Abstract
Under natural conditions, the dissolved inorganic carbon (DIC) in river water is dominantly derived from carbonate or silicate dissolution by carbonic acid. However, sulfuric and nitric acids produced by human activities provide additional acidity for chemical weathering, which would affect the DIC flux and change its isotopic composition. To identify the natural and anthropogenic impacts on DIC, the major ion concentrations and stable carbon isotopes of the DIC (δ13C-DIC) of river waters were measured in the Pearl River Delta (PRD) region, which is one of the most developed and populated areas in China. The mass balance calculations for DIC-apportionment showed that carbonate dissolution by carbonic acid was the dominant origin of DIC in the Beijiang (BJ) River (67%) and Xijiang (XJ) River (78%) and silicate dissolution by carbonic acid was the dominant origin of DIC in the Guangzhou (GZ) Channel (37%) and Dongjiang (DJ) River (50%), which was related to the lithology of the catchment. The contribution of carbonate dissolution by sulfuric and nitric acids, which represented the contribution of human activities to the total DIC concentrations in river water, showed high proportions in the GZ Channel and DJ River, with averages of 42% and 34%, respectively, which were associated with a high degree of urbanization. Evidence of hydrochemical parameters and δ13C-DIC signatures indicated that human activities had impacts on the DIC pool. Carbonate dissolution by sulfuric and nitric acids caused by human activities changed DIC apportionments rather than the DIC flux, and this part of DIC would ultimately become a source of CO2 to the atmosphere on the geological timescale and affects the CO2 budget. An increase in nutrient concentration due to increased sewage discharge in the urbanized area could promote phytoplankton photosynthesis, which could change the DIC pool and increase the δ13C-DIC value. This study quantitatively highlights the influence of human activities on DIC apportionment in river water, suggesting that anthropogenic impacts should be seriously considered when evaluating the evolution of DIC.
Similar content being viewed by others
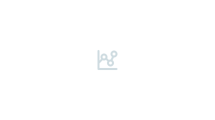
Explore related subjects
Discover the latest articles, news and stories from top researchers in related subjects.Avoid common mistakes on your manuscript.
Introduction
Chemical weathering of rocks driven by carbonic acid that originates from CO2 in the atmosphere or soil respiration under natural conditions is a fundamental geochemical process that links geochemical cycling of land to the atmosphere and ocean (Guo et al. 2015; Liu et al. 2018a), which regulates Earth’s climate and can convert atmospheric/soil CO2 into dissolved inorganic carbon (DIC) (Li et al. 2008; Murphy et al. 2016). This DIC is transported by rivers or groundwater and is thereafter precipitated in the ocean as carbonate minerals (Jiang 2013; Li et al. 2008). Carbonate and silicate weathering driven by carbonic acid are recognized as two typical categories of chemical weathering. Carbonate dissolution driven by carbonic acid can cause the accumulation or depletion of CO2 on short timescales, and the CO2 consumption on the continents is balanced by the flux of CO2 released into the atmosphere from the oceans by carbonate precipitation on the geological timescale (Berner and Kothavala 2001). The consumption of CO2 during silicate dissolution driven by carbonic acid has been regarded as the net sink of CO2 and regulates the global carbon cycle on the geological timescale (Hartmann et al. 2014; Lerman and Wu 2006). Although carbonate and silicate dissolution by carbonic acid are dominant weathering processes in a carbonate-silicate mixing ecosystem, other acids (such as sulfuric and nitric acids) of anthropogenic origins also provide the required acidity and become important chemical weathering agents (Ali and Atekwana 2011; Barnes and Raymond 2009; Cartwright 2010; Cuoco et al. 2017; Gandois et al. 2011; Raymond et al. 2008; Spence and Telmer 2005; Xia et al. 2017). In these cases, carbonate dissolution driven by sulfuric and nitric acids may result in increased DIC export in agricultural or urban areas (Jiang 2013) and become a source of CO2 to the atmosphere on the geological timescale, which plays a crucial role in balancing the CO2 budget (Li et al. 2008). Thus, under the influence of human activities, carbonate dissolution by the combination of carbonic, sulfuric, and nitric acids and silicate dissolution by carbonic acid control the net sink of atmospheric CO2, and it is essential to distinguish the contributions of these processes to DIC.
River have been recognized as an important pathway for the transport of DIC from land to the oceans. However, rivers are not passive transport agents of DIC produced by weathering processes to the oceans (Wachniew 2006) but are open systems linked to terrestrial ecosystems, groundwater, and the atmosphere (Michel 2003). Thus, biogeochemical processes occur are intertwined with physical processes in rivers, which results in a redistribution of carbon (Cuoco et al. 2017; Li et al. 2019; Yang et al. 2016). For example, DIC may be assimilated by primary producers and is incorporated into a complex aquatic food web, which involves the production and consumption of different forms of particulate or dissolved organic carbon (Gao and Wang 2015; Zavadlav et al. 2013). The DIC pool is also replenished by respiration from bacterial activities (Peter and James 2000) and photodissociation of auto- and allochthonous organic matter (Wachniew 2006). In addition, human activities such as the development of agriculture and industry and the increase in urbanization also affect riverine carbon cycling (Barnes and Raymond 2009). For example, photosynthesis and respiration processes are intensified by increases in nutrients and organic carbon caused by wastewaters and agricultural runoff inputs in rivers (Wachniew 2006). The export of DIC may increase due to urban and agricultural activities, which is related to enhanced CO2 production by organic matter due to land disturbance activities (Barnes and Raymond 2009). Thus, it is important to identify the carbon cycles in rivers and understand how these biogeochemical processes, especially the processes affected by human perturbations, function in current weathering systems.
In aquatic ecosystems, the stable carbon isotopic composition of DIC (δ13C-DIC) can reflect the integrated influence of biogeochemical processes in the water, including different sources, primary production, and carbonate chemistry (Niinikoski and Karhu 2017; Samanta et al. 2015; Schulte et al. 2011). Both the sources and internal biogeochemical processes of DIC can leave distinguishable traces in the stable carbon isotopic composition of DIC. For example, the δ13C values for most carbonates range from − 3 to + 2‰ (Telmer and Veizer 1999). The δ13C of atmospheric CO2 ranges from − 6 to − 8‰ with an average of − 7‰ (Levin et al. 1995), and the fractionation during dissolution of CO2 ranges from 9.2 ± 0.4‰ at 0 °C to 6.8 ± 0.4‰ at 30 °C (Halas et al. 1997). The δ13C of soil CO2, however, has a wide range (− 23 ~ − 13‰), which is inherited from decaying organic matter and depends largely on the photosynthetic pathway of vegetation (Vogel 1993). In addition, biogeochemical processes of DIC in the waters, such as photosynthesis and respiration, are also recorded in the δ13C-DIC signatures (Dubois et al. 2010; Schulte et al. 2011). Thus, δ13C-DIC is a valuable tracer in tracking sources of DIC and in understanding C cycling in aquatic ecosystems.
The Pearl River is the second largest river in China in terms of discharge volume and is characterized by a mixture of carbonate-silicate rocks in lithology. Over the past three decades, the Pearl River basin has undergone rapid urbanization and extensive agricultural development (Strokal et al. 2015; Zhang et al. 2007), resulting in a great influence of human activities on chemical weathering and the hydrochemical composition in the river water. The Pearl River Delta (PRD) region is located downstream of the Pearl River and is characterized by high levels of urbanization, dense populations, and extensive economic development (Hu and Li 2009; Lu et al. 2009). Thus, the hydrochemical composition of the PRD region can reflect both rock weathering processes and human activities affecting the water chemistry and biogeochemical processes of DIC. Therefore, in this study, the major physical-chemical parameters and the carbon isotope composition of river water in the PRD region were investigated to (1) identify the mechanisms controlling the major ion chemistry, (2) quantify the contributions of natural weathering processes and human activities to DIC, and (3) discuss the anthropogenic impacts on the DIC pool by δ13C-DIC signatures.
Materials and methods
Study area
The PRD region is located in the subtropical area of South China and receives riverine inputs from the Pearl River, which consists of three major tributaries: the Dongjiang (DJ) River, Beijiang (BJ) River, and Xijiang (XJ) River (Fig. 1). The DJ River is characterized as the silicate-dominated area (Xie et al. 2013), the XJ River has carbonate as the main rock type (Gao and Wang 2015), and the BJ River is characterized as a typical mixed carbonate-silicate basin (Xuan et al. 2018). The PRD region has a monsoon climate with an average annual temperature between 20 and 25 °C and an average annual precipitation between 1600 and 2000 mm (Du et al. 2015; Zhou et al. 2011). The warm and wet climatic conditions make the PRD a hyperactive region in China. In this region, physical and biogeochemical processes show seasonal variations due to the regular variations between warm and humid monsoons in summer and cool and dry monsoons in winter (Ye et al. 2017; Ye et al. 2016). During the wet season (April to September), large nutrient and solute loadings are transported from the upper stream to the PRD region due to high precipitation and runoff (Ou et al. 2019; Yu et al. 2010, 2015). During the dry season (October to March), the water column is well mixed, resulting in intense physical processes due to the effect of reduced river discharge (Ye et al. 2017).
The Pearl River drains an area of 453,700 km2. Over the past three decades, the Pearl River basin has experienced rapid urbanization and extensive agricultural development due to the Reform and Opening Up stage in China (Liu et al. 2018b; Xu et al. 2019). The dominant land uses in the upper drainage basin are forestry and agriculture, with the remainder used for urban areas or barren land (Xuan et al. 2018). The downstream area, which mainly consists of the PRD region, is dominated by agricultural land and urban and industrial use (Du et al. 2015). In addition, the PRD region is surrounded by a number of large metropolises, such as Guangzhou, Shenzhen, and Dongguan (Fig. 1). Therefore, in the PRD region, river water receives urban runoff, sewage inputs, and agricultural discharge from the upper stream and surrounding areas, which leads to a profound effect on the chemistry and biology of the river ecosystem.
Sample collection
Based on the precipitation and discharge, river water samples were collected in the PRD during the dry season in March 2018 and wet season in September 2018. The sampling sites are shown in Fig. 1. The total number of river water samples was 116, including 58 samples in the wet season and 58 samples in the dry season. Specifically, in the PRD region, river water samples were collected from 14 sites in the Guangzhou (GZ) Channel, 15 sites in the DJ River, 13 sites in the BJ River, and 16 sites in the XJ River (Fig. 1).
At each sampling site, surface water samples (approximately 0.5 m below the surface) were collected with a water-sampling bottle (2 L). Water samples for δ13CDIC analysis were collected in 50 ml brown glass vials, and saturated HgCl2 was added to prevent biological activity. Samples for major element analysis were filtered through a 0.45-μm glass fiber filter and stored in 100-ml tubes. All the water samples were stored below 4 °C until analysis.
Analysis method
Field parameters for river water samples including temperature (T), electric conductivity (EC), pH, and dissolved oxygen (DO) concentration were measured using a multi-parameter water quality meter (HACH-HQ40Q), and alkalinity of filtered water samples was measured by titration in situ. The nitrate (NO3−) concentrations were analyzed by continuous flow injection analyzer (AMS Alliance Integral Futura, Frepillon, France). The cations (Na+, K+, Ca2+, Mg2+) and anions (Cl−, SO42−) were analyzed by ion chromatography (ThermoFisher ICS-900) with limit of detection (L.O.D) of 0.01 mg/L. The ionic charge balance of the water samples was less than 5%. The dissolved SiO2 was measured by molybdenum yellow method and was analyzed by ultraviolet spectrophotometer (Shimadzu UV-2600). Stable carbon isotope ratios of DIC (δ13CDIC) were measured according to the method described by (Assayag et al. 2006). δ13CDIC analyses were performed on an elemental analysis-isotope ratios mass spectrometer (IRMS, Delta V Advantage) coupled with a GasBench II. All isotope analyses were conducted within 1 week after sampling. The analytical precision for δ13CDIC analysis was ± 0.06‰. All laboratory analysis were made in the laboratory of School of Environmental Science and Engineering, Sun Yat-Sen University.
Data analysis
All statistical tests were conducted using SPSS version 22.0. Two-way ANOVA was used to test the seasonal and spatial differences in major ion concentrations and dissolved inorganic carbonate isotopes with significance at p < 0.05. DIC is defined as the sum of [CO2] + [HCO3−] + [CO32−] in water samples, and the compositions of DIC were calculated by PHREEQC Interactive 3.1.4-8929. Principal component analysis (PCA) was employed to unravel the underlying data set through the reduced new variables and analyzed the significant factors affecting the characteristics of water chemistry. The map of sampling sites was generated by ArcGIS 10.2.
Results
Hydrochemical signatures of river water
The major physical-chemical parameters and the carbon isotope values of DIC (δ13C-DIC) in the river water samples are presented in Table 1. The surface water temperature was significantly higher in the wet season (mean ± SD: 29.95 ± 0.95 °C) than in the dry season (20.36 ± 1.53 °C). The average of pH was 7.11 ± 0.48 in the wet season and 7.79 ± 0.42 in the dry season. The EC was higher in the dry season than in the wet season, ranging from 110 to 14,200 μs cm−1. The EC increased from upstream to the lower estuary, with some slight fluctuations in the middle stream. The TDS of the river water samples varied from 32.66 to 1147.07 mg L−1 in the wet season and from 56.82 to 6146.28 mg L−1 in the dry season. The DIC which is defined as the sum of [CO2] + [HCO3−] + [CO32−] can be calculated by using the alkalinity, water temperature, and pH. Based on calculation by PHREEQC, [CO2] and [CO32−] only account for less than 5% in most sampling sites; thus, the concentrations of HCO3− was used to represent the DIC in this study.
The major ion compositions of the river water samples in the PRD region are shown with Piper plot (Fig. 2). The river water samples were divided into three groups (I, II, and III) based on the major ion compositions (Fig. 2). Group I included most of the samples from the BJ River and the XJ River. In group I, Ca2+ was the dominant cation, accounting for approximately 69% of the total cations, followed by Mg2+ (15%), Na+ (13%), and K+ (3%); HCO3− was the dominant anion, which comprised 75% of the total anions, followed by SO42− (13%), Cl− (9%), and NO3− (3%), which suggested that the river water chemistry was controlled by chemical weathering rather than human activities. In the group II, river water samples mostly came from the GZ Channel and the DJ River, Ca2+ (49%) was also the predominant cation; however, the proportion of Na+ was higher than that in group I, with an average of 34% of the total cations; HCO3− (49%) was also the predominant anion; however, the proportions of Cl−, SO42−, and NO3− were higher than those in group I, with averages of 23%, 21%, and 6% of the total anions, respectively, which indicated that the river water chemistry was affected by anthropogenic inputs. In group III, the samples were from the estuary region, which was characterized by high proportions of Na+ (64%) and Cl− (80%), indicating a mixing effect of river water and seawater.
δ13C signatures of dissolved inorganic carbon
The δ13C values of dissolved inorganic carbon in the rivers of the PRD region are given in Table 1. The δ13C-DIC of river water samples exhibited a wide range, from − 12.95 to – 5.25‰, with an average of − 9.15 ± 1.60‰. There were significant seasonal variations in the δ13C-DIC compositions of river waters (p < 0.01, by ANOVA), with lower values in the wet season (− 10.23 ± 1.10‰) and higher values in the dry season (− 8.02 ± 1.18‰). In addition, the spatial variations in the δ13C-DIC were also significant (p < 0.01). During the wet season, the δ13C-DIC values in the DJ River were the highest (− 9.20 ± 1.03‰), followed by the BJ River (− 9.98 ± 0.97‰) and XJ River (− 10.59 ± 0.28‰), and the δ13C-DIC in the GZ Channel showed the lowest values with an average of − 11.18 ± 0.94‰. In contrast, during the dry season, the δ13C-DIC values in the GZ Channel were the highest with an average of − 6.88 ± 0.93‰, followed by those in the DJ River (− 7.64 ± 1.20‰), BJ River (− 8.27 ± 0.47‰), and XJ River (− 9.13 ± 0.66‰). The δ13C-DIC values measured in this study were similar to those from the Wuzhou and Makou stations in the Xijiang River (− 11.19‰ ± 1.18‰) (Gao and Wang 2015), which are located in the upper stream of our study region. However, the δ13C-DIC values in this study were significantly heavier than the δ13C-DIC values measured in the southeast coastal river basin (− 24.3 to − 11‰) (Liu et al. 2018a) and Ottawa River and its tributaries (− 17.4 to − 7.3‰) (Telmer and Veizer 1999).
Discussion
Mechanisms controlling the major ion chemistry
Principal component analysis (PCA) was used to study the factors controlling the chemical compositions, which is a multivariate technique that analyzes a data table representing the observations described by several intercorrelated quantitative dependent variables (Abdi and Williams 2010). The total dataset for the parameters of river water in the PRD was analyzed by PCA, and varimax rotation was used to reduce the number of variables to four principal components (PCs), which together explained 88.38% of the total variance in the data (Table 2). The first PC (PC1) explained approximately 38.18% of the total variations and had strong positive loadings on EC, Na+, K+, Mg2+, and Cl−, which was considered to represent the “salinity factor.” This factor indicated that salinity enhancement was caused by mineral dissolution and that salinity contributed to most of the TDS for water samples in the PRD region (Cao et al. 2016b; Yidana et al. 2008). The second PC (PC2) explained 20.03% of the total variance and presented high loadings for NO3− and SO42− concentrations. This result was recognized as an “anthropogenic pollution” factor, which was identified in the areas with intensive urbanization development, industrialization, or agriculture due to domestic sewage discharge and agricultural activities (Cao et al. 2016a; Cuoco et al. 2017; Wen et al. 2018). As the PRD region is characterized by a high degree of urbanization (Liu et al. 2018b), the high concentrations of NO3− and SO42− come from domestic sewage and atmospheric deposition. The third component (PC3) explained 17.66% of the total variance and was considered a “rock weathering factor” because of the high contributions of Ca2+, HCO3−, and SiO2. The fourth component (PC4) explained 12.49% of the total variance and had strong positive loadings on pH and δ13C-DIC, and the negative pole had a strong loading on temperature. This factor was defined as the “DIC source factor,” which reflected that the sources and transformations of DIC influenced the characteristics of hydrochemistry. In addition, the relationships between δ13C-DIC and pH or temperature primarily reflected a strong dependence of δ13C-DIC on pH and temperature values. First, the distribution of DIC species is set by pH; additions and withdrawals of CO2 result in the decrease and increase in pH of river water, respectively, due to changes in the carbonate system equilibria; for example, dissolution of carbonates generally increases pH and δ13C-DIC (Clark and Fritz 2013). Second, the changes in the δ13C-DIC were also caused by photosynthetic assimilation, which was affected by temperature. Additions of the respired CO2 decreased δ13C-DIC, while withdrawals of CO2 via photosynthetic assimilation increased δ13C-DIC (Wachniew 2006). Overall, in the PRD region, salinity, anthropogenic pollution, and rock weathering were treated as major factors controlling hydrochemistry, DIC was the major product of chemical weathering, and δ13C-DIC could reflect biogeochemical processes and record the influences of those major factors on hydrochemistry. The mechanisms are discussed below.
Natural and anthropogenic factors
The factor scores were employed to quantify the differences in hydrochemistry on river water among different rivers and between different seasons in the PRD region (Fig. 3). As discussed above, factor 2 and factor 3 are represented as “anthropogenic factor” and “rock weathering factor or natural factor,” respectively. These two factors were treated as two major reasons resulting in spatial variation in hydrochemistry because the samples from different rivers were well separated by the scores of factor 2 and factor 3 (Fig. 3a). In Fig. 3a, most of samples from the BJ River and XJ River were located in the left quadrant with factor 2 scores ≤ 0, and samples from the GZ Channel were located in the right quadrant with factor 2 scores ≥ 0. This result implied that the influence of anthropogenic activities was higher in the GZ Channel and was lower in the BJ River and XJ River. Specifically, in the GZ Channel during the dry season, samples were “extremely affected” by anthropogenic activities with factor 2 scores higher than + 1 (Fig. 3a). This finding suggested that the influence of anthropogenic factors was more effective in the dry season than in the wet season, which was related to the dilution of major ion concentrations by increased discharge in the wet season (Zeng et al. 2016). Figure 3a also shows that all of the samples from the XJ River were located in the upper quadrant with factor 3 scores ≥ 0 and most of the samples from the DJ River were located in the lower quadrant with factor 3 scores ≤ 0, while samples from the GZ Channel and BJ River were not well separated by factor 3. These results indicated that (1) rock weathering had a great influence on river water in the XJ River and less influence on the river water in the DJ River and (2) the hydrochemistry in the GZ Channel and BJ River was controlled by mixing processes including rock weathering, anthropogenic activities, and others, such as biological sources (Cuoco et al. 2017; Ding et al. 2017).
Seasonal and spatial patterns of the δ13C-DIC signature
As disused above, factor 1 was recognized as the “salinity factor” and factor 4 was treated as the “DIC source factor”. The PRD region is characterized by a large deltaic and coastal system, and thus, samples characterized by high Na+ and Cl− concentrations were from the estuary region. Figure 3b shows that some of the samples were located in the right quadrant with factor 1 scores ≥ 0, which suggested that the hydrochemistry of these samples was mainly affected by salinity and that these samples came from the estuary region. In addition, the samples from the wet season and the dry season were well separated by factor 4, showing factor 4 scores ≥ 0 for the dry season and factor 4 scores ≤ 0 for the wet season. This result suggested that DIC sources or relative biogeochemical processes of DIC exhibited significant seasonal variations in the PRD region because the δ13C-DIC values were determined by contributions of different DIC sources with distinct isotopic compositions and fractionation effects during biogeochemical processes (Gao and Wang 2015; Li et al. 2019; Zavadlav et al. 2013). More detailed sources and transformations of DIC are discussed in “Anthropogenic impacts on DIC.”
Anthropogenic impacts on DIC
As discussed above, anthropogenic factors were one of the most important factors affecting the hydrochemistry of river water, and the effect of human activities on DIC exhibited significant spatial variations in the PRD region. Generally, under natural conditions, the DIC in river water is dominantly derived from carbonate and silicate dissolution by carbonic acid (Eqs. (1)~(3)); however, increases in human activities affect the DIC flux; for example, sulfuric and nitric acids contribute to carbonate dissolution (Eqs. (4) and (5)) and increase the DIC flux (Ali and Atekwana 2011; Barnes and Raymond 2009; Gandois et al. 2011). Carbonate dissolution and silicate dissolution under natural conditions and anthropogenic impact can be expressed as follows:
-
(a)
Natural conditions:
Weathering of carbonate minerals by H2CO3:
Weathering of silicate minerals by H2CO3:
-
(b)
Anthropogenic impact:
Weathering of carbonate minerals by H2SO4 and HNO3:
Weathering of silicate minerals by H2SO4 and HNO3:
-
(c)
Nature condition and anthropogenic impacts:
Weathering of carbonate minerals by H2CO3, H2SO4, and HNO3:
Weathering of silicate minerals by H2CO3, H2SO4, and HNO3:
Divalent-cation silicates:
Contribution of human activities to DIC
In this study, the molar ratios between HCO3− and (Ca2+ + Mg2+) of river water averaged 2.17 in the wet season (Fig. 4a), which was close to the expected molar ratios of HCO3− and (Ca2+ + Mg2+) in carbonate dissolution by carbonic acid, according to Eq. (1). This result indicated that weathering of carbonate minerals by carbonic acids might be the dominant source of DIC, and rock weathering by other acids caused by human activities was not obvious in the wet season. The average molar ratio between HCO3− and (Ca2+ + Mg2+) in river water during the dry season was 1.60, and those from the GZ Channel and DJ River were 1.50 and 1.40, respectively (Fig. 4a). According to Eqs. (4) and (5), the molar ratio between HCO3− and (Ca2+ + Mg2+) is expected to be 1 when carbonate dissolution is controlled by sulfuric or nitric acid (Fig. 4a). The lower molar ratio between HCO3− and (Ca2+ + Mg2+) in the dry season suggested that rock weathering by carbonic acids could not be a unique weathering agent and sulfuric or nitric acid caused by human activities played an important role in rock weathering during this period, especially in the GZ Channel and DJ River, which was characterized by a high degree of urbanization (Du et al. 2015).
In this case, the carbonate and silicate weathering by carbonic, sulfuric, and nitric acids were considered in this study. If the contributions of carbonic, sulfuric, and nitric acids to carbonate or silicate dissolution are in equimolar amounts, carbonate and silicate dissolution can be expressed as Eqs. (10), (11), and (12), and the molar ratio between (HCO3− + SO42− + NO3−) and (Ca2+ + Mg2+) should be 7/4 (1.75) in carbonate dissolution and 9/4 (2.25) in the silicate dissolution (Fig. 4b). In the PRD region, the molar ratio between (HCO3− + SO42− + NO3−) and (Ca2+ + Mg2+) in the river water varied from 1.24 to 5.40 in the wet season and from 1.44 to 2.81 in the dry season, indicating that mixed rock weathering including carbonate dissolution and silicate dissolution in the river water was controlled by carbonic, sulfuric, and nitric acids (Jiang 2013).
We assumed that f1, f2, and f3 were the proportions of silicate dissolution contributed by carbonic acid, carbonate dissolution contributed by carbonic acid, and carbonate dissolution contributed by sulfuric and nitric acids to DIC, respectively. According to Eqs. (1)~(12), the contributions of silicate dissolution by carbonic acid (f1) and carbonate dissolution by carbonic acid (f2) could represent natural processes contributing to DIC, and the contributions of human activities to the DIC in river water were only through carbonate dissolution by sulfuric or nitric acids (f3). The results of f1, f2, and f3 can be calculated by the following steps.
First, the amounts of HCO3− derived from silicate dissolution and carbonate dissolution were calculated. In this study, precipitation inputs and evaporate weathering were not carried out due to their minor influences on water chemistry in the river water in the PRD region (Zhang et al. 2007). The Ca2+/Na+ ratio of 0.4 and Mg2+/Na+ ratio of 0.2 for the silicate end-member in the Pearl River (Zhang et al. 2007) were used to calculate the contributions of Ca2+ and Mg2+ from silicate weathering, and then residual Ca2+ and Mg2+ were attributed to carbonate weathering. Thus, the concentrations of HCO3− derived from silicate dissolution and carbonate dissolution can be calculated by the following equations:
Second, the amounts of HCO3− derived from carbonate dissolution by carbonic acid were discriminated. According to Eqs. (1), (4), and (5), the results can be calculated by the following equation:
Third, the amounts of HCO3− derived from carbonate dissolution by sulfuric and nitric acids were discriminated as followed:
Finally, the results of f1, f2 and f3 can be calculated as follows:
The calculated results are presented in Fig. 5, and the proportions of different weathering processes contributing to the DIC showed significant spatial variations in the PRD region. The contributions of carbonate dissolution by carbonic acid to the total concentrations of DIC in river water were high in the BJ River and XJ River, averaging 67% and 78%, respectively. This result was related to the larger area ratios of carbonate rock outcrops in the BJ River (Xuan et al. 2018) and XJ River (Gao et al. 2009). The contributions of silicate dissolution by carbonic acid to the total concentrations of DIC in river water were high in the GZ Channel and DJ River, averaging 37% and 50%, respectively, which was associated with a larger proportion of silicate outcrops (Xie chenji et al. 2013). In addition, carbonate dissolution by sulfuric and nitric acids, which represented human activities contributing to DIC, showed high proportions in the GZ Channel and DJ River, with averages of 42% and 34%, respectively. As shown in Fig. 5, the GZ Channel and the DJ River flow through the cities of Guangzhou and Dongguan, which are the metropolitan areas with high percentages of urbanized land, resulting in strong influences of human activities on water chemistry. Therefore, lithology (i.e., the nature of the rock) was the most important factor controlling the compositions of water chemistry; however, rock weathering was not controlled by only natural processes, and the influences of human activities on rock dissolution processes cannot be ignored, especially in highly urbanized areas.
Spatial distribution of contributed proportions of silicate dissolution by carbonic acid, carbonate dissolution by carbonic acid, and carbonate dissolution by sulfuric and nitric acids to DIC in the river water during the wet season (a) and the dry season (b), together with the extent of urban land in the Pearl River Delta. Data of urban land was referenced from (Liu et al. 2018b)
δ13C-DIC fingerprint of anthropogenic impacts on DIC
Mixing effect of different DIC sources
The δ13C-DIC composition of river water can reflect the contributions of DIC from different sources (Brunet et al. 2005; Spence and Telmer 2005). Here, the δ13C-DIC signatures were used to validate the mass balance results. In this study, the contribution of atmospheric CO2 to the DIC was minor due to the high partial pressure of CO2 in river water (Gao and Wang 2015). Thus, the DIC mainly comes from two primary sources, soil CO2 and carbonate bedrock. Soil CO2 is a product of the decomposition of organic matter during periods of high biological activity and root respiration (Calmels et al. 2014; Rasse et al. 2001). Depending on the pH, the equilibrium dissolution of soil CO2 into groundwater or river water resulted in the average δ13C-DIC of − 17‰ (Fig. 6) (Telmer and Veizer 1999). Most carbonate rocks, which originate in the oceans, result in marine δ13C, which has remained close to 0‰ since the end of the Proterozoic (Veizer et al. 1999). However, the δ13C of marine carbonate rocks of Paleozoic to Tertiary age have an average of 0.5‰ (Faure 1977). In addition, the δ13C of DIC could be affected by carbonate mineral precipitation (fractionation factor is approximately 1‰) (Zhang et al. 1995). Thus, to remain conservative, the DIC from carbonate dissolution had a δ13C of 0.5‰ in this study (Fig. 6). Using the δ13C-DIC values of primary sources and the stoichiometry of dissolution (Eqs. (1)~(12)), the δ13C-DIC values of end-members for this study were defined as follows: (1) silicate dissolution by carbonic acid involved only carbon from soil CO2, producing the end-member of δ13C-DIC = − 17‰; (2) dissolution of 1 mole of calcium or magnesium carbonate (δ13C = 0.5‰) by carbonic acid involved 1 mole of dissolved soil CO2 (δ13C = − 17‰), producing an end-member of δ13C-DIC = − 8.25‰; (3) dissolution of carbonate by sulfuric and nitric acids involved only carbon in calcium or magnesium carbonate, resulting in a δ13C-DIC = + 0.5‰ (Fig. 6).
Carbon isotopic signatures of various components of the carbon cycle in the river water system. White rectangles indicated the δ13C-DIC of end-members used in this study. Modified from (Schulte et al. 2011)
The relationships between the δ13C-DIC and major element ratios are presented in Fig. 7. As discussed in “Contribution of human activities to DIC,” the proportion of carbonate dissolution by sulfuric and nitric acids contributed to the DIC was high in the GZ Channel and DJ River; however, the DIC concentration was not higher than that in the BJ River and XJ River (Fig. 7a). This result suggested that although the contribution of carbonate dissolution by sulfuric and nitric acids to DIC increased, the export of DIC would not increase due to increases in sulfur and nitric acids by human activities. Thus, human activities changed only the proportion of DIC sources, and the increase in the proportion of DIC from carbonate dissolution by sulfuric and nitric acids would ultimately become a source of CO2 to the atmosphere on the geological timescale and affect the CO2 budget (Li et al. 2008). Figure 7b illustrates that most of the samples were located in the area defined by the three end-members, indicating that carbonate dissolution and silicate dissolution by carbonic, sulfuric, and nitric acids involved chemical weathering and had effects on ionic composition and carbon isotopes in river waters. To verify whether only these three end-members were the major contributors affecting the DIC in the river water, the theoretical δ13C-DIC value was calculated based on the results of the contributions of different end-members in “Contribution of human activities to DIC” and the δ13C-DIC signatures of each end-member. The calculated equation as follows:
The calculated results of the theoretical δ13C-DIC values of river water are shown in Fig. 8. The differences between the measured and theoretical δ13C-DIC values of most samples were within ± 2‰, indicating that the δ13C-DIC values of river waters were mainly controlled by the three isotopic end-members. Thus, the carbon isotopic signatures of DIC could be a useful tool to identify the sources and the δ13C-DIC fingerprint could reflect the mixing effect of weathering processes in the river ecosystem (Ali and Atekwana 2011; Jiang 2013). However, the differences between the measured and theoretical δ13C-DIC values of some samples were large, suggesting that there might be other processes affecting the δ13C-DIC value of river waters that are not accounted for by the isotopic end-members (Spence and Telmer 2005).
Biochemical alternation of the DIC pool
River water chemistry is largely a production of chemical rock weathering, dissolution/hydrolysis, and mineral precipitation (Schulte et al. 2011). As discussed above, the three weathering processes were primarily responsible for the compositions of the δ13C-DIC. Biochemical processes, such as outgassing of CO2 from the water-air interface (Spence and Telmer 2005; Varekamp et al. 2016), photosynthesis by phytoplankton (Liu et al. 2010; Wachniew 2006), and decomposition of organic matter by micro- and macro-organisms (de Medeiros Engelmann et al. 2018; Pozzato et al. 2018), are other controls of the enrichment or depletion of δ13C-DIC values. In this study, (1) during the wet season, the measured δ13C-DIC values were generally lower than the theoretical values (right of the 1:1 line in Fig. 8). This depleted δ13C-DIC might be caused by increased respiration of organic matter due to high temperatures in the wet season (Barnes and Raymond 2009). (2) During the dry season, the measured δ13C-DIC values were significantly higher than the theoretical values in the DJ River (left of the 1:1 line in Fig. 8), which might be associated with photosynthesis in the river water. Phytoplankton preferentially incorporate light 12C over heavy 13C during DIC uptake, leaving heavier δ13C in the residual DIC (Hama et al. 1983; Rau et al. 2001). In addition, the increase in δ13C-DIC during the dry season reflected the anthropogenic impact on the DIC pool. During the dry season, the nitrogen source in the river was mainly derived from a point source (e.g. sewage), coupled with an increase in nutrient concentration due to decreased precipitation and reduced runoff and flow velocity, which was beneficial to the growth of phytoplankton and subsequently increased the δ13C-DIC signature. Moreover, in the dry season, the measured δ13C-DIC values in the GZ Channel and DJ River were significantly higher than those in the BJ River and XJ River (p < 0.01), which further indicated that increased sewage discharge due to high degree of urbanization caused an enrichment of δ13C-DIC (Barnes and Raymond 2009).
Overall, the evidence from both the chemical data and δ13C-DIC of river water indicated that chemical compositions in the river water were controlled by both natural and anthropogenic factors in the PRD region, and carbonate dissolution by sulfuric and nitric acids introduced by human activities should be responsible for the increased δ13C-DIC of river water. In addition, the δ13C-DIC of river water was also affected by biogeochemical processes (e.g. photosynthesis and nitrification) due to isotope fractionation, and high δ13C-DIC values in the highly urbanized area reflected anthropogenic impacts on the δ13C-DIC signatures. Therefore, human activities promoted the use of DIC by phytoplankton, thereby fixing carbon and decreasing the DIC flux, and the DIC contributed from carbonate dissolution by sulfuric and nitric acids caused by human activities would become a source of atmospheric CO2 on the geological timescale.
Conclusions
The hydrochemical compositions and isotopic signatures of the dissolved inorganic carbon in the PRD region revealed that carbonate dissolution, silicate dissolution, and anthropogenic inputs provided the majority of the solutes in the river water. For the river water samples in the BJ River and XJ River, Ca2+ was the most abundant cation, accounting for 69% of the total cations, and HCO3− was the dominant anion, accounting for 75% of the total anions, which suggested that the chemical compositions were mainly contributed by carbonate dissolution. For the river water samples in the GZ Channel and DJ River, the proportions of Na+, Cl−, SO42−, and NO3− significantly increased, indicating that the increase in anthropogenic inputs contributed to the solutes in the river water. The results of principal component analysis showed that anthropogenic pollution and rock weathering were recognized as the main factors controlling the regional hydrochemistry. These two factor scores indicated the spatial variation in hydrochemistry. The water samples in the GZ Channel were characterized by high relative anthropogenic pollution factor; however, the influence of the rock weathering factor was greater in the XJ River and BJ River. In addition, significant seasonal variation in the DIC sources or relative biogeochemical processes of DIC were found by the evidence of δ13C-DIC signatures.
As the major product of chemical weathering, DIC concentrations and their carbon isotopes provide a better understanding of the processes controlling regional hydrochemistry. The proportions of the contribution from three theoretical weathering processes to the DIC showed that the contributions of carbonate dissolution by carbonic acid to the total concentrations of DIC in river water were high in the BJ River and XJ River, and the contributions of silicate dissolution by carbonic acid were high in the GZ Channel and DJ River, which were related to different rock outcrops. The contributions of carbonate dissolution by sulfuric and nitric acids to the DIC were high in the GZ Channel and DJ River, which was associated with a higher degree of urbanization, indicating that human activities played important roles in chemical compositions in the river water. However, carbonate dissolution by sulfuric and nitric acids caused by human activities did not result in an increase of in the DIC flux but changed the distribution of DIC sources, and this part of DIC would ultimately become a source of CO2 to the atmosphere on the geological timescale and affect the CO2 budget. The evidence of the δ13C-DIC signature also indicated that human activities promoted photosynthesis of phytoplankton which changed the DIC pool.
References
Abdi H, Williams LJ (2010) Principal component analysis. Wiley Interdiscip Rev Comput Stat 2:433–459
Ali HN, Atekwana EA (2011) The effect of sulfuric acid neutralization on carbonate and stable carbon isotope evolution of shallow groundwater. Chem Geol 284:217–228
Assayag N, Rivé K, Ader M, Jézéquel D, Agrinier P (2006) Improved method for isotopic and quantitative analysis of dissolved inorganic carbon in natural water samples. Rapid Commun Mass Spectrom 20:2243–2251
Barnes RT, Raymond PA (2009) The contribution of agricultural and urban activities to inorganic carbon fluxes within temperate watersheds. Chem Geol 266:318–327
Berner RA, Kothavala Z (2001) GEOCARB III: a revised model of atmospheric CO2 over Phanerozoic time. Am J Sci 301:182–204
Brunet F et al (2005) δ13C tracing of dissolved inorganic carbon sources in Patagonian rivers (Argentina). Hydrol Process 19:3321–3344
Calmels D, Gaillardet J, François L (2014) Sensitivity of carbonate weathering to soil CO2 production by biological activity along a temperate climate transect. Chem Geol 390:74–86
Cao Y, Tang C, Cao G, Wang X (2016a) Hydrochemical zoning: natural and anthropogenic origins of the major elements in the surface water of Taizi River Basin, Northeast China. Environ Earth Sci 75:811
Cao Y, Tang C, Song X, Liu C, Zhang Y (2016b) Identifying the hydrochemical characteristics of rivers and groundwater by multivariate statistical analysis in the Sanjiang Plain, China. Appl Water Sci 6:169–178
Cartwright I (2010) The origins and behaviour of carbon in a major semi-arid river, the Murray River, Australia, as constrained by carbon isotopes and hydrochemistry. Appl Geochem 25:1734–1745
Clark ID, Fritz P (2013) Environmental isotopes in hydrogeology. CRC Press, Boca Raton
Cuoco E et al (2017) Geolithological and anthropogenic controls on the hydrochemistry of the Volturno river (Southern Italy). Hydrol Process 31
de Medeiros Engelmann P, Dos Santos VHJM, Barbieri CB, Augustin AH, Ketzer JMM, Rodrigues LF (2018) Environmental monitoring of a landfill area through the application of carbon stable isotopes, chemical parameters and multivariate analysis. Waste Manag 76:591–605
Ding H et al (2017) Geochemistry of the dissolved loads of the Liao River basin in northeast China under anthropogenic pressure: chemical weathering and controlling factors. J Asian Earth Sci 138:657–671
Du S, Van Rompaey A, Shi P, Wang Ja (2015) A dual effect of urban expansion on flood risk in the Pearl River Delta (China) revealed by land-use scenarios and direct runoff simulation. Nat Hazards 77:111–128
Dubois KD, Lee D, Veizer J (2010) Isotopic constraints on alkalinity, dissolved organic carbon, and atmospheric carbon dioxide fluxes in the Mississippi River. J Geophys Res Biogeosci:115
Faure G (1977) Principles of Isotope Geology. Wiley, New York 589
Gandois L, Perrin A-S, Probst A (2011) Impact of nitrogenous fertiliser-induced proton release on cultivated soils with contrasting carbonate contents: a column experiment. Geochim Cosmochim Acta 75:1185–1198
Gao Q, Wang Z (2015) Dissolved inorganic carbon in the Xijiang River: concentration and stable isotopic composition. Environ Earth Sci 73:253–266
Gao Q et al (2009) Chemical weathering and CO2 consumption in the Xijiang River basin, South China. Geomorphology 106:324–332
Guo J, Wang F, Vogt RD, Zhang Y, Liu CQ (2015) Anthropogenically enhanced chemical weathering and carbon evasion in the Yangtze Basin. Sci Rep 5:11941
Halas S, Szaran J, Niezgoda H (1997) Experimental determination of carbon isotope equilibrium fractionation between dissolved carbonate and carbon dioxide. Geochim Cosmochim Acta 61:2691–2695
Hama T et al (1983) Measurement of photosynthetic production of a marine phytoplankton population using a stable 13C isotope. Mar Biol 73(1):31–36
Hartmann J, Moosdorf N, Lauerwald R, Hinderer M, West AJ (2014) Global chemical weathering and associated P-release—the role of lithology, temperature and soil properties. Chem Geol 363:145–163
Hu J, Li S (2009) Modeling the mass fluxes and transformations of nutrients in the Pearl River Delta, China. J Mar Syst 78:146–167
Jiang Y (2013) The contribution of human activities to dissolved inorganic carbon fluxes in a karst underground river system: evidence from major elements and δ13CDIC in Nandong, Southwest China. J Contam Hydrol 152:1–11
Lerman A, Wu L (2006) CO2 and sulfuric acid controls of weathering and river water composition. J Geochem Explor 88:427–430
Levin I, Graul R, Trivett NBA (1995) Long-term observations of atmospheric CO2 and carbon isotopes at continental sites in Germany. Tellus B 47:23–34
Li S-L, Calmels D, Han G, Gaillardet J, Liu C-Q (2008) Sulfuric acid as an agent of carbonate weathering constrained by δ13CDIC: examples from Southwest China. Earth Planet Sci Lett 270:189–199
Li X et al (2019) Hydrochemistry and dissolved inorganic carbon (DIC) cycling in a tropical agricultural river, Mun River Basin, Northeast Thailand. Int J Environ Res Public Health 16:3410
Liu Z, Dreybrodt W, Wang H (2010) A new direction in effective accounting for the atmospheric CO2 budget: considering the combined action of carbonate dissolution, the global water cycle and photosynthetic uptake of DIC by aquatic organisms. Earth Sci Rev 99:162–172
Liu W et al (2018a) Geochemistry of the dissolved loads during high-flow season of rivers in the southeastern coastal region of China: anthropogenic impact on chemical weathering and carbon sequestration. Biogeosciences 15:1726–4170
Liu X et al (2018b) High-resolution multi-temporal mapping of global urban land using Landsat images based on the Google Earth Engine Platform. Remote Sens Environ 209:227–239
Lu F-H, Ni H-G, Liu F, Zeng EY (2009) Occurrence of nutrients in riverine runoff of the Pearl River Delta, South China. J Hydrol 376:107–115
Michel M (2003) Global analysis of river systems: from Earth system controls to Anthropocene syndromes. Philos Trans Biol Sci 358:1935–1955
Murphy BP, Johnson JPL, Gasparini NM, Sklar LS (2016) Chemical weathering as a mechanism for the climatic control of bedrock river incision. Nature 532:223–227
Niinikoski PIA, Karhu JA (2017) Natural vs. anthropogenic effects in the composition of dissolved inorganic carbon in a boreal river with a seasonal base flow component. Hydrol Res 48:1585–1593
Ou S et al (2019) The influence of runoff and wind on the dispersion patterns of suspended sediment in the Zhujiang (Pearl) River Estuary based on MODIS data. Acta Oceanol Sin 38:26–35
Peter AR, James EB (2000) Bacterial consumption of DOC during transport through a temperate estuary. Aquat Microb Ecol 22:1–12
Pozzato L et al (2018) Origin of remineralized organic matter in sediments from the Rhone River prodelta (NW Mediterranean) traced by Δ14C and δ13C signatures of pore water DIC. Prog Oceanogr 163:0079–6611
Rasse DP et al (2001) Modelling short-term CO2 fluxes and long-term tree growth in temperate forests with ASPECTS. Ecol Model 141:35–52
Rau GH, Chavez FP, Friederich GE (2001) Plankton 13C/12C variations in Monterey Bay, California: evidence of non-diffusive inorganic carbon uptake by phytoplankton in an upwelling environment. Deep-Sea Res I Oceanogr Res Pap 48:79–94
Raymond PA, Oh N-H, Turner RE, Broussard W (2008) Anthropogenically enhanced fluxes of water and carbon from the Mississippi River. Nature 451:449
Samanta S, Dalai TK, Pattanaik JK, Rai SK, Mazumdar A (2015) Dissolved inorganic carbon (DIC) and its δ13C in the Ganga (Hooghly) River estuary, India: evidence of DIC generation via organic carbon degradation and carbonate dissolution. Geochim Cosmochim Acta 165:226–248
Schulte P et al (2011) Applications of stable water and carbon isotopes in watershed research: weathering, carbon cycling, and water balances. Earth Sci Rev 109:20–31
Spence J, Telmer K (2005) The role of sulfur in chemical weathering and atmospheric CO2 fluxes: evidence from major ions, δ13CDIC, and δ34SSO4 in rivers of the Canadian Cordillera. Geochim Cosmochim Acta 69:5441–5458
Strokal M et al (2015) Increasing dissolved nitrogen and phosphorus export by the Pearl River (Zhujiang): a modeling approach at the sub-basin scale to assess effective nutrient management. Biogeochemistry 125:221–242
Telmer K, Veizer J (1999) Carbon fluxes, pCO2 and substrate weathering in a large northern river basin, Canada: carbon isotope perspectives. Chem Geol 159:61–86
Varekamp JC, Caldwell S, Capece L, Horne J, Upin H (2016) The Newberry crater lakes, oregon. Keck Geology Consortium, 29th Annual Symposium Volume
Veizer J et al (1999) 87Sr/86Sr, δ13C and δ18O evolution of Phanerozoic seawater. Chem Geol 161:59–88
Vogel JC (1993) Variability of carbon isotope fractionation during photosynthesis. In: Stable Isotopes and Plant Carbon-Water Relations. Academic, San Diego, pp 29–38
Wachniew P (2006) Isotopic composition of dissolved inorganic carbon in a large polluted river: the Vistula, Poland. Chem Geol 233:293–308
Wen J, Tang C, Cao Y, Li X, Chen Q (2018) Hydrochemical evolution of groundwater in a riparian zone affected by acid mine drainage (AMD), South China: the role of river–groundwater interactions and groundwater residence time. Environ Earth Sci 77:794
Xia D et al (2017) Isotope geochemistry, hydrochemistry, and mineralogy of a river affected by acid mine drainage in a mining area, South China. RSC Adv 7:43310–43318
Xie GQ, Zhen T, Longhai L, Lishanchi (2013) Chemical weathering and CO2 consumption in the Dongjiang River Basin. Acta Sci Circumst 33:2123–2133
Xu Q et al (2019) Temporal and spatial differences in carbon emissions in the Pearl River Delta based on multi-resolution emission inventory modeling. J Clean Prod 214:615–622
Xuan Y et al (2018) Spatial and temporal variation of hydro-chemistry and chemical weathering characteristics in the Beijiang River Basin. [in Chinese with English abstract]. Res Environ Sci 31:1078–1087
Yang M, Liu Z, Sun H, Yang R, Chen B (2016) Organic carbon source tracing and DIC fertilization effect in the Pearl River: insights from lipid biomarker and geochemical analysis. Appl Geochem 73:132–141
Ye F, Jia G, Xie L, Wei G, Xu J (2016) Isotope constraints on seasonal dynamics of dissolved and particulate N in the Pearl River Estuary, south China. J Geophys Res 121:8689–8705
Ye F, Guo W, Shi Z, Jia G, Wei G (2017) Seasonal dynamics of particulate organic matter and its response to flooding in the Pearl River Estuary, China, revealed by stable isotope (δ13C and δ15N) analyses. J Geophys Res Oceans 122:6835–6856
Yidana SM, Ophori D, Banoeng-Yakubo B (2008) A multivariate statistical analysis of surface water chemistry data—the Ankobra Basin, Ghana. J Environ Manag 86:80–87
Yu F et al (2010) Bulk organic δ13C and C/N as indicators for sediment sources in the Pearl River delta and estuary, southern China. Estuar Coast Shelf Sci 87:618–630
Yu H et al (2015) Seasonal variations in the nitrogen isotopic composition of dissolved nitrate in the Changjiang River estuary, China. Estuar Coast Shelf Sci 155:148–155
Zavadlav S, Kanduč T, McIntosh J, Lojen S (2013) Isotopic and chemical constraints on the biogeochemistry of dissolved inorganic carbon and chemical weathering in the karst watershed of Krka River (Slovenia). Aquat Geochem 19:209–230
Zeng C, Liu Z, Zhao M, Yang R (2016) Hydrologically-driven variations in the karst-related carbon sink fluxes: insights from high-resolution monitoring of three karst catchments in Southwest China. J Hydrol 533:74–90
Zhang J, Quay PD, Wilbur DO (1995) Carbon isotope fractionation during gas-water exchange and dissolution of CO2. Geochim Cosmochim Acta 59:107–114
Zhang SR et al (2007) Water chemistry of the Zhujiang (Pearl River): natural processes and anthropogenic influences. J Geophys Res J Geophys Res Atmos 112:137–161
Zhou H, Shi P, Wang Ja, Yu D, Gao L (2011) Rapid urbanization and implications for river ecological services restoration: case study in Shenzhen, China. J Urban Plann Dev 137:121–132
Funding
This research work was financially supported by the Fundamental Research Funds for the Central Universities (Grant No.19lgpy148), the General Program of the National Natural Science Foundation of China (Grant No.41877470), and the National Key R& D Program of China (Nos. 2017YFB0903700 and 2017YFB0903703).
Author information
Authors and Affiliations
Corresponding author
Additional information
Responsible Editor: Zhihong Xu
Publisher’s note
Springer Nature remains neutral with regard to jurisdictional claims in published maps and institutional affiliations.
Rights and permissions
About this article
Cite this article
Xuan, Y., Cao, Y., Tang, C. et al. Changes in dissolved inorganic carbon in river water due to urbanization revealed by hydrochemistry and carbon isotope in the Pearl River Delta, China. Environ Sci Pollut Res 27, 24542–24557 (2020). https://doi.org/10.1007/s11356-020-08454-4
Received:
Accepted:
Published:
Issue Date:
DOI: https://doi.org/10.1007/s11356-020-08454-4