Abstract
In the context of global carbon cycle management, accurate knowledge of carbon content in forests is a relevant issue in contemporary forest ecology. We measured the above-ground and soil carbon pools in the dark-coniferous boreal taiga. We compared measured carbon pools to those calculated from the forest inventory records containing volume stock and species composition data. The inventory data heavily underestimated the pools in the study area (Stolby State Nature Reserve, central Krasnoyarsk Territory, Russian Federation). The carbon pool estimated from the forest inventory data varied from 25 (t ha−1) (low-density stands) to 73 (t ha−1) (highly stocked stands). Our estimates ranged from 59 (t ha−1) (low-density stands) to 147 (t ha−1) (highly stocked stands). Our values included living trees, standing deadwood, living cover, brushwood and litter. We found that the proportion of biomass carbon (living trees): soil carbon varied from 99:1 to 8:2 for fully stocked and low-density forest stands, respectively. This contradicts the common understanding that the biomass in the boreal forests represents only 16–20 % of the total carbon pool, with the balance being the soil carbon pool.
Similar content being viewed by others
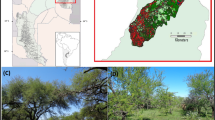
Explore related subjects
Discover the latest articles, news and stories from top researchers in related subjects.Avoid common mistakes on your manuscript.
Introduction
The last two decades in Earth sciences have been marked by the topics of global warming and global carbon cycle imbalances (IPCC 2013). The latter is often supposed to be the cause of the former, with the imbalance being understood mostly as an additional share of man-made carbon dioxide released into the atmosphere (IPCC 2001; Magnani et al. 2007). The primary source for the released excess carbon is attributed to fossil fuel combustion and ideas have been elaborated for possible sinks to offset excessive carbon accumulation in the atmosphere (McGuire et al. 2001; Scholze et al. 2003).
The most natural way to compensate for excess carbon emissions would be the storage of carbon in forest ecosystems, which includes both wood and soil, as well as in peat bogs (Alexeev and Berdsy 1994; Vasander and Kettunen 2006; Bazilevich and Titlyanova 2008).
Storage of excess carbon in forest biomass has been suggested as way to abate climatic changes attributed to increased atmospheric carbon dioxide (Shvidenko et al. 2000; Schimel et al. 2000; Schulze 2000; Pleshikov et al. 2002). Most of the expectations regarding carbon sequestration in forests are associated with cultivating of forest plantations which are often referred to as Kyoto forests (Roulet 2000). A possible basis for the expectations in the temperate zone is that as much as 56–65 % of the carbon stored in temperate regions is the result of changes in management, while the balance results from changes in land use (Sohngen and Sedjo 2006).
On the other hand, a vast area of boreal forest is forested land that, in a sense, cannot be managed; nor has there been a change of land use in most boreal forests. The cause of this is that the forests grow over large areas that are sparsely populated; they are also difficult to access so that commercial management for timber is not feasible. Large areas of boreal taiga distributed over northern Eurasia and Canada fall into this category.
While boreal forests are difficult to manage, they are still important components of the global carbon cycle even though their role is admittedly different from the function of forests growing in highly populated industrial lands. As reported by Sohngen and Sedjo (2006) there are two kinds of policies that can be implemented to reward carbon sequestering. The first, known as the rent policy, suggests rewarding the storing of carbon in the wood of trees, or in other words, the prevention of carbon release. The second, subsidy/taxation policy, provides rewards (subsidies) for carbon sequestration on, for example, an annual basis. Whatever policy is proposed, its implementation requires accurate biomass measurements.
There are many estimates of the carbon pools in Russian forests (e.g., Zamolodchikov et al. 2005). These authors addressed the continental level of the forest resource and their estimates were based on the rich database of the Russian Federal Forestry Agency. In particular, it has been estimated that Russian forests store about 218 × 109 tons of carbon, with 16 % of this amount being phytomass carbon and 84 % soil carbon (Utkin et al. 2001). Pan et al. (2011) investigated global carbon storages in tropical, boreal and temperate forests. They reported that the carbon stock densities in tropical and boreal forests are comparable, 242 and 239 (Mg ha−1), respectively, while the storage in temperate forest is lower at about 155 (Mg ha−1). They also reported that boreal forests stored 20 % of carbon in biomass and 60 % in soil.
There is no way to estimate carbon storage over huge forested areas other than to use the data of the periodic forestry inventories. This method however lacks accuracy and important detail: the more complicated the stand structure, the more detail drops out of the inventory. Thus the inventory data often need to be supplemented by more detailed field measurements, particularly in the case of native, highly uneven-aged mixed forests.
The aims of this study were (1) to measure in detail the storage of carbon in forest stands in eastern Siberian dark-coniferous taiga and (2) to compare carbon pool estimates based on our detailed field studies to those from the Russian Federal Forest Agency inventory data.
Materials and methods
Our study area was located in Stolby State Nature Reserve, established in 1925 and not subject to commercial forestry activity since then. The area is located on the right bank of Yenisey River in the vicinity of Krasnoyarsk city. Most of the Nature Reserve was covered by dark-coniferous low-mountain taiga. The most important dominant tree species included: Siberian fir (Abies sibirica Ledeb.), Siberian spruce (Picea obovata Ledeb.), Siberian pine (Pinus sibirica Du Tour), Scots pine (Pinus sylvestris L.), and Siberian larch (Larix sibirica Ledeb.). Soils were yellow–brown and brown light and heavy loams. The core rocks play the role of parent rocks only sporadically at well-drained elevations.
In 2007, the most recent forestry inventory was carried out in the Nature Reserve. In summer 2013, we performed a number of measurements on four sample plots with the goal of achieving a detailed estimation of stored carbon. Every plot was located within a forest inventory parcel. A summary description of the 2007 inventory data for the forest parcels is given in Table 1.
The sizes of plots #3, #4, #5 were 0.2, 0.21, 0.2 ha respectively, and the area of plot #6 was 0.01 ha. The plot coordinates were: #3 is E 92°51,869′N 55°52,174′; #4 is E 92°51,918′N 55°52,135′; #5 is E 92°50,267′N 55°50,673′; #6 is E 92°49,395′N 55°50,453′ (located by GPS).
In the sample plots, a number of measurements were recorded. For living trees (≥2 m height) and for standing deadwood we recorded species, DBH and height on a complete count basis. Species-specific formulae were then applied to estimate the volume of every bole measured. To calculate carbon content, we used coefficients for particular Siberian tree species from our previous study (Gavrikov and Khleboros 2013) (Table 2).
To estimate the carbon content of grass/moss cover, undergrowth, shrub layer and litter, we sampled three 2 × 20 m subplots in each sample plot. The exception was plot #6 where one subplot was sampled. For grass/moss cover, plants from 50 × 50 cm subplots were cut and weighed in the field, and subsamples of the grass, semishrubs and moss were taken to measure plant moisture in laboratory. A total of 30 50 × 50 cm subplots were sampled in each larger sample plot. To estimate the production of the undergrowth and the shrub layer, ten plants of both categories from each 2 × 20 m subplot were sampled and weighed in the field. We measured the height and diameter (projected cover) for each shrub and weight was measured for 10 specimens per subplot.
The 2 × 20 m subplots were divided into 2 × 2 m subplots and within the latter one 0.2 × 0.2 m sample of litter was cut out to measure litter oven-dry mass in the laboratory. To convert litter mass into carbon mass the factor 0.370 was used as recommended by IPCC.
On the same 2 × 20 m subplots, the stock of brushwood (deadwood lying on the ground) was measured. Within the subplots, all dead boles and stumps were counted and measured, and their volumes were calculated using geometric formulae for truncated cones. The class of wood decomposition was visually estimated for the measured pieces of brushwood. We used published density values for each decay class; for classes of decomposition I, II and III the wood density drops as 10, 30 and 50 % of the initial density, respectively (Klimchenko et al. 2011). Based on the recordings, the stocks of organic matter were calculated for the sample plots.
Mechanical (Particle-Size) Analysis (PSA) is one of the most often applied analyses in soil characterization because soil texture and the particle-size distribution can be related to many other soil properties. In our work, we used the Kachinsky pipette method (Kachinsky 1965). The proportion of rock fragments in soil horizons was estimated by volume. Bulk density was measured for mineral soil horizons (including humus and raw-humus horizons) with 50 cm3 metallic cylinders. For forest floor (organic horizons) and thin histic horizons we sampled using a 25 cm × 40 cm (0.1 m2) metallic frame. Soil sampling was performed in soil pits consecutively from every soil horizon.
Soil carbon was measured by the Tiurin wet oxidation method. Potassium dichromate was used in the presence of sulfuric acid as the oxidizer. The numbers of soil samples were 21, 15 and 15 for plots #3, #4 and #5, respectively. The soil was not sampled in plot #6.
Results and discussion
Most forest carbon estimates are based on the assumption that carbon content in wood is given by the factor 0.5 of the wood mass. From wood chemistry it is known, however, that this factor applies to the oven dry wood weight, and is not valid for trees standing in a forest. Gavrikov and Khleboros (2013) used data reported in the literature to develop a formula for the relationship of the volume of green wood of living trees to carbon mass for the five largest Siberian conifers (Table 2).
Taking into account these factors and the forest inventory data (Table 1) gives the opportunity to estimate the storage of carbon per ha for the parcels in the inventory (Table 3). In Table 3, the conversion factors were weighted by the relative proportions of species in the stand (Table 1) and based on the assumption that the forest included only conifers. Also, we assumed that the classes of decomposition for the standing deadwood and for the brushwood were I and II, respectively. Based on the forest inventory data, carbon storage varied between 50 and 70 tons C per ha for the fully stocked forest stands (parcels where sample plots #4–#6 were located) and was about 25 tons C per ha for the forest openings (parcel where sample plot #3 was located).
The carbon pools estimated based on our field measurements of the sample plots are given in Table 4. The same conversion factors were used in Tables 1 and 3. Also, as stated above, the standing deadwood and the brushwood were assumed to be of decomposition classes I and II, respectively.
Comparison of the results from the inventory data with those from field measurements revealed that the two methods corresponded regarding relative distribution of C pools among plots. Both showed minimal carbon storage at plot #3 and maximal carbon storage at plot #4.
In contrast, the inventory data appeared to severely underestimate carbon storage even within a storage pool, e.g. aboveground tree biomass. The field measurements coupled with other pools, including living cover and litter, showed twice as much stored carbon as predicted from the inventory data.
The estimated amounts of carbon in the study area soils are given in Table 5.
A comparison of the above-ground and soil carbon stocks showed that the proportion of biomass carbon (living trees): soil carbon varied for the sample plots from 99:1 to 8:2 for fully stocked and low-density forest stands, respectively.
The above estimates contradict with figures published in the literature. If one assumes a biomass carbon share of 20 % as reported by Pan et al. (2011) then the soil carbon stock in our plots would be four times larger than biomass carbon and would range from 34.7 × 4 = 138.8 to 135.9 × 4 = 541.6 tons per ha (Table 4), which is unrealistic.
The forest soils in our study area were similar in type and depth and, therefore, we assumed strong correlation between total organic carbon and soil carbon. A linear correlation of the correspondent values from Table 5 yields CS = 0.4129 × TOC — 0.0161, (R 2 = 0.9573), where CS is the carbon stock in tons per ha and TOC is total organic carbon as a percentage. The correlation means that even if the soil were pure carbon the carbon stock could not be larger than about 42 tons per ha.
A large body of literature has been devoted to the problems of quantifying the carbon cycle in terrestrial ecosystems and particularly in forest biotopes. The scope of the research encompasses both pools and fluxes of carbon but most interest has focused on carbon flux (Schulze et al. 1999; Magnani et al. 2007). This focus is explainable because the primary concern is to abate the growth of carbon input into the atmosphere, a flux process. Also, the highest expectations are linked to the cultivation of young forest stands since they are potentially large carbon sinks.
Yet attention has recently been drawn to the role of old-growth forests that continue to assimilate carbon (Luyssaert et al. 2008). We can add to this argumentation that boreal old-growth forests cover large areas in the northern hemisphere and represent large static (in the absence of catastrophic fires) pools of carbon. The data generated by forestry inventory may be insufficient to provide a realistic view of the current carbon pools in boreal forests. These pools should be more thoroughly estimated and measures are needed to prevent carbon emissions from them.
References
Alexeev VA, Berdsy RA (1994) Carbon in ecosystems of forests and peatlands of Russia. Publishing house of Institute of Forest of Siberian Branch of Russian Academy of Sciences, Krasnoyarsk, p 532 (in Russian)
Bazilevich NI, Titlyanova AA (2008) Biotic turnover on five continents: element exchange processes in terrestrial natural ecosystems. Publ. house SB RAS, Novosibirsk, p 380 (in Russian)
Gavrikov VL, Khleboros RG (2013) A conceptual model to analyze ecological and economical values of forest. Inzhenernaya Ecol 5:38–47 (in Russian)
IPCC (Intergovernmental Panel on Climate Change) (2001) Observed climate variability and change. In: Houghton JT, Ding Y, Griggs DJ, Noguer M, van der Linden PJ, Dai X, Maskell K, Johnson CA (eds) Climate Change 2001: the scientific basis. Contribution of Working Group I to the Third Assessment Report of the IPCC. Cambridge University Press, Cambridge, pp 99–181
IPCC (Intergovernmental Panel on Climate Change) (2013) Climate change 2013: the physical science basis. In: Stocker TF, Qin D, Plattner GK, Tignor M, Allen SK, Boschung J, Nauels A, Xia Y, Bex V, Midgley PM (eds) Contribution of Working Group I to the Fifth Assessment Report of the Intergovernmental Panel on Climate Change. Cambridge University Press, Cambridge, p 1535
Kachinsky NA (1965) Fizika Pochvy [Soil physics], vol I. Higher School Publishing, Moscow, p 323 (in Russian)
Klimchenko AV, Verlhovets SV, Slinkina OA, Koshurnikova NN (2011) The stock of large wood remains in middle-taiga ecosystems of Yenissei Siberia. Geografia i prirodnye resursy 2:91–97 (in Russian)
Luyssaert S, Schulze ED, Börner A, Knohl A, Hessenmöller D, Law BE et al (2008) Old-growth forests as global carbon sinks. Nature 455:213–215
Magnani F, Mencuccini M, Borghetti M, Berbigier P, Berninger F, Delzon S et al (2007) The human footprint in the carbon cycle of temperate and boreal forests. Nature 447:849–851
McGuire AD, Sitch S, Clein JS, Dargaville R, Esser G, Foley J, Heimann M, Joos F, Kaplan J, Kicklighter DW, Meier RA, Melillo JM, Moore B III, Prentice IC, Ramankutty N, Reichenau T, Schloss A, Tian H, Williams LJ, Wittenberg U (2001) Carbon balance of the terrestrial biosphere in the twentieth century: analysis of CO2, climate and land-use effects with four process-based models. Glob Biogeochem Cycles 15:183–206
Pan Y, Birdsey RA, Fang J, Houghton R, Kauppi PE, Kurz WA, Phillips OL, Shvidenko A, Lewis SL, Canadell JG, Ciais P, Jackson RB, Pacala SW, McGuire AD, Piao S, Rautiainen A, Sitch S, Hayes D (2011) A large and persistent carbon sink in the world’s forests. Science 333:988–993
Pleshikov FI, Kaplunov VJ, Tokmakov SV, Ben’kov AV, Titov SD, Pervunin VA (2002) Phytomass structure and annual production of boreal forests. In: Pleshikov FI (ed) Forest ecosystem of the Yenisey Meridian. Publishing house of SB RAS, Novosibirsk, pp 73–84 (in Russian)
Roulet NT (2000) Peatlands, carbon storage, greenhouse gases, and the Kyoto protocol: prospects and significance for Canada. Wetlands 20(4):605–615
Schimel DS, Melillo J, Tian H, McGuire AD, Kicklighter D, Kittel T, Rosenbloom N, Running S, Thornton P, Ojima D, Parton W, Kelly R, Sykes M, Neilson R, Rizzot B (2000) Contribution of increasing CO2 and climate to carbon stock by ecosystems in the United States. Science 287:2004–2006
Scholze M, Kaplan JO, Knorr W, Heimann M (2003) Climate and interannual variability of the atmosphere-biosphere 13CO2 flux. Geophys Res Lett 30(2):1097. doi:10.1029/2002GL015631
Schulze E-D (2000) Carbon and nitrogen cycling in European forest ecosystems. Springer, Berlin, p 500
Schulze E-D, Lloyd J, Kelliher FM, Wirth C, Rebmann C, Lühker B, Mund M, Knohl A, Milyukova IM, Schulze W, Ziegler W, Varlagin AB, Sogachev AF, Valentini R, Dore S, Grigoriev S, Kolle O, Panfyorov MI, Tchebakova N, Vygodskaya NN (1999) Productivity of forests in the Eurosiberian boreal region and their potential to act as a carbon sink—a synthesis. Glob Change Biol 5:703–722
Shvidenko AZ, Nilsson S, Stolbovoy VS, Gluck M, Shepacshenko DG, Rozchkov V (2000) Aggregated estimation of the basic parameters of biological productivity and carbon budget of Russian terrestrial ecosystems: 1. Stocks of organic mass. Russ J Ecol 6:403–410
Sohngen B, Sedjo R (2006) Carbon Sequestration in Global Forests Under Different Carbon Price Regimes. Energy J 27(Special issue 3):109–126
Utkin AI, Zamolodchikov DG, Chestnyh OV, Korovin GN, Zukert NV (2001) Russian forests as a reservoir of the organic carbon of biosphere. Lesovedenie 5:8–23 (in Russian)
Vasander H, Kettunen A (2006) Carbon in boreal peatlands. In: Wieder RK, Vitt DH (eds) Boreal peatland ecosystems. Ecological studies, vol 188. Springer, Berlin, pp 165–195
Zamolodchikov DG, Utkin AI, Korovin GN, Chestnykh OV (2005) Dynamics of carbon pools and fluxes in Russia’s forest lands. Russ J Ecol 36(5):291–301
Acknowledgments
The study was supported by the Russian Foundation for Basic Research, research grant 14-05-00831 ‘The landscape features and the integral assessment of carbon storing function in protected forest areas of Siberian southern taiga’. Authors are grateful to an anonymous reviewer whose comments helped to improve the manuscript. The authors declare that they have no conflict of interest.
Author information
Authors and Affiliations
Corresponding author
Additional information
Project funding: This research was supported by Russian Foundation for Basic Research, research grant 14-05-00831.
The online version is available at http://www.springerlink.com
Corresponding editor: Zhu Hong
Rights and permissions
About this article
Cite this article
Gavrikov, V.L., Sharafutdinov, R.A., Knorre, A.A. et al. How much carbon can the Siberian boreal taiga store: a case study of partitioning among the above-ground and soil pools. J. For. Res. 27, 907–912 (2016). https://doi.org/10.1007/s11676-015-0189-7
Received:
Accepted:
Published:
Issue Date:
DOI: https://doi.org/10.1007/s11676-015-0189-7