and above the ventral wing process (the second axillary is doubled, Fig. 17). Note that a similar upward shift and fusion occurs in the coxal exite of modern Archaeognatha and other arthropods. This evaginated in the coxo-trochanteral membrane and travelled to its present position in the middle of the coxa (Figs. 8.3, 10.1, 12.2). As a result, pterygote protowing exite is completely surrounded by the epicoxal pleuron and rests centro-ventrally on a jutting projection from the subcoxal pleuron (the ventral wing process) (Fig. 17.3). After the epicoxal pleuron and the protowing base fragmented around their muscular insertions, the fragments formed the dorsal and ventral articular sclerites (pteralia). Dorsal pteralia were arranged proximo-distally into eight rows (each resting on top of the blood supplying channels and aligned with eight wing veins), and antero-posteriorly into three columns, composed of epicoxal pleuron subdivided into two parts (like thoracic subcoxa) and the base of protowing exite; all three columns bear muscular insertions (Figs. 18, 19). Ventral pteralia formed two clusters, the basalare and subalare, which flanked the ventral wing process and supported the wing (Fig. 17.3). As a result, the advanced protowing rested on a wall composed of three groups of pleural pivots: the basalare, the ventral wing process, and the subalare (Fig. 17.3). Since the muscles cannot pull across the wall, the veinal basivenalia (=sclerotized blood sinuses of the wing veins) plus the entire veinal system in the wing blade completely lack musculature. Movement of the wing is generated by limb and thorax musculature, to which the basivenalia and the veinal system (through its fluting, fusions, braces, forks, bullae, breaks, joints, bends, flexion lines, folds, fields, sclerotized areas, etc.) respond by a highly efficient cascading series of movements. As a result, a single rectangle in a dragonfly wing exerts micro-engineering forces that prevent the posterior wing margin from fluttering and shredding, as the edge of a flag (Wootton et al. 1998), and earwig wings execute astonishingly complex triple hind wing folding (Haas in: Haas and Kukalová-Peck 2001).
In †Diaphanopterodea (Fig. 18), the most primitive order of Pterygota and Paleoptera, almost all rows and columns of sclerites are in a state researched as the ancestral protowing state shared by all Pterygota. The only (and quite minor) derived fusion is between the precostal and costal row of sclerites (a synapomorphy of Palaeodictyopterida) (Kukalová-Peck 1991, Fig. 6.10F). Because of their plesiomorphic articulation, the wings of †Diaphanopterodea were able to move in all directions as in other arthropod exites, including backwards and into a roof-like position (Kukalová-Peck and Brauckmann 1990). This plesiomorphic mobility was also retained in Neoptera (a symplesiomorphy) (Fig. 19), but on top of it their pteralia became adapted to lock the wings securely and tightly in the flexed position, very probably to assist in hiding from predators in the very dense and low-growing, swampy Silurian vegetation (Kukalová-Peck 1983, 1998; Brodsky 1994; Kukalová-Peck and Lawrence 2004).
The Evidence for Derived Paleoptery
During flight modern basal Neoptera must hold their wings in an outstretched position by a muscular pull on the basalare, which is connected with the humeral plate and the anterior wing margin (Fig. 19). To eliminate an energy-consuming pull, various devices evolved in derived neopterous groups, locking the wing pairs together, etc. In Paleoptera, the aligned parallel arrangement of wing sclerites and basivenalia (Fig. 18.2–3) allowed yet another solution: secondary fusions between basivenalia on the wing side of the pleural wall, and sclerites on the thoracic side of it. These elongated the wing base across the wall of pleural pivots into the thorax, so that it can rest on them and rock like a seesaw (Figs. 16, 17). Note that in Ephemeroptera + Odonatoptera shared fusions in articular plates and shared fusions and braces in the veinal system are very different from those in †Palaeodictyopterida, and indicate two independent origins from a †Diaphanopterodea-like ancestor (Kukalová-Peck 1992, 1998). In Paleoptera, the wings equipped by a rocking wing base (e.g., in extant Odonatoptera + Ephemeroptera) are permanently in a “ready-to-fly” position: this provides an efficient, energy saving device, which is variously composed in individual orders, or absent (in †Diaphanopterodea) and thus quite clearly and consistently derived. In the absence of aerial predators such as flying dinosaurs, birds, and bats, the wings of Paleozoic Ephemeroptera, Odonatoptera, and †Palaeodictyoptera grew to gigantic sizes (44, 71, and 56 cm in span, respectively) (Fig. 16). In contrast, Paleozoic †Diaphanopterodea and Neoptera (Figs. 17, 18), which lacked these energy saving rocking plates, remained medium-sized or small. Extant Neoptera often reach larger sizes than their Paleozoic predecessors. Fully homologized articular sclerites harbour many higher-level synapomorphies (Kukalová-Peck and Lawrence 2004), while wrongly homologized sclerites offer none. Instead, they invite speculation leading to unrealistic phylogenies (see examples above).
Wing Support for the Sister Groups Odonatoptera + Ephemeroptera
As a practical demonstration of the groundplan method, this relationship (in dispute since about 1991, after being rejected by Kristensen and other authors using the post-groundplan method) is shown below. The wing characters are judged against a single protowing ancestor.
All modern and Paleozoic mayflies and dragonflies share the following synapomorphies (revealed by the groundplan method): fusions between six basivenalia on the wing side and six sclerites on the thorax side forming a rocking wing base; a unique anterior articular plate of four precostal and costal sclerites; a unique anal brace (three different veinal sections, aligned into a protruding arch; a derived, recurrent ScA forming a strong, posteriorly bent subcostal brace; radial sectors basally close or touching in a double barrel position (not superimposed as in Neoptera); two always fused veinal stems, M and Cu; four shared braces (cross-vein braces or fusions) near wing base (a total of 21 superordinal synapomorphies around the wing base). In the Neoptera groundplan, none of these characters is present (Kukalová-Peck 1983, 1985, 1991, 1998; Riek and Kukalová-Peck 1984; Haas and Kukalová-Peck 2001; JKP et al. manuscript in progress; Kukalová-Peck and Lawrence 2004).
Origin of Insect Flight: Different Views by Competing Methods
The Post-Groundplan Method
A paranotal theory deriving wings from “lateral tergal extensions” postulates, that flight developed probably with the advent of trees in the Late Devonian. It proposes that pterygote ancestors climbed the trees or rocks and glided down on their extended lateral tergal lobes. As shown in many human experiments, gliding improves with larger wing size, while a narrower and twistable base considerably improves manoeverability and controlled landing. This shows that the wing base adapted toward better flight by becoming narrower and by fragmenting basally into sclerites, which acquired muscular attachments. Twisting of the wing base followed, and protowings thus equipped, gradually became able to turn gliding into a powered flapping flight. However, this scenario is seriously flawed. Only human engineer premeditates a process, and then secures its execution. Nature is quite thoughtless and follows consistently only one principle: survival of the fittest.
Discrepancies
Combined data from fossils and biological fields (reviewed above) show protowings as small, articulated and flattened appendages mobilized by two sets of limb muscles, and thus quite incapable of gliding! In nature, gliding is basically a slowed-down fall and a dead-end adaptation, not a “natural” (=easiest) doorway to flapping flight. Many groups of animals can glide: fish, frogs, lizards, snakes, dinosaurs, opossums, squirrels, monkeys, and even humans. But, we know that with time these gliders only become better gliders. All animals capable of powered flapping flight (flying dinosaurs, birds, bats, and insects) built their wings from previously articulated and muscled limb-derived appendages! This is because powered aerial flapping flight requires a massive preadaptation (which probably could not have originated de novo): in insect wings, a large aerodynamic wing blade, thinned membrane, a sophisticated veinal support, a set of perfectly orchestrated articular sclerites, and a complex muscular apparatus from limbs and thorax promoting a wing movement following a horizontally oriented number “8”. During this intricate preadaptation, the wings would be constantly useless for aerial flight. While human engineer uses foresight, there is never a future goal to aim for in nature, just satisfying a momentary need for survival. Hence, the right question to ask is what the protowings were doing before they were ready for the complex flapping movement necessary to generate powered aerial flight? It was something quite useful for survival, or they would be either reduced (as in jumping bristletails, †Monura and Archaeognatha), or absorbed into tergal sidelobes (as in †Cercopoda, Zygentoma (silverfish) and in the prothoraces and abdomens of most Pterygota) (Figs. 2, 7–9, 13).
The Groundplan Method
Combined evidence shows that insect protowings originated from articulated and mobile arthropod epicoxal exites. Exites occur in the Precambrian limb groundplan, i.e., are significantly older than Hexapoda, Insecta, or Archaeognatha. Therefore, the loss of protowing exites, even in otherwise primitive taxa, is secondary! In Archaeognatha, this loss is very probably due to their early change to cryptic lifestyle in narrow places. Later on, †Cercopoda (Fig. 13) and silverfish (the sister of Pterygota) (Kukalová-Peck 1987, Figs. 15–17; 1991; 1998) also entered the same habitat and diverged from the free-living main stock, but only after their protowings were larger and more advanced. Therefore, the latter became fused to the terga as wing-based sidelobes separated by deep sutures (similar as prothoracic sidelobes and wing pads in modern nymphs (Fig. 2), but sutures are retained only in modern nymphal peloridiids). Evidence: Structure: Modern silverfish bear inside their thoracic sidelobes the wing tracheae with a veinal branching pattern, which shows them as derived from wings (Šulc 1927); in Paleozoic silverfish, they are separated from terga by deep sutures like in prothoracic winglets. Comparative morphology: Epicoxal exites in Crustacea, Syncarida (Tasmanian shrimp Anaspides tasmaniae) and Branchiopoda (fairy shrimp Artemia sp.) are genetically serially homologous to insect wings (see references above), and serve as articulated and movable aquatic plate gills; the abdominal plate gills in modern mayfly nymphs are in a position serially homologous to thoracic wings, and are mobilized by coxal muscles like flying wings. They are not serially homologous to and derived from, abdominal leglets (Norling 1982; Kukalová-Peck 1983, 1985).
Vegetation at the Onset of Flight Preadaptation
The oldest known fossil Archaeognatha and the oldest known dicondylous jaws were both found in the Early Devonian. Therefore, the shared insect ancestor of Monocondylia (with protowings lost: †Monura + Archaeognatha), plus Dicondylia (with protowings retained and reduced/fused only in cryptic groups: †Cercopoda, silverfish, Pterygota) occurred either in the Silurian or Ordovician, up to 500 million years ago. Silurian vegetation was less than a meter high and grew in very dense patches rooted in mud and shallow water. Before this habitat became available, terrestrial insects could have lived on algal mats and in algal beach drift. In any case, long adaptation toward powered flapping aerial flight must have started in habitats with low-growing vegetation.
Flight Preadaptation in Ordovician and Silurian insects
In aquatic mandibulate Arthropoda and early Crustacea, the epicoxal exites probably functioned as respiratory/locomotory plate gills. Their very oldest function in water-saturated vegetation could have been as movable auxiliary respiratory organs (see a review by Kingsolver and Koehl 1994). In the early dicondylians, protowings most probably somehow assisted the escape from numerous arthropodan predators of that time (Shear and Kukalová-Peck 1990). Inactive protowings would have ended up fused to terga as wing-based sidelobes separated by a suture.
Skimming Hypothesis
This hypothesis of the origin of flight is the only one compatible with the evidence in oldest fossils, assembled over the years by this author. Marden (1995, 2003) proposed that escaping insects landed on the water surface which supported their abdomen, then flapped vigorously their short protowings to skim away from the predator. Later, they became capable of both faster skimming and short flying episodes. Marden’s inspiration was modern stoneflies with secondarily reduced wings, which escape in this way from predators. This active and functionally close flapping movement explains very well why and how the veinal system, articulation, musculature, size, wing membrane, etc. would gradually prepare for the rigors of powered flapping aerial flight.
Vertical Jumps Hypothesis
Yet another ancient escape device, which might have predated or initially be combined with, an escape by skimming on water deserves attention. Jumping bristletails (Archaeognatha) share with some modern Crustacea ancient rope muscles, which “wind up” inside the body and, when released, generate a series of high vertical jumps (Manton 1977). Sudden jumps startle the predator but are uncontrolled, and the last one might end on a predator’s back. When a jumping bristletail dies, the rope muscles contract its body into a typical arched position (Fig. 13).
The Carboniferous and Permian fossil record includes about 400 cryptic bristletail-like insects fossilized in an arched position. For a long time, these were a taxonomic puzzle, but they are now recognized as an early dicondylous order †Cercopoda (Kukalová-Peck 1998, Fig. 19.6b; see also: Kukalová-Peck 1987, Fig. 10, 1991). †Cercopoda (Fig. 13) share with other Dicondylia broad dicondylous jaws; wing-based thoracic sidelobes delimited by sutures; long, massive ovipositors with a gonangulum; but bear plesiomorphic double-clawed cercopods instead of cerci and abdominal rope muscles. This shows them as the sister of silverfish + winged insects (Dicondylia = (†Cercopoda + (Zygentoma + Pterygota))). Ancestral rope muscles, a symplesiomorphy with Archaeognatha and Crustacea, are reduced in silverfish + pterygotes. A discovery of rope muscles adds an unexpected, new aspect to the origin of insect flight. The oldest Dicondylia bore epicoxal protowings (the same as plesiomorphic Crustacea bore epicoxal exite-gills; see above) while both groups had abdominal rope muscles and escaped predators by their contractions (vertical jumping/swimming). In terrestrial Crustacea a similar escape still occurs in landhoppers (terrestrial amphipods), such as in Tethorchestia antillensis common in Jamaican forests. Protowings very probably initially functioned in cohut with vertical jumping and possibly earned their existence by prolonging jumps, allowing insects to be swept away by wind, and controlling landing. Precisely how they did it, and what the effect of flapping may have been (if any), must be tested experimentally.
Ancestral Protowing-Bearing Insects: A Composite Model
Evidence available for this model is as follows. Fossil record: Paleozoic insects bore the following set of appendages: 12 pairs of mobile protowings (3 larger pairs on the thorax; 9 probably smaller pairs on the abdomen); and 15 pairs of telopodite limb appendages with flexible, climbing, once subsegmented tarsi, ending in pretarsi with curved double claws (two pairs on the head, three pairs on the thorax, nine pairs on the abdomen, plus one pair of cercopods (only in †Cercopoda)). Of these, the maxillary palps were probably long and strong, arched anteriorly and pulling the body forward, abdominal leglets quite long, and the cercopods were arched posteriorly and probably engaged in pushing and stabilization (as in Archaeognatha, †Monura and †Cercopoda, Figs. 7, 8, 10, 12, 13) (Kukalová-Peck 1983; 1987, Fig. 10; 1998, Figs. 19.5, 19.6). Since the oldest insects with the smallest protowing exites had abdominal rope muscles, they were able to jump above the dense, low growing vegetation. With the help of flapping protowings and perhaps also extended legs and leglets, they possibly were able to direct their jumps away from the predator, toward open water, or take better advantage of the wind and escape. Later on, the rope muscles diminished, and the insects landed on the water surface and skimmed away from danger by flapping their short protowings. This activity prepared their muscular apparatus, wing articulation and wings themselves for powered flapping flights (Marden 1995, 2003; Kukalová-Peck 1998; this paper). This flight model is preliminary, tests are wanting.
Groundplans in Systematics, From the Perspective of the Post-Groundplan Approach
Beutel and Pohl (2006) criticized the use of wing-based groundplans in the phylogeny of Pterygota as “limited to a single set of characters.” This implies using too few characters for meaningful results. In reality, wing venation/articulation is a massive, hugely diversified organ system, which offers many sets of characters, each of them descending from the Pterygota level, to divisions, lineages, superorders, orders and suborders (e.g., divisions Paleoptera and Neoptera differ in astonishing 65 wing characters, Haas and Kukalová-Peck 2001). The groundplan method is designed to work only with fully homologized arthropod characters. Besides wings, so far only the limb organ system (Fig. 1) is ready to produce independently reconstructed phylogenies. In this, Beutel and Pohl (2006) overlooked the now available data: for instance confirmation of the wing-based basal split in Neoptera in the divergence of female genitalia (Fig. 20, here). As shown by Sharov (1957, 1966) and Hennig (1981), the ovipositor third valve (gonoplac) in Neoptera is formed in two different ways: either by the stiffened gonostyli (in Orthoneoptera + † ancestral Pleconeoptera), or by the elongated gonocoxites (in Blattoneoptera + Hemineoptera + Endoneoptera). The authors also overlooked that the same basal split of Neoptera is repeated in three separate wing character sets: (i) in two alternative irreversible fusions of the medial sector MA (MA fused either with MP into the medial stem, or MA fused basally with radius), (ii) in two alternative irreversible veinal supports in anojugal lobes of the hind wings (both anal sectors present or AA reduced; two different arrangement and function of flexion lines and folds), and (iii) in two alternative irreversible expressions of the second axillary in the wing articulation (Kukalová-Peck and Lawrence 2004). This shows that the same basal split in Neoptera lineages occurs in four sets of characters, veryfiable in modern insects. All characters involved are fully homologized under Pterygota, and are judged by their distance from the shared monophyletic ancestral model by using the irreversibility rules valid in all pterygote higher taxa. This rather recently proposed substantial change in the higher system of Pterygota is now wide open to verification, exploring and supplementation by additional data in other biological fields.
Why Any Future Remedies to the Post-Groundplan Method Will not Work
The ongoing failure of the post-groundplan method to objectively resolve insect higher phylogenies has generated some suggestions for an improved approach. The most frequently proposed remedies, which work in the lower taxa, recommend: (1) maximizing the number of the processed morphological character states; (2) combining morphological and molecular character states in huge, mixed datasets called total evidence; and (3) replacing the (supposedly) uninformative morphological character states by molecular character states, after they will be improved in the future (Beutel and Pohl 2006; Wheeler et al. 2000; Kristensen 1995; 1998).
These remedies are fatally flawed because they invariably sidestep two Hennigian basal rules of phylogenetic systematics: using only (1) the fully homologized characters; and, (2) at their groundplan level. Enlarged datasets do improve results in the lower taxa, which do not have this problem: they have easily homologized characters, at or close to the groundplan level. However, just the opposite is true in the higher taxa. Their groundplan character states (which solely include easily recognizable synapomorphies) are obscured by later changes, and are both unknown and not fully homologized between extant orders, lineages and divisions. This jeopardy is the real cause of unsolvable higher phylogenies, and it cannot be either sidestepped or replaced by computerized methods! Combining morphological and molecular datasets is helpful in lower taxa, in which molecular analysis often delivers additional character states. In higher taxa molecular analysis very often delivers unrealistic phylogenetic reconstructions. In principle, mixing molecular and visually selected morphological data for “better” phylogenetic support is objectionable on the same grounds, as once was numerical taxonomy. As a conclusive fact, any subjective concoctions of inadequately understood characters from different sources, with or without processing, are as futile an exercise as were the thousands of exact measurements defining numerical taxa. Evolution of morphological structures from the phylum level down to classes, divisions, lineages and extant orders, is morphologically marked by the diversification of organ systems. This can be traced, compared and interpreted for phylogenetic purposes, as often done in vertebrates, and now also in arthropods. In contrast, the same evolutionary events are not reflected by larger numbers of similar post-groundplan character states found in the descendant derived fauna, no matter how carefully and cleverly they are analyzed or processed! There are just too many unpredictable “false” synapomorphies, and larger datasets will only manipulate even larger numbers of unrecognizable false data, with no objective defendable solution in sight. For more evidence, see Kukalová-Peck (1998), Haas and Kukalová-Peck (2001), Kukalová-Peck and Lawrence (2004), Wheeler (2004), and Schwartz and Maresca (2006).
Phylogeny of the Higher Taxa Based on Morphological Characters
This paper is offering evidence that the present problems with higher phylogenies are not caused by any shortcomings of morphology (as sometimes proposed), but solely and only by unsuitable analytic methods, which bypass systematic rules and use quite insufficient evidence. Morphology is the corner stone and essence of the entire morphological system at all its taxonomic levels and definitely not a culprit of its failures! Realistic morphological phylogenetic trees reflect the nodal evolutionary events in morphological organ systems, as they evolve and diversify from a single ancestor. The foundation of shared groundplan characters, recognized when compared with the shared ancestor, defines each higher taxon so clearly that it allows us to discern and group together modern insect orders instantly by eyeball! In insects, often a single detached wing provides all the information needed for recognizing it to order. Groundplan characters always include synapomorphies. Since many related insect orders are recognized by us instantly in living insects (as “orthopteroids” or “blattoids”), they obviously do exist. Identifying them for scientific purposes is quite clearly the matter of our dilligence and the choice of suitable systematic method.
For almost a century, the related modern orders were grouped together visually for practical purposes, as paleopterous insects (=mayflies, dragonflies); plecopteroids; orthopteroids; blattoids; hemipteroids; and endopterygotes. By applying the groundplan method in the limb/wing organ system, this author and co-authors identified their groundplan character states, recognized synapomorphies they shared, and validated them as legitimate pterygote divisions and lineages, as follows: division Paleoptera with lineages (†Palaeodictyopterida + (Ephemeroptera + Odonatoptera)); and division Neoptera, with lineages (Pleconeoptera + Orthoneoptera) + (Blattoneoptera + (Hemineoptera + Endoneoptera)) (Haas and Kukalová-Peck 2001; Kukalová-Peck and Lawrence 2004).
Summary
Solutions to the Phylogenetic Problems in Arthropod Higher Taxa
Relationships among the modern higher taxa of Insecta/Arthropoda are determined by synapomorphies included in the groundplans of sister groups at the event of their divergence. In almost all extant orders and in all lineages, divisions and other higher taxa, this divergence took place more than 245 million years ago, in the Paleozoic era. Then, during the Great end-Permian Extinction, several million years of oxygen deprivation dramatically cut down insect diversity to a handful of largely derived species (according to Hennig 1981, to as little as 40–50 stem species). These slowly re-populated dry land by passing through a narrow environmental bottleneck of a devastated landscape. As a result, the Mesozoic-to-Quaternary entomofauna of families, genera and species under Paleozoic orders, is usually significantly derived, with parallel reductions and fusions, disconnected and very much dissimilar when compared with Paleozoic ordinal groundplans. These were invariably much richer in podites, exites, leglets and outer genitalia, sclerites perfectly delimited by sutures, barely developed veinal braces, and other plesiomorphies all of which were lost independently and many times in parallel in the extant fauna. Yet, it is these old, unfamiliar sister group groundplans that solely harbour distinct synapomorphies! In order to recognize them, we must go all the way back and first record and then reconstruct evolution of an entire organ system, from the shared monophyletic ancestor to present times.
Currently used systematic methods are successfully applied in extant species, genera and families, in reconstructing phylogenies from the character states present in extant species. These are cladistically analysed and computerized. However, when applied in higher taxa (orders, lineages, divisions, and classes) these methods have been unable to identify synapomorphies and resolve relationships. This is because the post-extinction families, genera and species of unrelated higher taxa developed many convergent similarities called homoplasies (as they adapted to similar habitats), which are masquerading as synapomorphies. The problem is so severe that presently the majority of higher relationships is in dispute. Alternative solutions abound and some systematists see as a culprit morphology, which “does not provide synapomorphies distinctive enough in the higher taxa”.
As documented by Hennig (1969, 1981), only the groundplan characters of higher insect taxa contain clear, unambiguous morphological synapomorphies, which are more frequent than false synapomorphies (homoplasies, parallelisms) and easily separable from them. Thus, the groundplan character states are the Rosetta stone of higher systematics, solving problems and generating answers. Groundplan characters must always be identified in modern constituent species before higher taxa are phylogenetically analyzed. Their identification is done with the help of the groundplan systematic method. This has been extensively used in vertebrates and by paleontologists, and has now also been applied in the limb/wing organ system in arthropods. The application is discussed and demonstrated on examples in this account.
The Groundplan Method
This systematic method traces and records the character transformation series in all insect lineages, from the Paleozoic to the Present time, and uses these concrete data for reconstructing the monophyletic ancestor in an organ system. The ancestor is repeatedly tested and crosschecked, corrected in all lineages, and against all data available in genetics, ontogeny, embryology, etc. It is considered complete only after all character states (in existence) can be flawlessly derived from it, by using the character transformation series advancing exactly as they were recorded from specimens. This ancestral model is also used as a reference for homologizing organ systems of different orders. Since the groundplan character states of extant higher taxa are irreversible (Hennig 1969, 1981), a simple reference to the ancestral model shows which character states in their constituent living species are (1) present in all representatives (visibly or obscured), (2) closest to the ancestral model, and (3) irreversible. Example: In the limb/wing organ, the least fused and least reduced states with regard to the ancestral model, available in a significant sample of ordinal constituent species, are also the groundplan character states of this order (see above text). These must be researched prior to analysis. Once established, a comparison of groundplan character states between analyzed orders (or other higher taxa) usually instantly reveals the synapomorphies shared by ordinal sister groups. The groundplan method was applied to the arthopod limb/wing organ, which was gradually upgraded for the last 30 years and is offered here in several evolutionary models (Figs. 1, 15, 17–20). Its potential in Insecta is still far from being exhausted, and it has not yet been broadly applied to Crustacea and Chelicerata in published contributions.
By no means is this author trying to imply that the groundplan method is “infallible”. Solving some evolutionary puzzles took 30 years of effort, and was paved with mistakes. However, there is a difference: when used in higher taxa, this method is transparent, objective and repeatable because all character states are judged by their step-wise distance from the same shared ancestor and by using the same systematic rules based on concrete character transformation series. The results are objective since they can be independently crosschecked and updated when new material occurs. In contrast, the currently used systematic methods are designed for solving quite different systematic problems in the lower taxa, and their results become quite opaque and subjective in the higher taxa. This is because they choose homologues and identify synapomorphies from several “eligible” similarities. Resulting multiple and conflicting phylogenies are presented as perennial “democratic possibilities”—but phylogeny is about objective reasons and straight answers.
Note that in the insect lower taxa, the character states are best analyzed by the presently used systematic methods. The groundplan method is stumped by the presence of reversibility in their character states, as it is not able to handle reversals.
History of the Groundplan Method
The bulk of synapomorphies in the arthropod higher taxa comes from the limb/wing organ systems. Sharov in his book (1966) became a pioneer of the groundplan method by reconstructing the ancestral insect limb model with respect to the data available in other Arthropoda and in biological fields. This model and the method was upgraded during the past three decades, through the broadly based comparative research by E. L. Smith and this author, in cooperation with several specialists in modern groups. E. L. Smith almost completely homologized the arthropod limb, except for the insect hypopharynx and some highly derived male genitalia (Fig. 1). J. Kukalová-Peck contributed evidence from Paleozoic fossils (Figs. 2–5, 7, 12a, 13, 16, 19, 20, 21). In a separate project, this author homologized insect protowing and protoarticulation, with the input from other biological fields (Figs. 2, 3, 7, 13–15, 17–19). The groundplan method made it possible to separate synapomorphies in extant higher taxa of Hexapoda, from numerous homoplasies and parallelisms. Newly reconstructed phylogenies are mentioned only shortly, because the background documentation is quite extensive and is available in relatively recent publications cited in the text.
Misconceptions
The groundplan method is very different from currently used systematic methods designed to solve problems in the lower taxa. The latter rely mainly on character states directly observed in living insects and on their cladistic analysis processed by computerized methods. Related lower taxa show the largest number of similarities present in several character series. The groundplan research (involving Paleozoic groundplans recognized and homologized through reference to a shared ancestor) is not needed since their groundplan level characters are more or less evident (except for reversals) and the character states are comparatively easy to homologize. But, unfamiliarity breeds doubt. One frequent misconception is a distrust of objectivity in the groundplan method: the shared ancestor (essential for judging character states in the higher taxa) is imagined as a “subjectively constructed intuitive model” (something like “live” Dinosaurs), which may vary from author to author. Nothing is farther from the truth. The ancestral model consists of concrete plesiomorphies assembled through long-term very broad research. It must be instantly updated, when a more plesiomorphic state is discovered. Example: Kukalová-Peck and Lawrence (2004) had to update, with much trouble, the ancestral anojugal field in the Neoptera and Coleoptera. This update instantly showed Coleopterida as the sister of (Neuropterida + Mecopterida), and Neoptera as split into two super-lineages: (Pleconeoptera + Orthoneoptera) and (Blattoneoptera + (Hemineoptera + Endoneoptera)). Both relationships were reinforced by other character sets, shared irreversible synapomorphies in veinal fusions and braces, and two different origins of the third ovipositor valvula (Fig. 20).
Other misconceptions are that the Paleozoic groundplan character states of modern orders are supposedly (i) “unstable” and (ii) completely “replaced” in modern fauna by younger character states. Instead, they are irreversible, which fact is easily verified by comparing the character states by eye. They are exactly the same in the Paleozoic and the extant representatives (Hennig 1981). They may be occasionally obscured by younger reduction or other modification, but this definitely does not “remove” their presence from the groundplan! They are only hidden. A modern order devoid of its ordinal character states in one or two families and a handful of genera is an empty and absurd taxonomic concept! As a fact, modern families differ in their autapomorphies, which are variously added on top of the shared ordinal groundplan framework. The “visibility” of ordinal states in families varies but allegiance to the order is permanently there, irreversibly so whether the characters are visibly expressed or obscured.
Why the Current Systematic Methods Do not Work in the Higher Taxa?
Almost all current problems with higher-level phylogenies result from using unpredictably derived, and partly or erroneously homologized character states, as their source of synapomorphies. Such character states are misleading because they include many false synapomorphies (convergencies, homoplasies, and parallelisms) and cannot be trusted. The presently used systematic methods are designed to solve problems with reversals, ring species, crypto-species, hybrids, etc., which bedevil lower taxa. However, they are not at all designed to recognize Paleozoic ordinal groundplan characters and to separate synapomorphies they include from similar-looking parallelisms and convergent states (homoplasies) autapomorphically added in the Mesozoic-to-Quaternary families, genera and species of unrelated higher taxa.
The current systematic approach dealing with volatile lower taxa is focused on the weight of numbers of similarities in different character sets and on their computerized analysis, which links them together. Larger numbers of post-groundplan similarities, occurring in larger numbers of character sets, are often an indicator of a real relationship. Unfortunately, the great end-Permian Extinction disrupted this gradual flow of adaptations, which existed in the Paleozoic. As a result, the raw numbers of younger, post-extinction similarities are mostly uninformative to relationships between the higher taxa. In the not so distant past, enormous rows of numbers in numerical taxonomy costing years of effort, turned to be equally useless! Presently, many conflicting and quite unrealistic higher phylogenies based explicitly on post-groundplan similarities, are offered and tolerated in publications. This approach is on a collision course with the long known and accepted fact that the post-groundplan changes are irrelevant for higher relationships (Hennig 1969, 1981). Not surprisingly, the most “enigmatic”, “inpenetrable” and hotly debated phylogenies concern the basal sister groups inside the oldest taxa: Mandibulata, Atelocerata, Parainsecta, Insecta. In Pterygota, the most debated phylogenies concern pterygote basal divisions, lineages and basal orders in these lineages. It is proposed in this account that these relationships are all solvable by the groundplan method in an objective and repeatable way (see documentation above and in Figs. 1–21).
Computerized Cladistic Analysis
This approach works for both lower and higher taxa, but there is a difference. In lower taxa, it can be used directly for all character states, because their character states are at- or close to their groundplan and homologization is not a problem. But in higher taxa, it can be applied only after the character states of an organ system, used in the analysis, were set at their groundplan level and fully homologized. Unless this extra step is fulfilled, it is impossible to (1) identify correctly all synapomorphies, and (2) to separate them from homoplasies and parallelisms. When the groundplan method is used, cladistic analysis and computer processing is not necessary for reaching phylogenetic decisions. But, it is frequently used anyway (e.g., by this author and co-authors), because it presents character states and their judgments in an easy to use arrangement.
Morphological Research of Living Insects
Using the groundplan method does not in any way diminish the importance of a comparative morphological study of modern insects. Just the opposite is true, but the emphasis is often on informative minute details. Nevertheless, even the most thorough study may fall short of an evolutionary insight, because of the massive evidence erased by the end-Permian Extinction. The post-extinction taxa show rampant parallelism and convergence in derived character states, which can look convincingly like a monophyletic state. Example: Under the present-day focus only on insects, with emphasis on their extant character states and their numbers, some entomologists started again using insect limb-derived appendages, as if they had only 6–7 segments (as proposed by Snodgrass 1935 and used to deconstruct arthropods by Manton 1977); were not serially homologous in segments; bore secondary de-novo rami instead of sharing Proterozoic, all-arthropod exites and endites; and the wing articulation is evaluated, as if articular sclerites evolved from fragmented tergum (but, they evolved from the limb). It is forgotten that three decades ago the same old, “direct”, but naive approach to limb evolution and homology exploded Arthropoda and started a massive debate unparalleled in the history of modern biology. As during the heady era of numerical taxonomy, the lure of numbers is again eroding an evolutionary approach, in spite of strong and ever growing evidence supporting evolution (see Figs. 1–21 above).
Living species can deliver at best only about 50% of the evidence necessary for the objective assessments of inter-ordinal and higher relationships. The remaining data come from the arthropod homologues, ontogeny, genetics and developmental genetics, and from tracing and recording the actual character transformation series, as they cascade from the monophyletic ancestor down toward Mandibulata, Atelocerata, Hexapoda, Insecta, Pterygota, divisions and lineages, and to the ordinal character states expressed in living constituent species. As shown here, this evolutionary history can be tracked in the limb/wing organ systems, to produce defendable and objective phylogenetic reconstructions in the modern higher taxa.
Need for Thematic Research
The current practice of gathering and processing various inter-ordinal “similarities” and then offering several “feasible” ordinal relationships is an unfortunate and evasive, rather than a vigorous problem solving, strategy. Hennig (1969, 1981) specifically called for, and practiced the thematic research of modern morphological organs including their past evolution as witnessed in the fossil record. This type of research strives to extract a single solution from multiple sources. First, data from extant and extinct insects are collected and interpreted as a working hypothesis; then, this hypothesis is tested against additional sources and updated; then, more data are recorded and tests are made; then, this cycle is repeated until all possible sources are covered and all new data fall repeatedly into the same place. In modern Insecta, each higher taxon sought to be based on evidence is considered to be monophyletic, i.e., it is expected to have only one ancestor, one groundplan, one sister group, and one character transformation series, all bound together by one course of evolution. The goal is to find all this information and to arrive at a single, broadly verified decision, in which all facts must fit flawlessly together. The present-day tolerance of multiple “democratic” solutions to phylogenies stands scientific principles on the head. First of all, multiple higher “relationships” are clearly caused by false synapomorphies, yet they are presented as several results (presumed unsolvable under present circumstances). In thematic research such “results” would rank as a first draft of conflicting raw data with which the research cycle should begin. After merely scratching the surface and discovering that the first handful of options cannot be yet decided, some entomologists claim to have “proved” that morphology is “incapable” of providing clear higher-level synapomorphies! In fact, just the opposite is true but the entire research program must be all-encompassing, and focused on evolution in the broadest of sense.
The Future of Arthropod Phylogeny
The obstacles to progress in the higher morphological system of Arthropoda/Insecta were explored by Wheeler (2004). Some systematists appear to have missed all updates in arthropod limb morphology since Snodgrass and Manton by calling Hexapoda “uniramous” and Crustacea “biramous” (as a fact, extant Archaeognatha are polyramous with up to three retained exites (Fig. 8), extant basal Crustacea bear up to four exites, all Arthropoda chew with two endites, and Trilobita bear up to nine endites). Rather than accepting that a very large part of evolution is reduction, some systematists conceptualize instead that Arthropoda move around on “polyphyletic” limbs, and morphology is “incapable” of resolving (its own) morphological system! As mentioned in my papers and discussed with many colleagues interested in the grand evolution of Hexapoda, the current trend in higher-level systematics has swung too much toward engineering the data, and too far away from researching their origin and evolution within the organ systems. A healthy balance is lost with deleterious consequences. Until this is restored, well-supported higher phylogenies will remain in very short supply.
The Possible Benefit for Molecular Analysis
No field in biology can be neglected for long without retarding progress in other fields as well. One costly consequence (in grant money) of current marginalizing evolutionary morphology is the burgeoning numbers of conflicting phylogenetic reconstructions of the higher taxa produced by molecular analysis. Most of them have been fruitlessly disputed for over a decade with no end in sight! Like other disciplines, molecular analysis does not operate in a vacuum and urgently needs crosschecking of its results, as well as complementary evidence and further clues from other biological fields, to verify its endeavors. Some molecular biologists will probably agree that their most rigorous results are likely to correlate with a well-documented morphological phylogenetic tree. Perhaps it would be worth of giving a crosscheck a good, energetic try!
A Final Word of Hope
For about seven decades now, erroneously interpreted major organ systems in arthropods have been obsfuscating the results of phylogenetics, systematics, and other biological fields, including currently explosively developing molecular analysis. Perhaps, the time has come to update evolutionary morphology and higher systematics of arthropods on a broad scale with more realistic (and less mechanistic), evolution-minding method, and to make the data available to molecular analysis and other biological fields, for their special purposes.
References
Abouheif, E., & Wray, G. A. (2002). Evolution of the gene network underlying wing polyphenism in ants. Science, 297, 249–252.
Averov, M., & Cohen, M. (1997). Evolutionary origin of wings from ancestral gills. Nature, 385, 627–630.
Bechly, G., Brauckmann, C., Zessin, W., & Gröning, E. (2001). New results concerning the morphology of the most ancient dragonflies (Insecta: Odonatoptera from the Namurian of Hagen-Vorhalle (Germany). Journal of Zoology, Systematic Evolutionary Research, 39, 209–226.
Benton, M. (2003). When life nearly died. London: Thames & Hudsoon, 330 p.
Beutel, R. G., & Pohl, H. (2006). Endopterygote systematics—Where do we stand and what is the goal (Hexapoda, Arthropoda)? Systematic Entomology, 31, 202–219.
Bininda-Emonds, O. R. P., Bryant, H. N., & Russel, A. P. (1998). Supraspecific taxa as terminals in cladistic analysis: implicit assumptions of monophyly and a comparison method. Biological Journal of the Linnean Society, 64, 101–133.
Bocharova-Messner, O. M. (1959). Development of the wing in the early postembryonic stage in the ontogeny of dragonflies (order Odonata). Institut Morfologii Zhitelnych Imeni Severtsova, 27, 187–200, in Russian.
Boudreaux, H. B. (1979). Arthropod phylogeny with special references to Insects. New York: Wiley & Sons, 320 p.
Brodsky, A. K. (1994). The evolution of insect flight. Oxford: Oxford University Press, 229 p.
Carpenter, F. M. (1992). Treatise of invertebrate paleontology, superclass Hexapoda, part R, Arthropoda, 3. Boulder, Colorado: The Geological Society of America, 655 p.
Carpenter, F. M., & Richardson, E. S. (1968). Megasecopterous nymphs in Pennsylvanian concretions from Illinois. Psyche, 75, 295–309.
Carpenter, F. M., & Richardson, E. S. (1971). Additional insects in Pennsylvanian concretions from Illinois. Psyche, 78, 267–295.
CSIRO. (1991). The insects of Australia. The textbook for students and research workers. Naumann, I. F. (Chief Ed.). Melbourne University Press, Melbourne, 1137 p.
Erwin, D. H. (1993). The Great Paleozoic Crisis. Life and death in the Permian. Columbia University Press, 327 p.
Garcia-Beillido, A. (1975). Genetic control of wing disc development in Drosophila. In Cell Patterning. Ciba Foundation Symposium, Vol. 29, pp. 161–182.
Grimaldi, D., & Engel, M. S. (2005). Evolution of the insects. New York: Cambridge University Press, 755 p.
Haas, F., & Kukalová-Peck, J. (2001). Dermaptera hindwing structure and folding: new evidence for familial, ordinal and superordinal relationships within Neoptera (Insecta). European Journal of Entomology, 98, 445–509.
Hennig, W. (1969). Die Stammesgeschichte der Insekten. Frankfurt am Main: Kramer, 436 p.
Hennig, W. (1981). Insect phylogeny. New York: Wiley & Sons, 514 p.
Hovmöler, R., Pape, T., & Kallersjö, M. (2002). The Paleoptera problem: basal pterygote phylogeny inferred from 18S and 28S rDNA sequences. Cladistics, 18, 313–323.
Kjer, K. M. (2004). Aligned 18S and insect phylogeny. Systematic Biology, 53(3), 506–514.
Kingsolver, J. G., & Koehl, M. A. R. (1994). Selective factors in the evolution of insect wings. Annual Review of Entomology, 39, 425–451.
Kristensen, N. P. (1991). Phylogeny of extant hexapods. The insects of Australia. A textbook for students and research workers (2nd ed.), Vol. 1. Melbourne: Melbourne University Press, pp. 125–140.
Kristensen, N. P. (1995). Forty years’ insect phylogenetic systematics. Zoologische Beitrage, (N.F.), 36, 83–124.
Kristensen, N. P. (1998). The groundplan and basal diversification of the hexapods. In R. A. Fortey, & R. H. Thomas (Eds.), Arthropod Relationships (pp. 281–293). London: Chapman & Hall.
Kukalová-Peck, J. (1969). Revisional study of the order Palaeodictyoptera in the Upper Carboniferous shales of Commentry, France. Part I. Psyche, 76(2), 163–215.
Kukalová-Peck, J. (1970). Revisional study of the order Palaeodictyoptera in the Upper Carboniferous shales of Commentry, France. Part II and III. Psyche, 76, 439–486; 77, 1–44.
Kukalová-Peck, J. (1974). The order Megasecoptera (Insecta) from the Lower Permian of Moravia with description of new families and nymphal development. Psyche, 82, 1–19.
Kukalová-Peck, J. (1978). Origin and evolution of insect wings and their relation to metamorphosis, as documented by the fossil record. Journal of Morphology, 156, 53–126.
Kukalová-Peck, J. (1983). Origin of the insect wing and wing articulation from the arthropodan leg. Canadian Journal of Zoology, 62, 618–1669.
Kukalová-Peck, J. (1985). Ephemeroid wing venation based upon new gigantic Carboniferous mayflies and basic morphology, phylogeny, and metamorphosis of pterygote insects (Insecta, Ephemerida). Canadian Journal of Zoology, 63, 993–955.
Kukalová-Peck, J. (1987). New Carboniferous Diplura, Monura, and Thysanura, the hexapod ground plan, and the role of thoracic lobes in the origin of wings (Insecta). Canadian Journal of Zoology, 65, 2327–2345.
Kukalová-Peck, J. (1991). Fossil history and the evolution of hexapod structures. The Insects of Australia. A textbook for students and research workers (2nd ed.), Vol. l. Melbourne: Melbourne University Press, pp. 41–179.
Kukalová-Peck, J. (1992). The “Uniramia” do not exist: the groundplan of Pterygota as revealed by the Permian Diaphanopterodea from Russia (Insecta: Paleodictyopteroidea). Canadian Journal of Zoology, 70, 236–255.
Kukalová-Peck, J. (1998). Arthropod phylogeny and “basal” morphological structures. Arthropod Relationships. In R. A. Fortey & R. H Thomas (Eds.), London: Chapman & Hall pp. 249–268.
Kukalová-Peck, J., & Brauckmann, C. (1990). Wing folding in pterygote insects, and the oldest Diaphanopterodea from the early Late Carboniferous of West Germany. Canadian Journal of Zoology, 68, 1104–1111.
Kukalová-Peck, J., & Brauckmann, C. (1992). Most Paleozoic Protorthoptera are ancestral hemipteroids: Major wing braces as clues to a new phylogeny of Neoptera (Insecta). Canadian Journal of Zoology, 70, 2452–2473.
Kukalová-Peck, J., & Lawrence, J. F. (2004). Relationship among coleopteran suborders and major endoneopteran lineages: Evidence from hind wing characters. European Journal of. Entomology, 101, 95–144.
Kukalová-Peck, J., & Richardson, E. S. Jr. (1983). New Homoiopteridae (Insecta: Paleodictyoptera) with wing articulation from Upper Carboniferous strata of Mazon Creek, Illinois. Canadian Journal of Zoology, 61, 670–687.
Maas, A., Waloszek, D., & Müller, K. J. (2003). Morphology, ontogeny and phylogeny of the Phosphatocopina (Crustacea) from Upper Cambrian ‘Orten’ of Sweden. Fossils and Strata, 49, 1–238.
Manton, S. M. (1977). The Arthropoda. Habits, functional morphology and evolution. Oxford: Clarendon Press, 527 p.
Marden, J. H. (1995). Flying lessons from a flightless insect. Natural History, 2(95), 6–8.
Marden, J. H. (2003). The surface-skimming hypothesis for the evolution of insect flight. Acta zoologica cracoviensia, 46(Suppl.-Fossil Insects), 73–84.
Marshall, C. R., Raff, E. C., & Raff, R. A. (1994). Dollo’s law and the death and resurrection of genes. Proceedings of National Academy of Sciences, USA, 91, 1283–1287.
Martynov, A. V. (1923). On two basic types of insect wings and their significance for the general classification of insects. In: Deryugina, K. M. (Ed.), Trudy Pervogo Vserossijskogo S’ezda Zoologov, Anatomov i Gistologov, Vol. 1, pp. 88–89.
Martynov, A. V. (1924). Über zwei Grundtypen der Flügel bei den Insekten und ihre Evolution. Zeitschrift für Morphologie und Oekologie, 4, 465–501.
Martynov, A. V. (1931). New Permian Paleoptera with the discussion of some problems of their evolution. Trudy Paleozoologicheskogo Instituta, 1, 1–44.
Norling, U. (1982). Structure and ontogeny of the lateral abdominal gills and the caudal gill in Euphaeidae (Odonata: Zygoptera) larvae. Zoologisches Jahrbuch Anatomy, 107, 343–389.
Paganiban, G. A., Serbin, L., Nagy, I., & Caroll, S. (1995). The evolution and patterning of insect limbs. Current Biology, 4, 671–675.
Popadić, A. G., Paganiban, G. A. Rush, Shear, W. A., & Kaufmann, T. C. (1998). Molecular evidence for the gnathobasic derivation of arthropod mandibles and for the appendicular origin of the labrum and other structures. Development Genes and Evolution, 208, 42–150.
Prendini, L. (2001). Species or supraspecific taxa as terminals in cladistic analysis? Groundplans versus exemplars revisited. Systematic Biology, 50, 290–300.
Raff, R. A. (1996). The shape of life. Genes, development, and the evolution of animal form. University of Chicago Press, 520 p.
Raff, R. A., Wray, G. A., & Henry, J. J. (1991). Implications of radical evolutionary changes in early development for concepts of developmental constraints. In L. Waren & H. Koprowski (Eds.), New perspectives on evolution (pp. 189–207). New York: Wiley-Liss.
Riek, E. F., & Kukalová-Peck, J. (1984). A new interpretation of dragonfly wing venation based upon Early Upper Carboniferous fossils from Argentina (Insecta: Odonatoidea) and basic character states in pterygote wings. Canadian Journal of Zoology, 62, 1150–1166.
Ross, H. H. (1974). Biological systematics. Addison-Wesley Publishing Company, Inc., 345 pp.
Schwartz, J. H., & Maresca, B. (2006). Do molecular clocks run at all? A critique of molecular systematics. Biological Theory, 1(4), 357–371.
Sharov, A. G. (1957). Peculiar Paleozoic wingless insects of the new order Monura (Insecta, Apterygota. Doklady Akademii Nauk USSR, 115, 796–798.
Sharov, A. G. (1966). Basic arthropodan stock. London: Pergamon Press, 271 p.
Shear, W. A., & Kukalová-Peck, J. (1990). The ecology of Paleozoic terrestrial arthropods: The fossil evidence. Canadial Journal of Zoology, 68, 1807–1934.
Shubin, N. H., Tabin, C., & Carroll, S. B. (1997). Fossils, genes and the evolution of animal limbs. Nature, 388, 639–648.
Smith, E. L. (1970). Biology and structure of some California bristletails and silverfish. Pan-Pacific Entomologist, 46, 212–225.
Smith, E. L. (1988). Morphology and evolution of the hexapod head. In: Proceedings of the 18th International Congress on Entomology, Vancouver, 73 p.
Snodgrass, R. E. (1935). Principles of insect morphology. New York: Mc Graw-Hill, 667 p.
Stenzhorn, H. J. (1974). Experimentelle Untersuchungen zur Entwicklung des Lymantria dispar L. (Lepidoptera). Wilhelm Roux Archive, Entwicklungen Mechanische Organisation, 175, 65–86.
Šulc, K. (1927). Das Tracheensystem von Lepisma (Thysanura) und Phylogenie der Pterygogenea. Acta Societatis Scientifica Naturalis Moravicae, 4(7/39), 108 p.
Tower, W. L. (1903). The origin and development of the wings in Coleoptera. Zoologisches Jahrbuch, Anatomy, 17, 517–572.
Wägele, J. W. (1993). Rejection of the “Uniramia” hypothesis and implications of the Mandibulata concept. Zoologisches Jahrbuch, Systematik, 120, 253–288.
Wägele, J. W. (1996). First principles of phylogenetic systematics, a basis of numerical methods used for morphological and molecular characters. Vie Milieu, 46(2), 125–138.
Webster, M. (2007). A Cambrian peak in morphological variation within trilobite species. Science, 317, 499–502.
Wheeler, Q. D. (2004). Taxonomic triage and the poverty of phylogeny. Philosophical Transactions of the Royal Society of London, B, 359, 571–583.
Wheeler, W. C., Whiting, M., Wheeler, Q. D., & Carpenter, J. M. (2000). The phylogeny of the extant hexapod orders. Cladistics, 17, 13–169.
Williams, J. A., & Carroll, S. B. (1993). The origin, patterning and evolution of insect appendages. Biological Essays, 15, 567–577.
Willmann, R. (1997). Advances and problems in insect phylogeny. Arthropod relationships. In: R. A. Fortey & R. H. Thomas (Eds.) London: Chapman & Hall, pp. 69–279.
Wootton, R. J., & Kukalová-Peck, J. (2000). Flight adaptations in Palaeozoic Palaeoptera (Insecta). Biological Reviews, 75, 129–167.
Wootton, R. J., Kukalová-Peck, J., Newman, D. J. S., & Muzon, P. (1998). Smart engineering in the Mid-Carboniferous: how well could Palaeozoic dragonflies fly? Science, 282, 749–751.
Yeates, D. K. (1995). Groundplans and exemplars: path of the tree of life. Cladistics, 11, 343–357.
Acknowledgements
Broad evolutionary-morphological contributions in insects are quite impossible without selfless and massive help from systematists specializing in individual orders. I am deeply obliged to many entomologists who, over the years, by their suggestions and their wonderful insect collections, have helped to clarify for me the theoretical and practical aspects of the groundplan method. This includes my past and present co-authors and friends, who patiently shared with me their extensive knowledge of modern and fossil entomofaunas. This essay would never be possible without the profound morphological analysis of arthropods by Edward Laidlaw Smith (The California Academy of Sciences, San Francisco), which I had the privilege to witness and participate in, and without my field assistant Karel Havlata, who organized the fossil excavations in Moravia. Because of my politically challenged origins, my international background in Paleozoic insects was possible only with the most generous support of Frank M. Carpenter, Harvard University, Cambridge, and Ernst Mayr, Museum of Comparative Zoology, Cambridge. With their grant I also studied, over multiple prolonged visits, all Paleozoic insects deposited at the Muséum d’Histoire Naturelle, Paris, at the Palentological Institute, Moscow (with B. B. Rohdendorf and A. G. Sharov), and at the Museum of Natural History, London. My work in Canada was supported by the Canadian NSERC grant agency, and by Stewart B. Peck, Carleton University, Ottawa. The grants made it possible for me to study extensively Paleozoic insects in Russia, Germany, on many occasions at the Field Museum, Chicago (with E. S. Richardson) and in numerous private collections in the Chicago area. Fossils and rare primitive hemipteroids were researched at The Australian Museum, Sydney (Australia), and at the Natal Museum, Pietermaritzburg (South Africa). Modern insects were collected extensively in North, Central, and South America, including Alaska, Yukon, Tierra del Fuego, Galápagos Islands, Puerto Rico, Dominican Republic, Jamaica, Cuba, Lesser Antilles, South Africa and Ghana, all around Australia, New Zealand, New Caledonia, Fiji, Lord Howe Island, Japan, and China. I studied extensively in several months-long visits, modern Ephemeroptera at the Florida A&M University, Tallahassee (with J. & J. Peters) and at the Czech Academy of Sciences, České Budějovice (Czech Republic) (with T. Soldán), modern Coleoptera (with J. F. Lawrence), orthopteroids (with D. Rentz) at CSIRO, Canberra, and orthopteroids, blattoids and Dermaptera at the Academy of Sciences, Philadelphia (with D. Otte and F. Haas). Orthopteroids and tropical Hemipteroids were studied in Prague (with P. Štys), at INBIO, Santo Domingo, Costa Rica, and at the Territorial Museum, Darwin, peloridiids in Sydney (Australia) (with J. Evans), DSIR Auckland (New Zealand) (with W. Kuschel), and in the Bishop Museum, Honolulu (with S. Miller). At the Smithonian Institution, Washington, D.C., I studied Mecoptera and Trichoptera (with O. Flint), orthopteroids (with D. Nickles), and Carboniferous insects (with C. Labandeira). Plecoptera were researched at the Max Planck Institute, Schlitz, Germany (with P. Zwick), Embioptera at the California Academy of Sciences, San Francisco (with E.S. Ross), and Neuroptera and Megaloptera mainly in Ottawa (on material delivered by K. Lambkin). Hymenoptera were researched in the collection of Agriculture, Canada, Ottawa (with M. Sharkey and G. Gibson). My friend Jan G. Peters, Florida A&M University, Tallahassee devoted many hours to reviewing this manuscript. G. S. Ball and B. S. Heming, University of Alberta, Edmonton, R.G. Beutel, Friedrich Schiller Universität, Jena, and V.A. Grebenikov, Agriculture and Agri-Food Canada, Ottawa provided critical remarks and important pointers in contemporary systematic methods. From G. Bechly, Museum für Naturkunde, Stuttgart, I received useful suggestions clarifying some terms. I also received invaluable editorial help from B. Hallgrimsson, and from two reviewers who devoted generously their time to improve the presentation of arguments. To all these colleagues, co-authors and friends, and others too many to be named, goes my sincere gratitude and thanks.
Author information
Authors and Affiliations
Corresponding author
Rights and permissions
About this article
Cite this article
Kukalová-Peck, J. Phylogeny of Higher Taxa in Insecta: Finding Synapomorphies in the Extant Fauna and Separating Them from Homoplasies. Evol Biol 35, 4–51 (2008). https://doi.org/10.1007/s11692-007-9013-4
Received:
Accepted:
Published:
Issue Date:
DOI: https://doi.org/10.1007/s11692-007-9013-4