Abstract
Purpose
The drainage of a riparian peatland interspersed with organic and mineral substrates in Northern Germany has led to subsidence morphology due to mineralisation, initial settlement and shrinkage processes causing cultivation problems and yield declines. To explain the relevancy of shrinkage to changes of the surface morphology and soil properties, organic and mineral substrates were analysed with regard to (1) shrinkage potential (i.e. the maximum shrinkage), (2) shrinkage curve, (3) actual in situ shrinkage and (4) irreversible shrinkage proportions.
Materials and methods
The investigated substrates are sedge peat, peat-clay, half-bog and alluvial sand of Fibric Limnic Histosols (Drainic) and a glacial clay for comparison. The methods used to obtain the shrinkage curves include the consideration of crack formation due to desiccation, as digital photography and image analysis were used in addition to height change measurements. To characterise the actual in situ shrinkage, continuous records of the soil matric potential for a 4.5-year period at two different intensively drained Histosols (moderately and deeply drained: mean groundwater table 0.6 and 1.7 m under ground level) were accomplished in four depths (15, 40, 70, 100 cm).
Results
The measured shrinkage potentials vary between 2% (alluvial sand) and 65% (peat-clay). The sedge peat and peat-clay are characterised by distinct higher shrinkage potentials compared to the glacial clay and show distinct proportional shrinkage. It has to be pointed out that the soil volume loss partly even exceeds the loss in water volume. The sedge peat does not reach residual or zero shrinkage but shows distinct structural shrinkage at initial desiccation. For the moderately drained site, matric potentials reached up to −60 kPa in summer in 15-cm depth, whilst capillary rise almost prevented desiccation of deeper zones. For the deeply drained site, summer desiccation reached deeper zones as well (matric potential up to −50 kPa in 40-cm depth) due to absent capillary rise. In the range of actually occurring maximum desiccation, the half-bog and peat-clay with high decomposed organic matter are particularly affected by shrinkage with volume decreases of 10–14%, in contrast to the fibred sedge peat with only 4%. When uniquely shrunken substrates will be re-wetted, distinct irreversible shrinkage proportions reaching between 18% and 31% subsequent to drying to a matric potential of −50 kPa and re-saturation were measured.
Discussion
Particularly, the organic-rich substrates appeared as characteristically sensitive to soil shrinkage. Contrary to mineral substrates, they lack in solid particles, leading, along with high hydraulic stresses due to dominantly finer pores, to intense volume decreases after desiccation. These substrates therefore partly also lack in residual or zero shrinkage, and the soil volume loss can even exceed the water loss due to shrinkage. Accordingly, the alterations of soil properties, e.g. conductivities, are especially pronounced.
Conclusions
The calculated proportion of actual in situ shrinkage (0.5 to 1 dm) to total subsidence of the investigated peatland is rather low for the deeply drained site in comparison to initial settlement (2–3 dm) and long-term active mineralisation (e.g. 4 dm in 40 years, mineralisation rate 1 cm year−1) but accelerates the latter because of enhanced aeration due to crack formation. Irreversible shrinkage is one reason why re-wetted fen peats do not retrieve their initial volume as well as their initial physical and hydraulic properties. Thus, a re-wetting of drained fen peats can only preserve the present condition.
Recommendations and perspectives
To impede the further impairment of riparian peatlands, it is necessary to maintain a near-surface water table particularly throughout the summer months, when high desiccation occurs, leading to a spatially differentiated proportion of initial settlement, shrinkage and mineralisation, causing subsidence morphology. Our recommendation is a drainage depth of less than 60 cm to enable pasture or grassland land use and to minimise mineralisation as well as structure formation in the (sub)soil.
Similar content being viewed by others
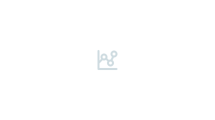
Avoid common mistakes on your manuscript.
1 Introduction
According to the draft of the European Soil Framework Directive (2006), soil organic matter decline is one of the main problems of soil degradation. The fen peat area of the most northern state of Germany, Schleswig-Holstein, aggregates to approximately 115,000 ha to this day. That corresponds roughly to 79% of the total peat area in Schleswig-Holstein and 7.3% of the state area, respectively. Between the 1950s and today, the peat area decreased by 11.5% (LANU 2002). Reasons for the reduction of the peat area are mineralisation subsequent to drainage and commercial peat cutover but also sedimentation due to water erosion (Richter et al. 2007). Approximately 87,000 ha fen peat area is under cultivation today, meaning that nearly 76% of the fen peat area of the state is agriculturally used and usually drained. Particularly in subareas of Schleswig-Holstein with poor fertile mineral soils, drained fen peats are important production locations for local farmers and are normally used as pasture or grassland.
As a result of drainage, different processes lead to volume changes within the peat: (1) initial settlement and subsequent shrinkage and (2) mineralisation. (1) Settlement is most active during the first years following drainage (Brandyk et al. 2002). The lowering of the groundwater table includes a loss of the buoyant force of water in the upper layers. Therefore, the peat layers below the phreatic zone have to bear an increased weight, as the weight of the overlying material is transferred from the liquid to the peat fibres, causing compression (Sauerbrey and Zeitz 1999). Additionally, higher desiccation intensities due to the lowering of the groundwater table and less capillary rise occur, leading to shrinkage processes (Brandyk et al. 2002; McLay et al. 1992; Oleszczuk et al. 2003; Schwärzel et al. 2002). (2) Typical mineralisation rates of drained fen peats in Northern Germany reach up to 1 cm year−1 (Göttlich 1990). The entry of air into the peat causes oxidation of the organic matter because of an increased activity of microorganisms. In drained fen peats, the release of nutrients, particularly nitrate in the water cycle, and carbon dioxide and nitrous oxide in the water cycle and the atmosphere is a pressing issue (Bonnett et al. 2006; Freibauer 2003; Kasimir-Klemedtsson et al. 1997; Scholz and Trepel 2004).
Peatlands often show subsidence morphology because of a spatially varying proportion of settlement, shrinkage and mineralisation, impairing tillage operations and causing yield losses. The emerging ground subsidence, resulting in an uneven soil surface, complicates the cultivation, as, besides difficulties concerning tillage operations, subareas will be provided differently with capillary raised groundwater. Moreover, compacted zones within the peat profile can develop and may be intensified after trafficking the particular unstable soils with agricultural machinery, leading to negative implications to soil hydraulic properties with the result of water logging or less capillary rise. The increases in bulk density as well as the alteration in pore structure of the peat directly impact the hydrological functioning of the peatland (Gebhardt et al. 2009; Schindler et al. 2003).
In order to maintain a worthwhile agricultural use of drained riparian peatlands, the role of shrinkage processes in dependence of water balance and occurring substrates taking place in addition to mineralisation and accelerating the latter due to structure formation and consequently enhanced aeration needs a better understanding. In this regard, it is essential to know the actual depth-dependent seasonal desiccation of the soils and the related shrinkage in order to find a drainage depth which is best suited to minimise detrimental effects caused by the named processes. The objective of this paper is to investigate the influence of shrinkage processes on the subsidence morphology of a drained riparian peatland. A characterisation of (1) shrinkage potential, (2) shrinkage curve, (3) actual in situ shrinkage and (4) irreversible shrinkage proportions of typical substrates was implemented. By means of measurements of the matric potential during a 4.5-year period at a moderately and deeply drained site, the depth-dependent actual shrinkage could be estimated.
2 Materials and methods
2.1 Site description
The study area is a drained riparian peatland situated in the North of Germany about 50 km northwest of Hamburg in the transition area of deposits of a Saalean terminal push moraine and the Lower Elbe depression (Fig. 1, left). The mean annual temperature is 7.8°C; the precipitation totals 998 mm year−1 with a reference evapotranspiration of 530 mm year−1, resulting in a distinct humid climate. Typical soils are Fibric and Limnic Histosols (Drainic) with thicknesses of up to 2 m (IUSS Working Group WRB 2007). A soil mapping of approximately 100 ha (186 bore holes) confirmed that the study area is in particular characterised by the appearance of different substrates. Peat-clays below or within the peat body, which developed in bodies of standing water of the formerly meandering ditches, and layers of sandy, partly organic-rich sediments, which were deposited during flooding, are characteristic. To enable agricultural grassland or pasture use, the groundwater in the study area was artificially lowered to mean surface distances between 50 and 200 cm by means of drainage since 1970 and river regulation. Due to the heterogeneity of the peat area with different organic and mineral substrates, settlement and shrinkage as well as mineralisation of the organic matter consequently led to the formation of subsidence morphology, representing a copy of the areal distribution of the different substrates (see Fig. 1, right). The emerging relief exhibits small-scale differences of the ground level partly exceeding 1 m. Local farmers complain about deficits in dry matter yield exceeding 20%.
2.2 Soil sampling and soil analyses
Soil sampling was conducted almost at water saturation in spring. The samples were almost unaffected by previous shrinkage as they were sampled in a depth of about 50 cm. Only the sedge peat had already experienced some shrinkage in the past. In total, 30 undisturbed soil core samples were collected vertically to characterise the shrinkage properties. Disturbed samples were used to measure particle density (pycnometer method); dry bulk density was determined gravimetrically by weighing the soil after drying at 105°C for 16 h (Blake 1986a, b). Organic carbon (Corg) was determined by dry combustion at 1,200°C and colorimetric CO2 measurement (Allison 1986). Soil texture was measured by the combined sieve (>63 µm) and pipette method (Gee and Bauder 1986); water retention characteristics were determined during the shrinkage measurements on ceramic suction plates and pressure chambers.
2.3 Soils
Fibric Limnic Histosols (Drainic) (IUSS Working Group WRB 2007) composed of sedge peat and further mineral, partly organic-rich, substrates were analysed concerning general soil properties and shrinkage behaviour: the substrates are (1) sedge peat, (2) peat-clay, (3) half-bog (an organic-rich substrate developed under gleyic conditions and having less than 20 vol.% Corg formed from alluvial sediment), (4) alluvial sand and furthermore glacial clay from a Planosol occurring in the adjacent terminal moraine (Table 1). Particularly in dependence of organic C, ranging from 265 g kg−1 in case of the peat to only 3.1 and 3.4 g kg−1 for the clay and sand, respectively, different particle densities between 1.71 and 2.63 g cm−3 and dry bulk densities between 0.20 and 1.30 g cm−3 were determined. The substrates have different textures ranging from sand to heavy clay (FAO 2006) and pore size distributions with high proportions of fine pores for the organic-rich substrates and the clay.
The scanning electron microscopy (SEM) features in Fig. 2 visualise the characteristic microstructure of the different substrates. In case of the sedge peat, the organic matter is less decomposed; porous and fibred plant structures are identifiable. The organic matter of the peat-clay and half-bog is more decomposed and sand-sized particles are observable. In case of the alluvial sand, consisting predominantly of rounded quartz particles, single-grain structure is typical. The illite-rich glacial clay forms a ‘chart house’ structure with numerous fine pores in between.
2.4 Soil shrinkage
Results from shrinkage measurements are commonly depicted as shrinkage curves, where the decrease in volume of water (moisture ratio, ϑ) is compared with the decrease in total soil volume (void ratio, e) by shrinkage (Bronswijk 1991b; Groenevelt and Grant 2004; Kim et al. 1992). Void ratio and moisture ratio are defined as ratios of pore or water volume to the volume of solid particles, respectively. Usually, four characteristic shrinkage zones can be distinguished, which indicate different structural rigidities of the soil as it desiccates (from wet to dry): structural, proportional (normal, basic), residual and zero shrinkage (Groenevelt and Grant 2001; McGarry and Malafant 1987; Mitchell 1992). During the start of desiccation, structural shrinkage takes place. Air enters large, water-filled pores causing minor volume changes. Ongoing desiccation leads to proportional shrinkage where smaller voids become drained. The capillary forces result in a considerable volume loss being proportional to the water loss. Intense desiccation may lead to a situation, where former shrinkage strongly increased the rigidity of the soil pore system and the additional volume loss becomes lower than the water loss (residual shrinkage) until at some point the soil volume does not change any more (zero shrinkage).
Because of the difficult shrinkage measurement process, a number of methods were developed and proved. Most commonly, these methods were based on measurements of shrinkage-related height changes of a soil sample, and isotropic assumption or vertical and horizontal deformations were considered individually (Boivin 2007; Braudeau et al. 1999; Bronswijk 1988, 1989, 1991a, b; Bronswijk and Eversvermeer 1990; Chertkov 2005; Chertkov et al. 2004; Garnier et al. 1997a, b; Oleszczuk et al. 2003). Most of these methods are only partly applicable for transferring the acquired shrinkage results from soil samples to natural, vertically and horizontally unbounded soils. Therefore, we used an approach, which is capable of considering crack formation as well (see below).
Shrinkage characteristics were determined using undisturbed soil core samples (v = 470 cm3). In total, 15 samples (three for each substrate) were saturated by capillary rise and drained to fixed matric potentials. Meanwhile, the samples were placed on a filter paper to ensure free shrinkage at the bottom. The used drainage steps were −3, −6, −15, −30 and −50 kPa with ceramic suction plates, 300, 600 and 1,500 kPa with pressure chambers and 30°C, 60°C und 105°C in the oven. After each drainage step, the weight of each sample as well as the volume decrease due to shrinkage was measured. The corresponding water contents, actual sample volumes and bulk densities were calculated and converted to the respective void ratios and corresponding moisture ratios by means of the measured particle densities. Finally, the shrinkage potentials (i.e. the maximum shrinkage from saturation to complete desiccation at 105°C) and the shrinkage curves were derived. In addition, 15 samples (three for each substrate) were drained to a soil matric potential of −50 kPa only and thereafter re-saturated completely to quantify irreversible shrinkage components.
After each drainage step, the vertical and horizontal shrinkage components were measured simultaneously as follows:
2.4.1 Soil vertical shrinkage
To account for potential differences in sample height, a digital calliper with an accuracy of 0.05 mm was used at eight fixed points at the sample surface to measure the sample height change. The total height change was then calculated from the arithmetic mean of these eight values. The change of sample height multiplied by the actual surface area gives the vertical volume change.
2.4.2 Soil horizontal shrinkage
By means of digital photography and image analysis, crack formation could be considered, whereby the surface crack area was used to calculate the total crack volume. The complex paraffin treatment proposed by Peng et al. (2006) was not applied, as they found that one can extrapolate from the crack surface to the crack volume quite well. Eventually, it is possible that, due to the stress distribution at the soil surface, occurring cracks taper into the soil, so that the exclusive registration of the crack surface using digital photography may lead to a slight overestimation of the crack volume (Peng et al. 2006).
The sample surfaces were uniformly illuminated with oblique incident light from a bright spotlight source so that occurring cracks were shaded. A digital camera was used with a tripod to record the soil surface after each drainage step from a constant distance directly above the sample. The resolution of the original digital colour images was 2,048 × 1,536 pixels. The pictures were then cut to soil core size, and the cracks were separated using Corel Photo-Paint software, which was admittedly time consuming, but, because of the high contrast between soil and crack area, the latter could be identified easily. The resulting greyscale pictures contained the separated cracks solely. Available methods for the evaluation of fractal dimensions, however, require the number of greyscale levels to be reduced to just two: black and white. The images therefore needed to be thresholded. Greyscale levels customarily range from 0 to 255 (i.e. there are 256 or 162 greyscale values in total). Various automatic thresholding algorithms may be used to generate binary images. This analysis was carried out with a threshold value of 127, meaning every pixel with a greyscale value less or equal to 127 becomes white (greyscale level is 0) and every value of more than 127 becomes black (greyscale level is 255). To account for thresholding problems (Baveye et al. 1998; Pendleton et al. 2005), the image resolution used was relatively high (1,180 × 1,180 pixels). The thresholding and the following adjustment of the picture scale and measure of the actual crack area were carried out with the public domain Scion Image software. Eventually, the horizontal volume change of the samples due to shrinkage could be calculated.
2.4.3 In situ soil volume changes
To assess seasonal variations of the investigated soils due to shrinkage, continuous time series of soil matric potential data for different depths (15, 40, 70 and 100 cm), which were recorded with tensiometers at two differently drained Histosols (mean groundwater tables 0.6 and 1.7 m below ground level) in the study area, were used. These data were related with the measured shrinkage properties of the different substrates. As a result, the actually occurring shrinkage intensity could be derived.
3 Results
3.1 Shrinkage potential
The substrates show different shrinkage potentials, i.e., maximum shrinkage-dependent volume decreases from saturation to complete desiccation, varying from 2.3% to 65.5% (Fig. 3). The shrinkage potential of the sand is lowest (corresponding to a small increase of dry bulk density from 1.30 to 1.33 g cm−3). Soil volume decreases of 50.4% for the sedge peat result in a doubling of the dry bulk density from 0.20 to 0.40 g cm−3, whilst the shrinkage potential amounts to 65.5% for the peat-clay (which almost is a triplication of the soil dry bulk density from 0.45 to 1.30 g cm−3), meaning that the shrinkage potential of these organic-rich substrates even exceeds that of the glacial clay with a clay content of more than 70%. The latter reaches maximum volume decreases of 33.1% (corresponding to an increase of the dry bulk density from 1.21 to 1.81 g cm−3), whilst the volume of the half-bog is reduced at least by 19.1% (increase in dry bulk density from 0.92 to 1.15 g cm−3).
3.2 Shrinkage curves
Individual differences already become obvious due to the differences of the characteristic void ratios (e) and moisture ratios (ϑ) between approximately 8.0 and 1.2 cm3 cm−3 under saturated conditions (Fig. 4; alluvial sand with low shrinkage potential is not considered). Distinct structural shrinkage (up to ϑ = 4) shows the sedge peat, which subsequently proceeds to proportional shrinkage. The soil volume loss partly even exceeds the loss in water volume, and even at high desiccation intensities this substrate does not reach residual or zero shrinkage. Despite differences concerning maximum shrinkage (see Fig. 3), comparable shrinkage curves are distinguished in the case of the peat-clay and the glacial clay. Both substrates merely show small structural shrinkage components, pronounced proportional shrinkage near the saturation line, where even in case of the peat-clay a tendency towards a stronger volume in proportion to water loss becomes obvious, and residual shrinkage occurs at high desiccation intensities likewise. The half-bog exhibits low structural and proportional shrinkage and already proceeds to residual shrinkage at approximately ϑ = 1.
3.3 Actual in situ shrinkage
In dependence of the drainage depth, the matric potential distributions show remarkable differences (Fig. 5, left). Maximum desiccation in the topsoil (15-cm depth) reached −60 kPa for the moderately drained and more than −70 kPa (where the measurement range becomes limiting) for the deeply drained site. In case of the latter, where re-wetting in summer only takes place due to precipitation, the subsoil (40 cm depth) attains up to −50 kPa. As a result of temporary capillary rise, the subsoil shows lower desiccation for the moderately (40-cm depth) and deeply drained site (70 cm depth), remaining below a matric potential of −20 kPa even in summer.
Course of soil matric potential at four different depths for a 4.5-year period for a moderately (top left; a) and deeply drained (bottom left, b) site monitored in the investigated riparian peatland. Course of soil volume change in dependence of matric potential for four substrates of Histosols and a glacial clay for comparison and enlarged section showing the maximum measured desiccation for both sites (a, b) and two depths (1, 2) and accordant shrinkage intensities (right)
The comparison with the recorded matric potentials for the moderately and deeply drained site proves that only a small range of the shrinkage potential will be reached during the year (see Fig. 5, right). In the enlarged section, the maximum measured matric potentials at both monitored sites in 15 and 40 cm are depicted (a1–b2). It shows that especially the peat-clay (14%) and the half-bog (10%) are affected by distinct volume decreases for the actually occurring matric potentials, whilst the fibred sedge peat (4%) shows only low actual shrinkage despite a high shrinkage potential.
3.4 Irreversible shrinkage components
Initially, the samples were drained to a matric potential of −50 kPa, which roughly corresponds to the maximum drying intensity of the deeply drained site in 40-cm soil depth (see Fig. 5). Volume decreases due to shrinkage range between 4% and 14%, with highest values in case of the peat-clay and lowest for the sedge peat (Fig. 6). Afterwards, the samples were re-saturated completely. As a result, none of the investigated substrates regained its initial volume. The proportion of re-swelling to total shrinkage was between 69% and 82%, i.e. the irreversible shrinkage proportion amounted between 18% and 31%.
4 Discussion
The substrates of the Histosols show very low to very high shrinkage potentials. Particularly, soils, which have high contents of organic matter, are characterised by extremely high shrinkage. Apparently, the organic matter represents an especially unstable element in the drying soil because of its lacking rigidity. Brandyk et al. (2002) mention largest shrinkage for sedge and alder peat material and lowest for moss peat. In our studies, the shrinkage potential of the peat-clay even exceeds that of the sedge peat. Yet, the half-bog shows higher shrinkage at the actually occurring desiccation but smaller maximum shrinkage. In general, shrinkage seems to increase with primary degree of decomposition (e.g. peat-clay > sedge peat) and decreases with ash content (Brandyk et al. 2002; Päivänen 1982 in Schwärzel et al. 2002). The ascertained moisture retention characteristics furthermore indicate that these organic soils comprise a relatively high fraction of fine pores (e.g. 45 vol.% for the sedge peat and 66 vol.% for the peat-clay), which causes high capillary forces. Thus, internal tensions at appropriate desiccation levels result in considerable volume decreases. During continuous dehydration, the decrease in soil volume even exceeds the water loss in case of the sedge peat and the peat-clay. These results are similar to those presented by Oleszczuk et al. (2003) for peat, although this phenomenon does not appear at very high suctions here. Contrary to the clay, the sedge peat does not include all four shrinkage zones but just structural and proportional shrinkage. McLay et al. (1992) explain the absence of a residual zone by the lack of sand and silt particles to form a rigid structure. On the one hand, the rigidity of the pore system, which depends on particle size and grading and type of particle (mineral, organic), as well as bulk density, and on the other hand the hydraulic stress, which mainly depends on pore size distribution, are of importance. Both interact in an oppositional manner, vary their characteristic value in the course of desiccation and shrinkage and result in different, distinctive shrinkage curves depending on relative strength.
Schothorst (1977) already investigated surface subsidence after lowering the ditchwater levels in a fen peat. Over a 6-year period, the surface subsided 6 to 10 cm, and 65% of it was caused by shrinkage and oxidation of organic matter above the groundwater table and 35% by compression of the layer below the groundwater table. Schlotzhauer and Price (1999) report settlement ranging from 11% to 23% of the lowering of the water table in a managed cutover peat. They showed that the resulting surface subsidence is partly due to shrinkage above the water table and partly due to compression of the saturated peat below the water table. In case of the genetically heterogenic occurrence of different substrates in the studied riparian peatland small-scale differences of the named processes due to widely varying subsidence, shrinkage and mineralisation properties take place. The arising subsidence morphology is all the more pronounced the deeper and longer the peatland was drained, i.e. how often intensive and deep summer desiccation takes place. In the range of matric potentials actually occurring in the study area, the peat-clay appeared particularly sensitive. Volume decreases of almost 15% may be expected by shrinkage solely. Considerably higher desiccation intensities and hence shrinkage-dependent volume changes may appear near the soil surface though since peats exposed freely to the solar radiation can heat up to 60°C in summer. However, the calculated proportion of actual in situ shrinkage (0.5 to 1 dm) to total subsidence for the deeply drained site is rather low in comparison to the initial settlement (2–3 dm) and long-term active mineralisation (e.g. 4 dm in 40 years, assuming a mineralisation rate of 1 cm year−1); even so, the effect on physical and hydraulic soil properties is high. Desiccation causes shrinkage, concomitant crack and structure formation and enhanced mineralisation of the organic matter due to continuing aeration. An increase of the saturated hydraulic conductivity is reported (e.g. Hennings 1996; Sauerbrey and Zeitz 1999; Zeitz and Velty 2002). Changing pore structure also changes the unsaturated hydraulic conductivity function (Brandyk et al. 2002; Kennedy and Price 2005) and the moisture retention of peat, often affected by distinct water repellency (Brandyk et al. 2002; Dekker and Ritsema 1996; Schwärzel et al. 2002).
The data could show that, even in initially shrunken soils, only a certain part of shrinkage is reversible. The more often such shrink–swell cycles occur, the more the soil volume will gradually shrink with decreasing intensity until the soil at some point is completely adapted to the new stress state (Peng et al. 2007). In the course of the year, several desiccation and re-wetting cycles naturally occur. That is the reason why it is difficult to implement shrinkage characteristics of soils in hydraulic models to enable a better specification of transient hydraulic properties (Camporese et al. 2005, 2006; Kennedy and Price 2004, 2005; Smiles 2000). As soon as a new initial shrinkage state occurs, the shrinkage characteristic of the soil itself changes, and the ‘old’ shrinkage characterisation and consequently also the derived hydraulic properties no longer apply (Baumgartl and Horn 1999). Moreover, hydrophobicity is to be expected at higher desiccation intensities in organic soils with corresponding anisotropy between shrinkage and swelling, being more pronounced in organic than in mineral soils (Peng and Horn 2007).
5 Conclusions
The development of the subsidence morphology in the investigated drained riparian peatland is, in addition to initial settlement and mineralisation, the result of shrinkage. In this regard, it is not the shrinkage potential but rather the actual shrinkage, depending on drainage depth and maximum desiccation intensity, respectively, that is of importance. By using hydraulic models, e.g. HYDRUS, it is possible to predict critical matric potentials relating to structure formation as a function of drainage depth. In the study area, the proportion of actual in situ shrinkage to total subsidence is lower in comparison to initial settlement and long-term active mineralisation but definitely accelerates the latter because of enhanced aeration due to crack formation. The arising soil structure is a considerable factor making a re-wetting of drained fen peats difficult, as the initial soil volume as well as the natural physical and hydraulic properties of the organic substrates will not be regained. Any desiccation being higher than the previously highest one moreover leads to irreversible shrinkage components. Nevertheless, a targeted re-wetting is able to impede the ongoing formation of small-scale ground subsidence as well as mineralisation and shrinkage of organic substrates and consequently further impairment of soil properties.
6 Recommendations and perspectives
To alleviate the negative consequences of drainage in peatlands, the groundwater table has to be maintained near the soil surface particularly throughout the summer months to prevent considerable desiccation of the upper peat layers and to reduce substrate-specific shrinkage processes and structure formation, concomitant aeration and decomposition, hydrophobicity as well as changes of soil hydraulic properties. Particularly, riparian peatlands, which consist of different substrates leading to spatially differentiated proportions of settlement, shrinkage and mineralisation, are sensitive. Our recommendation is a drainage depth of less than 60 cm to enable pasture or grassland use and to minimise mineralisation and structure formation in the (sub)soil. Because these processes are (mostly) irreversible, the physical and hydraulic properties of former deeply drained sites do not recover as a result of re-wetting.
References
Allison LE (1986) Organic carbon. In: Klute A (ed) Methods of soil analysis: part 1. American Society of Agronomy, Madison, pp 1367–1378
Baumgartl T, Horn R (1999) Influence of mechanical and hydraulic stresses on hydraulic properties of swelling soils. In: VanGenuchten MT, Leij FJ (eds) Characterization and measurement of the hydraulic properties of un-saturated porous media. University of California, Riverside, pp 449–458
Baveye P, Boast CW, Ogawa S, Parlange JY, Steenhuis T (1998) Influence of image resolution and thresholding on the apparent mass fractal characteristics of preferential flow patterns in field soils. Water Resour Res 34(11):2783–2796
Blake GR (1986a) Bulk density. In: Klute A (ed) Methods of soil analysis: part 1. American Society of Agronomy, Madison, pp 374–390
Blake GR (1986b) Particle density. In: Klute A (ed) Methods of soil analysis: part 1. American Society of Agronomy, Madison, pp 371–373
Boivin P (2007) Anisotropy, cracking, and shrinkage of vertisol samples—experimental study and shrinkage modeling. Geoderma 138(1–2):25–38
Bonnett SAF, Ostle N, Freeman C (2006) Seasonal variations in decomposition processes in a valley-bottom riparian peatland. Sci Total Environ 370(2–3):561–573
Brandyk T, Szatylowicz J, Oleszczuk R, Gnatowski T (2002) Water-related physical attributes of organic soils. In: Parent LE, Ilnicki P (eds) Organic soils and peat materials for sustainable agriculture. CRC, Boca Raton, pp 33–62
Braudeau E, Costantini JM, Bellier G, Colleuille H (1999) New device and method for soil shrinkage curve measurement and characterization. Soil Sci Soc Am J 63(3):525–535
Bronswijk JJB (1988) Modeling of water-balance, cracking and subsidence of clay soils. J Hydrol 97(3–4):199–212
Bronswijk JJB (1989) Prediction of actual cracking and subsidence in clay soils. Soil Sci 148(2):87–93
Bronswijk JJB (1991a) Drying, cracking, and subsidence of a clay soil in a Lysimeter. Soil Sci 152(2):92–99
Bronswijk JJB (1991b) Relation between vertical soil movements and water-content changes in cracking clays. Soil Sci Soc Am J 55(5):1220–1226
Bronswijk JJB, Eversvermeer JJ (1990) Shrinkage of Dutch clay soil aggregates. Neth J Agr Sci 38(2):175–194
Camporese M, Putti M, Salandin P, Teatini P (2005) Modeling swelling/shrinkage behaviour of peat soils. Geophysical Research Abstracts 7(07991)
Camporese M, Ferraris S, Putti M, Salandin P, Teatini P (2006) Hydrological modeling in swelling/shrinking peat soils. Water Resour Res 42:W06420. doi:10.1029/2005WR004495
Chertkov VY (2005) The shrinkage geometry factor of a soil layer. Soil Sci Soc Am J 69(6):1671–1683
Chertkov VY, Ravina I, Zadoenko V (2004) An approach for estimating the shrinkage geometry factor at a moisture content. Soil Sci Soc Am J 68(6):1807–1817
Dekker LW, Ritsema CJ (1996) Variation in water content and wetting patterns in Dutch water repellent peaty clay and clayey peat soils. Catena 28(1–2):89–105
European Soil Framework Directive (2006) Proposal for a directive of the European parliament and of the council establishing a framework for the protection of soil and amending directive 2004/35/EC. p 30
FAO (2006) Guidelines for soil description, 4th edn. FAO, Rome, p 110
Freibauer A (2003) Regionalised inventory of biogenic greenhouse gas emissions from European agriculture. Eur J Agron 19(2):135–160
Garnier P, Perrier E, Jaramillo RA, Baveye P (1997a) Numerical model of 3-dimensional anisotropic deformation and 1-dimensional water flow in swelling soils. Soil Sci 162(6):410–420
Garnier P, Rieu M, Boivin P, Vauclin M, Baveye P (1997b) Determining the hydraulic properties of a swelling soil from a transient evaporation experiment. Soil Sci Soc Am J 61(6):1555–1563
Gebhardt S, Fleige H, Horn R (2009) Effect of compaction on pore functions of soils in a North German Saalean moraine landscape. J Plant Nutr Soil Sci . doi:10.1002/jpln.200800073
Gee GW, Bauder JW (1986) Particle-size analysis. In: Klute A (ed) Methods of soil analysis: part 1. American Society of Agronomy, Madison, pp 383–411
Göttlich K (1990) Moor- und Torfkunde. 3. vollst. überarb. und erw. Aufl., Stuttgart
Groenevelt PH, Grant CD (2001) Re-evaluation of the structural properties of some British swelling soils. Eur J Soil Sci 52(3):469–477
Groenevelt PH, Grant CD (2004) Analysis of soil shrinkage data. Soil Till Res 79(1):71–77
Hennings HH (1996) Zur Wiedervernässung von Niedermoorböden. Dissertation, Georg-August-Universität, Göttingen
IUSS Working Group WRB (2007) World reference base for soil resources 2006, first update 2007. In: World Soil Resources Reports No. 103. FAO, Rome
Kasimir-Klemedtsson Å, Klemedtsson L, Berglund K, Martikainen P, Silvola J, Oenema O (1997) Greenhouse gas emissions from farmed organic soils: a review. Soil Use Manage 13(4):245–250
Kennedy GW, Price JS (2004) Simulating soil water dynamics in a cutover bog. Water Resour Res 40(12):1–13
Kennedy GW, Price JS (2005) A conceptual model of volume-change controls on the hydrology of cutover peats. J Hydrol 302(1–4):13–27
Kim DJ, Vereecken H, Feyen J, Boels D, Bronswijk JJB (1992) On the characterization of properties of an unripe marine clay soil.1. shrinkage processes of an unripe marine clay soil in relation to physical ripening. Soil Sci 153(6):471–481
LANU (2002) Programm zur Wiedervernässung von Niedermooren. Landesamt fuer Natur und Umwelt, Schleswig-Holstein
McGarry D, Malafant KWJ (1987) The analysis of volume change in un-confined units of soil. Soil Sci Soc Am J 51(2):290–297
McLay CDA, Allbrook RF, Thompson K (1992) Effect of development and cultivation on physical-properties of peat soils in New-Zealand. Geoderma 54(1–4):23–37
Mitchell AR (1992) Shrinkage terminology—escape from normalcy. Soil Sci Soc Am J 56(3):993–994
Oleszczuk R, Bohne K, Szatylowicz J, Brandyk T, Gnatowski T (2003) Influence of load on shrinkage behavior of peat soils. J Plant Nutr Soil Sci 166(2):220–224
Pendleton DE, Dathe A, Baveye P (2005) Influence of image resolution and evaluation algorithm on estimates of the lacunarity of porous media. Phys Rev E 72(1):041306.1–041306.9
Peng X, Horn R (2007) Anisotropic shrinkage and swelling of some organic and inorganic soils. Eur J Soil Sci 58(1):98–107
Peng X, Horn R, Peth S, Smucker A (2006) Quantification of soil shrinkage in 2D by digital image processing of soil surface. Soil Till Res 91(1–2):173–180
Peng X, Horn R, Smucker A (2007) Pore shrinkage dependency of inorganic and organic soils on wetting and drying cycles. Soil Sci Soc Am J 71(4):1095–1104
Richter FH, Fleige H, Blume HP, Horn R (2007) Bodengesellschaften von geomorphen Einheiten unter Berücksichtigung des Gefügestils im Stormaner Jungmoränengebiet (Brandenburger Stadium) in Nordwestdeutschland. J Plant Nutr Soil Sci 170:682–692
Sauerbrey R, Zeitz J (1999) Moore. In: Blume HP (ed) Handbuch der Bodenkunde. Ecomed, Landsberg
Schindler U, Mueller L, Behrendt A (2003) Field investigations of soil hydrological properties of fen soils in North-East Germany. J Plant Nutr Soil Sci 166(3):364–369
Schlotzhauer SM, Price JS (1999) Soil water flow dynamics in a managed cutover peat field, Quebec: field and laboratory investigations. Water Resour Res 35(12):3675–3683
Scholz M, Trepel M (2004) Water quality characteristics of vegetated groundwater-fed ditches in a riparian peatland. Sci Total Environ 332(1–3):109–122
Schothorst CJ (1977) Subsidence of low moor peat soils in western Netherlands. Geoderma 17(4):265–291
Schwärzel K, Renger M, Sauerbrey R, Wessolek G (2002) Soil physical characteristics of peat soils. J Plant Nutr Soil Sci 165(4):479–486
Smiles DE (2000) Hydrology of swelling soils: a review. Aust J Soil Res 38(3):501–521
Zeitz J, Velty S (2002) Soil properties of drained and rewetted fen soils. J Plant Nutr Soil Sci 165(5):618–626
Author information
Authors and Affiliations
Corresponding author
Additional information
Responsible editor: Winfried Blum.
Rights and permissions
About this article
Cite this article
Gebhardt, S., Fleige, H. & Horn, R. Shrinkage processes of a drained riparian peatland with subsidence morphology. J Soils Sediments 10, 484–493 (2010). https://doi.org/10.1007/s11368-009-0130-9
Received:
Accepted:
Published:
Issue Date:
DOI: https://doi.org/10.1007/s11368-009-0130-9