Abstract
Soil balls containing the so-called effective microorganisms (EM) have been applied to improve water quality of small ponds, lakes, and streams worldwide. However, neither the physical conditions facilitating their proper application nor the diversity of microbial community in such soil balls have been investigated. In this study, the application of 0.75 % of hardener to the soil balls exerted almost neutral pH (pH 7.3) which caused up to a fourfold increased hardness of the soil ball. Moreover, the 0.75 % of hardener in the soil ball also improved the water quality due to a significant reduction in dissolved oxygen, total phosphorus, and total nitrogen contents. Metagenomic analysis of the microbial community in the soil ball with 0.75 % hardener was compared with control (traditional soil ball) through next-generation sequencing. The traditional soil ball microbial community comprised 96.1 % bacteria, 2.7 % eukaryota, and 1 % archaea, whereas the soil ball with 0.75 % hardener comprised 71.4 % bacteria, 27.9 % eukaryota, and 0.2 % viruses. Additionally, metagenomic profiles for both traditional and improved soil balls revealed that the various xenobiotic biodegradation, such as those for caprolactam, atrazine, xylene, toluene, styrene, bisphenol, and chlorocyclohexane might be responsible for organic waste cleanup.
Similar content being viewed by others
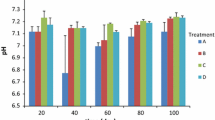
Explore related subjects
Discover the latest articles, news and stories from top researchers in related subjects.Avoid common mistakes on your manuscript.
Introduction
Microbial biotechnology is the most recent approach to wastewater treatment and is essential for protecting human health and the environment (Mielczarek et al. 2013). In order to guarantee the optimal operation using the effective microorganism technology, it is very important to understand the structure, function, and microbial community dynamics involved in these approaches (Zakaria et al. 2010). Soil balls have been used as carrier to immobilize effective microorganisms for the remediation of contaminated water environment (Ekpeghere et al. 2012). The concept of “effective microorganisms” (EM) was introduced by Dr. Teruo Higa, and since then, EMs became an important part of natural farming (Higa 1998). Those microbes are selected based on their functions as fixation of atmospheric nitrogen, decomposition of organic wastes and residues, suppression of soil-borne pathogens, recycling and increased availability of plant nutrients, solubilization of insoluble nutrient sources, and degradation of toxicants including pesticides (Higa and Parr 1994). EM is co-cultures of naturally occurring beneficial microorganisms, and it is widely applied as inoculants to soil, water and plants for the improvement of soil and water quality, as well as for plant growth and crop yield (Grover et al. 2011; Javaid 2010). Soil balls with EM can be a good alternative for stream water treatment in eco-friendly environments, but there have been no report on their physicochemical properties and microbial diversity, which can be the main factors in their efficacy. There are some skeptical views to the soil ball treatment. Generally, soil balls can be easily broken down to small particles in the stream water and therefore, its original role as an effective microorganism carrier is lost. Moreover, it is still unknown whether the microbial community of the soil ball is effective to water treatment or not.
Understanding microbial community composition in terms of different populations and its potential interactions in a particular environment is crucial for the efficient utilization of EM. Characterization of microbial community structure in wastewater and contaminated soil has been limited only to culturable microorganisms from the environmental samples. Currently, only a fraction of the microorganisms can be cultured through laboratory techniques (Nogales et al. 2001). While 90 to 99 % of microorganisms are not cultivable with conventional cultivation techniques although they may be viable in natural environments. One of the most significant innovations in microbial ecology research undoubtedly come in the 1990s with the application of culture-independent molecular biological tools to study the diversity and dynamics of microbial communities in fine detail (Gilbride et al. 2006).
The molecular techniques used to determine environmental microbial communities based on nucleic acid are DNA re-association kinetics (Torsvik et al. 1990), nucleic acid hybridization (Buckley et al. 1998), fluorescence in situ hybridization (Christensen et al. 1999; Ravenschlag et al. 2000), microarray (Rhee et al. 2004; Small et al. 2001), denaturing gradient gel electrophoresis (DGGE) (Muyzer et al. 1993), and quantitative real time polymerase chain reaction (qRT-PCR) (Heid et al. 1996). Following the pioneering work by Giovannoni et al. (1990), targeting the 16S ribosomal RNA (rRNA) gene,has been widely used to examine different wastewater treatment systems and is still often employed where detailed taxonomic information is required (Sanz and Köchling 2007). However, these laborious and time-consuming methods are not suitable for high-throughput analyses dealing with the large number of samples and have the drawback of detecting the whole community (Bustin 2002; Denman and McSweeney 2005; Fierer et al. 2005). Recently, the development of next-generation sequencing technology (NGS) has enabled the detection of a specific microorganism in a whole microbial community along with metabolic pathways that can be derived from NGS data of environmental metagenome.
In this study, an improved method for the EM soil ball making was developed. The formulation of the soil ball was altered to increase pH and physical hardness. Microbial community of soil ball was revealed by next-generation DNA sequencing technology using a semiconductor sequencer. The metabolic pathways responsible for xenobiotic degradation were also assessed from the metagenome data analysis and annotation.
Materials and methods
Soil balls preparation
Soil balls were prepared by combining 450 g loess, 25 mL of EM solution, 25 g EM bokashi mixture, to give a final weight of 500 g. The EM bokashi mixture was added to increase bioactivity and was prepared as follows: 500 g rice bran was combined with 500 μL molasses (DUKSAN Co., Seoul, Korea) and 2 mL EM solution, and then incubated at 30 °C for 2 weeks. After combining loess and EM bokashi, the mixture was molded into sphere balls and further incubated at 30 °C for 6 days for microbial growth.
Hardness and pH control of the soil balls
To increase the hardness of the soil balls, calcium oxide (CaO) and silicon dioxide (SiO2, Sigma-Aldrich Co., St. Louis, MO, USA) were used. Calcium oxide and silicon dioxide were mixed at 3:1 molar ratios and added at 0 to 1 % (w/w) to the soil ball. Mixture of calcium oxide and silicon dioxide changed the pH of soil ball dramatically to high alkaline. In order to maintain neutral pH, 25 mL of 1 M citrate buffer (pH 4.2) was also added to 500 g of soil ball-making ingredient. The hardness of the soil balls was measured by using a 5-kg fruit hardness tester (Takemura Co., Japan). Soil ball’s pH was measured as per NIAST’s (2000) “Soil and plant analysis method”: a soil ball aliquot of 5 g was dissolved in 25 mL sterile distilled water and incubated at 25 °C for 1 h at 200 rpm. The pH was then checked with an Orion 520A pH meter (Orion Research Inc., Boston, MA, USA).
Water quality analysis
To test water quality improvement ability of the soil ball, stream water was sampled from Dalseong Wetland, Daegu, Republic of Korea. Samples dissolved with oxygen (DO) was measured with a portable DO meter (Mettler Toledo, Columbus, OH, USA), total nitrogen (TN) was determined by Kjeldahl’s method (Apha 1998), and total phosphorus (TP) was determined using a spectrophotometer (Hitachi U-2001) by molybdate-ascorbic acid method. The DO, TN, and TP were measured before and after soil ball treatments. A soil ball effect demonstration apparatus was assembled with 100 L of total water volume containing 10 L/min water stream generator (Fig. 1).
Metagenomic DNA isolation from soil ball and wastewater
Metagenomic DNA from the soil samples and water samples were extracted using PowerSoil® DNA Isolation Kit and PowerWater® DNA Isolation Kit (Mo-Bio Co., Carlsbad, CA, USA), respectively, according to the manufacturer’s recommendations. After grinding the entire soil ball, 0.25 g of homogenized soil was taken. DNA quantity and purity for the sequencing was confirmed through a nanophotometer (Model 1443; Implan GmbH, Munich, Germany). The DNA was stored at −20 °C for further experimental use.
Metagenome sequencing using Ion Torrent PGM
For the next-generation sequencing library preparation, 1 μg of metagenomic DNA was sheared to 200-bp length by BioRuptor UCD-200 TS Sonication System (5 cycles of 15 min; Diagenode Co., Belgium and Denville, NJ, USA). The Ion Xpress Fragment Library Kit (Life Technologies, Carlsbad, CA, USA) was used for subsequent library preparation. Shearing and phosphate end repairing was followed by ligation of the respective barcode adapters to each sample. Library size selection was done by an E-Gel SizeSelect at 2 % agarose gel on the E-Gel iBase Power System (Life Technologies, Carlsbad, CA, USA). Further, the sample was nick translated and amplified by ten PCR cycles. Precise quality and quantity of the amplified DNA library was analyzed by an Agilent 2100 Bioanalyzer (Agilent Technology, Santa Clara, CA). The DNA library was diluted to 16 pM and emulsion PCR was performed using the Ion OneTouch System (Life Technologies, Gaithersburg, MD), followed by enrichment for template-positive ion sphere particles using Dynabeads MyOne Streptavidin C1 beads (Invitrogen, Carlsbad, CA). Each sequencing sample was loaded on an Ion 316D chip and sequenced on the Ion Torrent PGM for 520 flows with Ion PGM Sequencing 200 Kit (Life Technologies, Gaithersburg, MD). The Ion Torrent platform-specific pipeline software was used to generate sequence reads, trim adapter sequences, filter, and remove poor signal-profile reads from the raw data. Prepared DNA sequence was uploaded to the Metagenomics RAST (MG-RAST) server (http://metagenomics.anl.gov/) and subjected to bioinformatics analysis with MG-RAST pipeline.
Statistical analysis
For water quality and soil ball structure stability measurements, readings were done in three replicates. The results including mean and standard deviations (SD) were calculated using MS-Excel (version 2007; Microsoft Inc., Redmond, WA, USA). While the statistical significance at p < 0.05 was calculated through Duncan’s multiple range test (DMRT; SAS, version 9.5).
Results
Hardness and pH control of the soil ball
The hardness of the soil ball was increased up to sevenfold when 1.00 % (w/w) of hardener was applied. Soil ball with 0.75 % of hardener showed exact neutral pH (pH 7.32) and fourfold increased hardness (Table 1). Therefore, further demonstrative experiment of water quality improvement was performed with soil ball containing 0.75 % hardener. Under the controlled condition shown in Fig. 1 (10 L/min of water stream in a total of 100 L of volume), the unmodified soil ball dissociated after 48 h, whereas the soil ball with 0.75 % hardener maintained its exact shape up to 7 days. Furthermore, after soil ball dissociation, the unmodified soil ball was completely dispersed in suspension throughout the water, while the hardened soil ball dissociated over a small area and remained out of suspension (Fig. A1, Supplementary information). Basically, sand and zeolite are normally used as carrier for microbes instead of loess. But sand and zeolite could not make as a ball shape. They were just like powder formula and cannot be applied in stream water (Fig. A2, Supplementary information).
Quality analysis of soil ball-treated water
To assess soil ball effect on water, stream water samples from natural wetland were treated with traditional soil ball and soil ball with 0.75 % of hardener. The initial pH, DO, TP, and TN values were measured just after setting the water quality assessment apparatus (Table 2, day 0). Both soil ball treatments did not affect water quality at the first day of treatment except for a slight low pH value with traditional soil ball sample. After 30 days of treatment, traditional soil ball did not show the changes for all parameters but the soil ball with hardener showed significant reduction in DO, TP, and TN. This reduction may be attributed to oxygen, phosphorus, and nitrogen consumption by the activities of microorganisms.
Analysis of soil ball microbial community and metabolic pathways by whole metagenome sequencing
Next-generation sequencing analysis was performed to determine microbial community as well as metagenomic pathways of soil ball. A total of 2,464,533 DNA sequence reads with an average size of 213 bp (spanning 525.51 Mb of total sequences) were generated from the metagenome of the traditional soil ball, and a total of 2,823,708 sequence reads with an average size of 196 bp (spanning 552.61 Mb of total sequences) were generated from the improved soil ball with 0.75 % hardener. From the sequence reads, 10,207 (traditional soil ball) and 91,244 (improved soil ball) ribosomal RNA sequence reads were detected using the MG-RAST pipeline. The microbial community of the traditional soil ball comprised 96.1 % of Bacteria, 2.7 % of Eukaryota and 1 % of Archaea, whereas the improved soil ball comprised 71.4 % of Bacteria, 27.9 % of Eukaryota, and 0.2 % of viruses (Fig. 2). In the pilot test, improved soil ball with 0.5 % hardener did not show significant differences in microbial community change. So only improved soil ball with 0.75 % hardener detailed microbial community was compared with traditional soil ball microbial community (Fig. A3, Supplementary information). Notably, there was a remarkable increase of eukaryotic diversity in the improved soil ball sample. The heat map analysis based on metagenomic gene annotation showed a more detailed microbial community change in the phylum level (Fig. 3). Although absolute number of biodiversity was increased ninefold in the improved soil ball, relative bacterial portion was decreased including phyla Proteobacteria, Actinobacteria, Bacteroidetes, and Acidobacteria except for Firmicutes. While eukaryotic phyla Ascomycota, Glomeromycota, Apicomplexan, Cnidarian, Chytridiomycota, and other unclassified fungi were significantly increased (Fig. 3). The pH of traditional soil ball was 4.48 while the improved one’s pH was 7.54. The pH of improved soil ball was mainly increased by the calcium oxide (CaO) and silicon dioxide (SiO2) which were added as hardener. The neutral pH of the improved soil ball was caused to proliferate of Eukaryota which are major role in wastewater treatment.
Heat map of organism showing biodiversity. This data was calculated for the metagenomes of traditional soil ball (left) and improved soil ball (right) samples. The data were compared with M5RNA using a maximum e value of 1e−5, a minimum identity of 60 %, and a minimum alignment length of 15 measured in amino acid for protein and bp for RNA databases
Xenobiotic degradation pathway of soil ball metagenome
The complete metabolic pathways of the traditional and improved soil ball samples were constructed through the Kyoto Encyclopedia of Genes and Genomes (KEGG) server. Both samples showed lipid and carbohydrate metabolism, xenobiotics biodegradation, terpenoid, and polyketide metabolism pathway genes (Fig. 4). Although xenobiotics biodegradation-related genes were detected in both samples, the traditional soil ball showed a higher number of genes for xenobiotics degradation metabolic pathways than the improved one. More precisely, the lower pH enrichment was implicated in degradation of benzoate, aminobenzoate, caprolactam, atrazine, xylene, toluene, styrene, bisphenol, chlorocyclohexane, fluorobenzoate, and other related pathways.
Discussion
The recent development in molecular techniques that allow fine-scale, detailed description of microbial community structure has led to growing examinations of and interest in evaluating and quantifying the nature of soil property variation from the micro-scale to the continental (Fierer et al. 2005; Green et al. 2004; Ranjard and Richaume 2001; Ritz et al. 2004). Depending on soil physical and chemical composition, each soil environment has their own microbial community and could be different from each other (Bundy et al. 2002). In this current investigation, only one type of soil sample was evaluated. The purpose of the experiment was to check the effect of EM incorporation as well as soil ball physical composition on the soil microbial community. The study characterized microbial communities in soil balls of different pH values using metagenomic DNA sequencing, employing next-generation sequencing techniques.
Earlier approaches have investigated the effect of soil pH on fungal and bacterial growth in soil of forest humus or studied the acute effects of pH increase on fungal and bacterial growth (Rousk et al. 2009). In most cases, increased bacterial and decreased fungal growths were observed at higher pH. Our study showed that both bacterial and fungal diversity were increased with a change in pH from 4.48 to 7.54. One possible explanation could be independent physiological limitations by pH of different groups of organisms, as low hydrogen ion concentrations retard fungal growth and high hydrogen ion concentrations retard bacterial growth, with no direct causal relationship between the groups of organisms (Nicol et al. 2008). Genus Lactobacillus, one of the major members of EM, modify their environment by changing the pH to a value that is more optimal for their growth. It may be possible that the Lactobacillus spp. growing on traditional soil ball will decrease the soil ball pH, which has universal inhibition of all microbial variables. While pH of the improved soil ball was not lowering by the lactic acid bacteria since the hardener tend to increase alkalinity. This pH buffer effect may favor the growth of both eukaryotic and bacterial community.
Bacteria belonging to the phyla Proteobacteria and Bacteroidetes are generally observed in the wastewater environments, many of which are involved in contaminant degradation, suggesting that such communities are important for wastewater treatment (Hesham et al. 2011; Moura et al. 2009). In this study, comparison of bacterial communities between the traditional soil ball (pH 4.48) and the improved soil ball (pH 7.54) showed differences in community structure due to changes in pH. Overall, an increase in pH from acidic to neutral was correlated with an increase in growth of microbial communities. The DO reduction significantly in 0.75 % hardener at 30 days can be correlated with increased soil ball community. Similarly, the decrease in TN and TP can be correlated with increase in microbial community which actively consumes the TN and TP.
Treatment of wastewater using immobilized living cell systems is receiving increased attention, with different immobilization methods as well as a variety of carriers being applied. Certain reports have described ceramics as appropriate carriers for the adsorbed immobilized cells owing to their porosity and reusability (Kariminiaae-Hamedaani et al. 2003). The major problem of soil ball as immobilizing agent and carrier of EM for the water treatment is its rapid dissociation in flowing water, reducing their effectiveness. To overcome this problem, proper amount of hardener was added to soil ball, which delayed dissociation time to 7 days and resulted in dispersion in a more narrow area compared with the unmodified traditional soil ball, which dissociated in 2 days and dispersed completely throughout the water. Based on this result, we can expect that the improved soil ball containing hardener and EM may be more effective in stream water treatment by remaining stable longer in flowing water.
We used whole metagenome sequencing technique for microbial community characterization of soil balls to validate result and expend knowledge. This technique has the advantages of high sensitivity and no limitation of cultivability (Malik et al. 2008). Compared with 16S rRNA gene amplifying sequencing, whole metagenome sequencing of environment sample is superior in that the sequencing is direct and covers the entire metagenomic DNA, including 16S rRNA genes. Thus, the whole metagenome sequencing not only detects the complete microbial community but can also help in gene mining, revealing different metabolic pathways.
Based on the KEGG database, both traditional and improved soil balls have sufficient number of genes responsible for xenobiotic degradation and their related pathways. EMs are used for such general purposes, such as malodor removal, wastewater improvement, pet skin protection, dishwashing, the remediation of crude oil-contaminated water, and as PGPR for improving plant growth and crop yield (Teruo and James 1994). Similarly, soil balls with EM were used for the remediation of contaminated harbor sediments, as well as a biofilm filter for removal of total phosphorus from farm wastewater using Chromobacterium LEE-38 at a pilot plant (Shin et al. 2006).
Conclusions
Results of the present study led us to the conclusion that the soil ball with 0.75 % hardener and neutral pH (pH 7.3) effectively improved the water quality of the ponds, lakes, and streams. Metagenomic analysis of the microbiota of the improved soil ball (with 0.75 % hardener) revealed the flourishing prokaryotic and eukaryotic microbial communities, enhancing the structural stability of the soil ball that are important for the water treatment application. To the best of our knowledge, it is the first study to reveal the microbial diversity and associated metabolic pathways in soil ball through the modern approach of whole metagenomic DNA sequencing and metabolic pathway analysis. Based on our results, we suggest that the improved soil ball with specific EM with sufficient efficiency for various purposes might have potential in wastewater treatment.
References
APHA (1998) Standard methods for the examination of water and wastewater, 20th edn, Washington, DC: American Public Health Association, American Water Work Association, Water Environment Federation 252
Buckley DH, Graber JR, Schmidt TM (1998) Phylogenetic analysis of nonthermophilic members of the kingdom Crenarchaeota and their diversity and abundance in soils. Appl Environ Microbiol 64:4333–4339
Bundy JG, Paton GI, Campbell CD (2002) Microbial communities in different soil types do not converge after diesel contamination. J Appl Microbiol 92:276–288
Bustin S (2002) Quantification of mRNA using real-time reverse transcription PCR (RT-PCR): trends and problems. J Mol Endocrinol 29:23–39
Christensen H, Hansen M, Sørensen J (1999) Counting and size classification of active soil bacteria by fluorescence in situ hybridization with an rRNA oligonucleotide probe. Appl Environ Microbiol 65:1753–1761
Denman S, McSweeney C (2005) Quantitative (real-time) PCR. In: Makkar HS, McSweeney C (eds) Methods in gut microbial ecology for ruminants. Springer, The Netherlands, pp 105–115
Ekpeghere KI, Kim B-H, Son H-S, Whang K-S, Kim H-S, Koh S-C (2012) Functions of effective microorganisms in bioremediation of the contaminated harbor sediments. J Environ Sci Health A 47:44–53
Fierer N, Jackson JA, Vilgalys R, Jackson RB (2005) Assessment of soil microbial community structure by use of taxon-specific quantitative PCR assays. Appl Environ Microbiol 71:4117–4120
Gilbride KA, Lee DY, Beaudette LA (2006) Molecular techniques in wastewater: understanding microbial communities, detecting pathogens, and real-time process control. J Microbiol Methods 66:1–20
Giovannoni SJ, Britschgi TB, Moyer CL, Field KG (1990) Genetic diversity in Sargasso Sea bacterioplankton. Nature 345:60–63
Green JL et al (2004) Spatial scaling of microbial eukaryote diversity. Nature 432:747–750
Grover M, Ali SZ, Sandhya V, Rasul A, Venkateswarlu B (2011) Role of microorganisms in adaptation of agriculture crops to abiotic stresses. World J Microbiol Biotechnol 27:1231–1240
Heid CA, Stevens J, Livak KJ, Williams PM (1996) Real time quantitative PCR. Genome Res 6:986–994
Hesham Ael L, Qi R, Yang M (2011) Comparison of bacterial community structures in two systems of a sewage treatment plant using PCR-DGGE analysis. J Environ Sci (China) 23:2049–2054
Higa T (1998) An earth saving revolution: a means to resolve our world’s problems through effective microorganisms (EM). Sunmark Publishing, Inc., Tokyo, Japan
Higa T, Parr JF (1994) Beneficial and effective microorganisms for a sustainable agriculture and environment (vol. 1). Atami, Japan
Javaid A (2010) Growth and yield response of wheat to EM (effective microorganisms) and parthenium green manure. Afr J Biotechnol 9:3373
Kariminiaae-Hamedaani H-R, Kanda K, Kato F (2003) Wastewater treatment with bacteria immobilized onto a ceramic carrier in an aerated system. J Biosci Bioeng 95:128–132
Malik S, Beer M, Megharaj M, Naidu R (2008) The use of molecular techniques to characterize the microbial communities in contaminated soil and water. Environ Int 34:265–276
Mielczarek AT, Saunders AM, Larsen P, Albertsen M, Stevenson M, Nielsen JL, Nielsen PH (2013) The microbial database for Danish wastewater treatment plants with nutrient removal (MiDas-DK)—a tool for understanding activated sludge population dynamics and community stability. Water Sci Technol 67:2519–2526
Moura A, Tacão M, Henriques I, Dias J, Ferreira P, Correia A (2009) Characterization of bacterial diversity in two aerated lagoons of a wastewater treatment plant using PCR-DGGE analysis. Microbiol Res 164:560–569
Muyzer G, de Waal EC, Uitterlinden AG (1993) Profiling of complex microbial populations by denaturing gradient gel electrophoresis analysis of polymerase chain reaction-amplified genes coding for 16S rRNA. Appl Environ Microbiol 59:695–700
NIAST (2000) Method of soil and plant analysis. National Institute of Agricultural Science and Technology (NIAST), Suwan
Nicol GW, Leininger S, Schleper C, Prosser JI (2008) The influence of soil pH on the diversity, abundance and transcriptional activity of ammonia oxidizing archaea and bacteria. Environ Microbiol 10:2966–2978
Nogales B, Moore ER, Llobet-Brossa E, Rossello-Mora R, Amann R, Timmis KN (2001) Combined use of 16S ribosomal DNA and 16S rRNA to study the bacterial community of polychlorinated biphenyl-polluted soil. Appl Environ Microbiol 67:1874–1884
Ranjard L, Richaume A (2001) Quantitative and qualitative microscale distribution of bacteria in soil. Res Microbiol 152:707–716
Ravenschlag K, Sahm K, Knoblauch C, Jorgensen BB, Amann R (2000) Community structure, cellular rRNA content, and activity of sulfate-reducing bacteria in marine arctic sediments. Appl Environ Microbiol 66:3592–3602
Rhee SK, Liu X, Wu L, Chong SC, Wan X, Zhou J (2004) Detection of genes involved in biodegradation and biotransformation in microbial communities by using 50-mer oligonucleotide microarrays. Appl Environ Microbiol 70:4303–4317
Ritz K et al (2004) Spatial structure in soil chemical and microbiological properties in an upland grassland. FEMS Microbiol Ecol 49:191–205
Rousk J, Brookes PC, Bååth E (2009) Contrasting soil pH effects on fungal and bacterial growth suggest functional redundancy in carbon mineralization. Appl Environ Microbiol 75:1589–159
Sanz JL, Köchling T (2007) Molecular biology techniques used in wastewater treatment: an overview. Process Biochem 42:119–133
Shin S-E, Choi D, Lee C-B, Cha W-S (2006) Phosphorus removal in pilot plant using biofilm filter process from farm wastewater. Biotechnol Bioprocess Eng 11:325–331
Small J, Call DR, Brockman FJ, Straub TM, Chandler DP (2001) Direct detection of 16S rRNA in soil extracts by using oligonucleotide microarrays. Appl Environ Microbiol 67:4708–4716
Teruo H, James FP (1994) Beneficial and effective microorganisms for a sustainable agriculture and environment. International Nature Farming Research Centre, Nagano
Torsvik V, Salte K, Sorheim R, Goksoyr J (1990) Comparison of phenotypic diversity and DNA heterogeneity in a population of soil bacteria. Appl Environ Microbiol 56:776–781
Zakaria Z, Gairola S, Shariff NM (2010) Effective microorganisms (EM) technology for water quality restoration and potential for sustainable water resources and management. Biol Programme Sch Distance Educ 11800:1–8
Acknowledgments
This research was sponsored by the Korea Ministry of Environment as the Eco-Innovation project.
Author information
Authors and Affiliations
Corresponding author
Ethics declarations
The present research did not involve human participants and/or animals.
Conflict of interest
The authors declare no potential conflicts of interest.
Additional information
Responsible editor: Robert Duran
Electronic supplementary material
Below is the link to the electronic supplementary material.
Fig. A1
Soil ball dissociation in water tank after 7 days in controlled flow. a Soil ball containing 0 % (w/w) 3CaO∙SiO2 and b soil ball containing 0.75 % (w/w) 3CaO∙SiO2. (JPEG 134 kb)
Fig. A2
The shape of soil balls. a Soil ball making with loess and b soil ball making with send and zeolite. (JPEG 250 kb)
Fig. A3
Composition of microbial community pie charts of soil balls. a Traditional soil ball. b Improved soil ball with 0.5 % hardener. c Improved soil ball with 0.75 % hardener. (JPEG 70 kb)
Rights and permissions
About this article
Cite this article
Park, GS., Khan, A.R., Kwak, Y. et al. An improved effective microorganism (EM) soil ball-making method for water quality restoration. Environ Sci Pollut Res 23, 1100–1107 (2016). https://doi.org/10.1007/s11356-015-5617-x
Received:
Accepted:
Published:
Issue Date:
DOI: https://doi.org/10.1007/s11356-015-5617-x