Abstract
Resource-conserving irrigation and fertilizer management practices have been developed for rice systems which may help address water quality concerns by reducing N and P losses via surface runoff. Field experiments under three treatments, i.e., farmers’ conventional practice (FCP), alternate wetting and drying (AWD), and AWD integrated with site-specific nutrient management (AWD + SSNM) were carried out during two rice seasons at two sites in the southwest Yangtze River delta region. Across site years, results indicated that under AWD irrigation (i.e., AWD and AWD + SSNM), water inputs were reduced by 13.4 ~ 27.5 % and surface runoff was reduced by 30.2 ~ 36.7 % compared to FCP. When AWD was implemented alone, total N and P loss masses via surface runoff were reduced by 23.3 ~ 30.4 % and 26.9 ~ 31.7 %, respectively, compared to FCP. However, nutrient concentrations of surface runoff did not decrease under AWD alone. Under AWD + SSNM, total N and P loss masses via surface runoff were reduced to a greater extent than AWD alone (39.4 ~ 47.6 % and 46.1 ~ 48.3 % compared to FCP, respectively), while fertilizer inputs and N surpluses significantly decreased and rice grain yields increased relative to FCP. Therefore, by more closely matching nutrient supply with crop demand and reducing both surface runoff and nutrient concentrations of surface runoff, our results demonstrate that integration of AWD and SSNM practices can mitigate N and P losses via surface runoff from rice fields while maintaining high yields.
Similar content being viewed by others
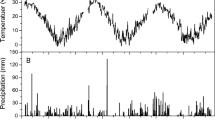
Explore related subjects
Discover the latest articles, news and stories from top researchers in related subjects.Avoid common mistakes on your manuscript.
Introduction
China is the world’s leading rice producer (~30 million hectares) and also the leading consumer of water and fertilizers for rice production (Timsina and Connor 2001). High inorganic fertilizer application rates can contribute to low nutrient use efficiencies for rice systems, resulting in high N and P losses and increased environmental impacts for surrounding ecosystems (Zhu et al. 2003). Degradation of water resources has become a major problem in intensive rice cropping regions of China, including those in the main rice producing area in the Yangtze River delta (Chen et al. 2010; Liang et al. 2008; Zhang et al. 2004). Along with domestic sewage and other agricultural activities (Qin et al. 2007), excessive nutrient loading by surface runoff from flooded rice fields into lakes, streams, and groundwater has contributed to serious surface water pollution and frequent algal blooms in Taihu Lake (Xu et al. 2009).
Surface runoff is the dominant pathway for N and P transport from flooded rice fields in addition to vertical leaching and lateral seepage (Liang et al. 2007; Pothig et al. 2010; Yoon et al. 2003; Zhao et al. 2012). Surface runoff generally occurs after heavy precipitation events or during field drainage, and has been shown to contribute up to 86 % of cumulative N losses during the rice growing season when surface runoff and leaching were monitored (Zhao et al. 2012). In contrast to conventional flooding, water saving practices such as intermittent or moistening irrigation often minimize runoff due to the maintenance of lower floodwater levels in fields, thereby increasing field buffering capacity, reducing runoff and, potentially, nutrient losses by nearly 50 ~ 60 % (Gao et al. 2002). Recently, alternate wetting and drying (AWD) irrigation has been widely adopted to reduce water use in rice cropping systems (Tabbal et al. 2002; Tuong et al. 2005). Numerous field experiments under AWD have demonstrated that it can decrease total water inputs by approximately 20 % and enhance water use efficiency by 36 ~ 55 % (Matsuo and Mochizuki 2009; Zhang et al. 2009). Some authors also report that AWD can have a positive effect on grain yield (Belder et al. 2004; Yang et al. 2009; De Vries et al. 2010; Zhang et al. 2008). Importantly, AWD allows for floodwater levels in the field to drop below the soil surface before the next irrigation is needed. Thus, it potentially has the capacity to buffer excess precipitation delivered by heavy storms, such that water levels in the field are less likely to become higher than the bund which can trigger surface runoff (Bouman and Tuong 2001). Previous work therefore suggests that AWD can be implemented to reduce irrigation water inputs and at the same time to minimize N and P losses from surface runoff events. Despite the relevance of this topic for protecting water quality in the rice growing regions of the Yangtze River delta, there is a lack of detailed research evaluating the potential for AWD to mitigate N and P losses via surface runoff from rice fields.
It is well-known that in most cropping systems, N and P fertilizers are applied in excess (Albiac 2009), with only about 20 % of fertilizer N (and P) making it into our diet (Deluca 2009). In rice cropping systems, it has been reported that fertilizer use could be cut by two thirds without sacrificing rice grain yield (Wang et al. 2001). High fertilizer rates have been shown to greatly increase the risk of nutrient losses in a number of studies (Wang et al. 2007; Gao et al. 2002). For example, in this region, Ju et al. (2009) determined that N losses from rice cropping systems under the N rate of 300 kg ha−1 were 1.7 times greater than sites where the optimum N rate of 200 kg ha−1 was applied. Furthermore, Wang et al. (2003) reported that fertilizer N use efficiency was generally very low in this study region and that the recovery of applied fertilizer N was usually less than 20 %. Accordingly, N inputs should be more accurately matched to crop N requirements to reduce nutrient losses and prevent further degradation of water supplies. The plant-based strategy of site-specific nutrient management (SSNM) has been shown to be an effective means of lowering fertilizer application rates and increasing N use efficiency (Dobermann et al. 2003; Pampolino et al. 2007; Peng et al. 2010). Generally, SSNM practices allow a farmer to achieve balanced plant nutrition for a specific field and season by estimating optimal fertilizer rates which can increase mineral fertilizer use efficiency (Dobermann et al. 2003). SSNM practices are designed to take into account soil nutrient supplying capacity, reasonable grain yield targets, past management history, and corresponding crop nutrient requirements for specific rice cropping seasons as well as socioeconomic factors. The SSNM approach commonly includes the following steps: target yield selection, estimation of plant nutrient uptake requirements and nutrient use efficiencies to select the optimal N rate, and in-season adjustment of fertilizer N application timing and/or rate using a chlorophyll meter (SPAD) or leaf color chart (LCC) (Witt and Dobermann 2002; Pampolino et al. 2007). Results from a review literature (Peng et al. 2010) reported that SSNM can, on average, reduce N fertilizer by 32 % and increase grain yield by 5 % compared with farmers’ N practices. However, to date, the SSNM approach has seen limited adoption in Chinese rice systems, which is mainly attributed to two reasons. First, SSNM can require more time than conventional management. For example, the frequency of N fertilizer application might increase from the normal three times to four or more times during a typical rice-growing season. Second, estimating the in-season leaf N content of a crop is a key step of SSNM, thus farmers need to learn how to use a SPAD or LCC. However, despite the LCC being an easy-to-use and inexpensive diagnostic tool for monitoring the relative greenness of a rice leaf as an indicator of plant N status (Alam et al. 2005), this step can mean SSNM is more knowledge intensive, which can limit adoption.
At present, it is increasingly accepted that integration of water and fertilizer management practices can play an important role in mitigating nutrient losses from rice cropping systems (Shao 2010). Few if any published reports have evaluated the effects of the combination of AWD and SSNM on the environment due to the transfer of nutrient load in the runoff to the surroundings. In the present study location, where sufficient precipitation occurs throughout the rice growing period (June to October) and typhoons often trigger surface runoff from rice fields, we hypothesized that (1) N and P losses via surface runoff could be decreased under AWD management and (2) that fertilizer rates and associated N and P losses could be further reduced under integrated AWD and SSNM management. This study aimed at quantifying irrigation and fertilizer inputs, assessing N and P losses via surface runoff, and yield of rice systems under AWD and SSNM practices in the Yangtze River delta.
Materials and methods
Site description and experimental design
The experiment was carried out at Jingshan (JS) Agricultural Research Station (30°21′ N, 119°53′ E) and Shuangqiao (SQ) Agricultural Research Station (30°50´ N, 120°40´ E) (Fig. 1). Both are representative of the rice production area in the southwest Yangtze River delta region of China. Rice–wheat and rice–rape rotations are common in this region. Both sites possessed the typical climate characteristics of the coastal plain area. Soils at the SQ site are classified as gleyed paddy soil (clay loam, mixed, mesic Mollic Endoaquepts) and the JS site as hydragic paddy soil (silt loam, mixed, mesic Mollic Endoaquepts). Soil pH, total N, total P, organic matter, bulk density, sand content, and clay content at the JS were 7.05, 2.38, 1.10, and 28.6 g kg−1, 1.29 g cm−3, 16.1 %, and 38.3 %, respectively. The SQ soil had a lower pH (6.35) and sand content (13.0 %) but higher total N (2.71 g kg−1), total P (1.59 g kg−1), organic matter (35.3 g kg−1), bulk density (1.48 g cm−3), and clay content (51.3 %) than the JS site. The water vertical percolation rate at the JS (7.5 mm per day) is about 1.5-folds higher than SQ (5.0 mm per day).
Field experiments were carried out from July to October each year and included two seasons of rice (Oryza sativa L.) grown at the JS site (2009 and 2010) and one season at the SQ site (2010). At both sites, the treatments were arranged in a randomized complete block design with three replications. The experimental field at each site consisted of nine plots (0.6 ha each). Three treatments representing different water and fertilizer regimes were applied at each site: (1) farmers’ conventional practice (FCP), (2) AWD, and (3) AWD integrated with SSNM (AWD + SSNM). Water and nutrient management in each treatment are described in the next section. Ridges were established between plots to isolate them from adjacent plots. Ridges (300-mm wide at the base and 200-mm high) were covered with plastic sheeting that was buried into the soil to a depth of 150 mm in order to prevent water movement from and to each plot.
Crop, nutrient, and water management
In all seasons, 25-day-old rice seedlings were transplanted at 150 × 150 mm spacing. For FCP and AWD treatments, urea–N, superphosphate–P, and KCl–K fertilizers were applied at 240 kg N ha−1, 55 kg P2O5 ha−1, and 115 kg K2O ha−1, respectively, at the JS site and at 200 kg N ha−1, 45 kg P2O5 ha−1, and 110 kg K2O ha−1 at the SQ site. For the FCP and AWD treatments, fertilizer N was always applied in three splits: 35 % at ET (early tillering) stage which corresponded to 13–15 days after transplanting (AT), 25 % at PT (peak tillering) stage (31–35 days AT), and 40 % at booting stage (57–60 days AT). Phosphorus and potassium fertilizers were applied as a single dose at PT (31–32 days AT). In the AWD + SSNM treatment, urea–N, superphosphate–P, and KCl–K fertilizer application rates were adjusted by standardized leaf color charts (LCC) according to procedures described by Alam et al. (2005). Briefly, the LCC approach included three steps as follows: (1) at least ten disease-free rice plants in a field with uniform plant population were randomly selected; (2) the middle part of the topmost fully expanded leaf from each plant was placed on the leaf chart to compare the leaf color with the color panels of the LCC; (3) the leaf color was measured to determine the average LCC reading for the selected leaves. Fertilization schedule and total N, P, and K fertilizer rates for FCP, AWD, and AWD + SSNM are presented in Tables 1 and 2. Irrigation practices for the FCP treatment followed typical methods for flooded rice in the region. Each plot was irrigated until the field water (also called floodwater) depth reached 70 mm aboveground. The field water level was allowed to drop to 10 mm before it was irrigated again. Thus, floodwater depths in the FCP plots fluctuated from 10 to 70 mm until rice maturity and the field was drained 30 days prior to harvest. In AWD treatments, irrigation management followed previously published recommendations (Cabangon et al. 2004). After initial irrigation to 70 mm aboveground, AWD and AWD + SSNM plots were kept flooded until 15 days AS to allow seedlings to recover from transplanting and for weed control. Beyond 15 days AS, the floodwater level was allowed to fall below the soil surface and reach a depth of approximately −120 mm before irrigation was applied, typically back up to 70 mm. In AWD and AWD + SSNM treatments, the depth of standing water was measured daily from instruments described by Bouman and Tuong (2001). Briefly, hollow and bottomless PVC tubes (200 mm in diameter and 400 mm in length) were perforated with small holes 5 mm in diameter and spaced 20-mm apart. Each tube was inserted in the soil to a depth of 250 mm and the soil within the tube was removed. Water in the fields, either above or under the soil surface, could thus enter the tubes via the perforation. Throughout the growing season, irrigation events were scheduled for AWD and AWD + SSNM plots after the depth of floodwater was determined in the PVC tubes and considerations were made for local weather forecasts, soil characteristics, and anticipated water demand by the rice crop.
Water and plant sampling
A mini-meteorological station (HOBO-U30, America, Onset Computer Co.) was placed at each experimental site for collecting climate data once an hour including precipitation, temperature, air pressure, and humidity. During each surface runoff event, water samples were collected from field plots using specially designed devices that were installed in bunds separating field plots from drainage ditches approximately 3 months before the start of the experiment. The design of this device was patented in China (no. 201010520499) and therefore had undergone rigorous testing prior to this experiment. In brief, each device was an oblong container fixed with a flat top surface fastened by two spindles. The container was divided into one water collection chamber and one flow measurement chamber by a vertical clapboard. The collection chamber consisted of three smaller spaces of the same size divided by two clapboards. The water inlet was positioned in the first space and fixed with a screen. The first and second spaces were connected at the bottom of the clapboard which created a large reservoir. The second and third spaces were connected in the upper portion of the clapboard to help control water flow. The water collection chamber was connected to the flow measurement chamber by two tubes of different sizes, each containing a flow-through water meter attached with 90° fittings to accurately record small and large runoff events. All runoff water exiting plots travelled through these devices throughout the season, which allowed for water samples to be obtained and the volume of drainage water to be quantified.
Water samples were preserved with several drops of H2SO4 and subjected to chemical analysis within 24 h of sampling. Concentrations of NH4 +, TN, PO4 3−, and TP were determined using a continuous flow analyzer (BRAN+LUEBBE, AA3, Germany).
At the end of the growing season, rice yields were determined by harvesting five quadrats each 1 m2 from each plot. Plant biomass and grains were oven-dried to a constant weight, and total N in plant tissues was determined by an Elemental Analyzer (Vario Max, Germany). Field apparent N surplus was estimated as total N applied minus crop N uptake.
Data analysis
Nutrient losses were calculated by multiplying the nutrient concentrations of water samples by the corresponding volume of surface runoff quantified at each sampling event. The cumulative amount of nutrients lost over the entire growing period was calculated as the sum of individual runoff events. Nutrient concentrations of irrigation and precipitation water were not determined in this study and therefore our N and P loss estimates include nutrient inputs from the environment (aside from inorganic fertilizers). A flow meter was applied to measure the volume of irrigation water input, and irrigation amounts (millimeter) in this study were determined by dividing the volume of irrigation input by field plot area. The unit of surface runoff was also expressed as millimeter to be consistent with irrigation and precipitation results, and was calculated for each event by dividing the total surface runoff volume by the plot area. The differences in field water depth, times and amounts of irrigation and surface runoff water, total fertilizer inputs, and NH4 +, TN, PO4 3−, and TP masses in surface runoff between sites and seasons were analyzed using the paired t test in SPSS software. The treatment differences between FCP, AWD, and AWD + SSNM for the above parameters were analyzed with ANOVA in SPSS software, and the resulting error mean squares were used to calculate the LSD values for mean separation.
Results
Irrigation inputs and surface runoff under AWD
Large differences in field floodwater profiles were observed between FCP and AWD treatments (Fig. 2). For example, four clear wet and dry cycles occurred under AWD in JS-2009 with the floodwater depth dropping to −120 mm in each cycle which contributed to a large savings in the number of irrigation events. As a result, in all seasons, the amount of irrigation water applied under AWD and AWD + SSNM was significantly reduced compared to FCP (p < 0.01) by 13.4 %, 14.3 %, and 27.5 % at JS-2009, JS-2010, and SQ-2010, respectively (Fig. 3). Aside from periods where floodwater depths were similar either during crop establishment or following heavy rainfall, AWD contributed to cutbacks in irrigation inputs, particularly during periods of non-continuous rainfall including 23 and 33 days AT for JS-2009 (Figs. 2 and 3). Similar results for floodwater fluctuation profiles under FCP and AWD were found at JS-2010 and SQ-2010 (Figs. 2 and 3). Meanwhile, the number of surface runoff events under AWD significantly decreased compared to FCP (p < 0.01), and the total volume of surface runoff water decreased by 36.7 %, 34.8 %, and 30.2 % in JS-2009, JS-2010, and SQ-2010, respectively (Fig. 3). As expected, irrigation and surface runoff amounts under AWD differed by year and site.
Fertilizer rate under AWD + SSNM
SSNM practices contributed to a clear reduction in N, P, and K fertilizer use for all seasons in this experiment compared to FCP, and across two sites, N, P, and K fertilizer inputs under AWD + SSNM were reduced by 23.3 %, 17.7 %, and 8.9 %, respectively (Table 1). Differences in fertilizer rate under AWD + SSNM and FCP were more related to site than season in this study.
Nutrient concentrations and losses
Profiles of nutrient concentrations for surface runoff fluctuated over the growing season, with surface runoff events frequently following precipitation events and triggering nutrient export in all treatments (Figs. 4 and 5). The periods of greatest nutrient flux generally occurred immediately following N, P, and K fertilizer applications at the peak tillering growth stage of rice and steadily decreased over the following 2 weeks (i.e., 35–50 days AT). The concentrations of NH4 +–N, TN, PO4 3−, and TP in surface runoff under AWD did not decrease compared to FCP for each season (p > 0.05). In contrast, when SSNM was integrated with AWD irrigation, NH4 +–N, TN, PO4 3−, and TP concentrations in surface runoff significantly were decreased to lower levels with respect to FCP (p < 0.01).
Under AWD, cumulative N losses were significantly lower than FCP (p < 0.01) for all seasons, with reductions of 30.4 %, 27.0 %, and 23.3 % for total N in JS-2009, JS-2010, and SQ-2010, respectively (Table 2), and 42.9 %, 27.2 %, and 23.5 %, respectively, for NH4 +–N in these seasons. Similar results revealed that P losses under AWD were also significantly lower than FCP (p < 0.01) for all seasons. The reduction of P losses was 26.9 %, 29.2 %, and 31.7 % for total P and 30.5 %, 33.3 %, and 30.0 % for PO4 3−–P in JS-2009, JS-2010, and SQ-2010, respectively (Table 2). Overall, the integrated AWD + SSNM better prevented nutrient losses than AWD alone, with NH4 +–N and TN losses being reduced by 47.2 ~ 54.5 % and 39.4 ~ 47.6 %, respectively, compared to FCP across site years. Meanwhile, corresponding PO4 3−–P and TP losses via surface runoff also decreased to a greater extent across site years under AWD + SSNM, ranging from 44.1 ~ 46.7 % and 46.1 ~ 48.3 %, respectively, compared to FCP (Table 2).
Rice grain yields and N surplus
Relationships between N surplus (estimated as N applied–N uptake) and rice grain yields were significantly different between FCP, AWD, and AWD + SSNM treatments (Fig. 6). Across all seasons, AWD + SSNM had the lowest N surplus (43 ~ 74 kg N ha−1) and highest rice grain yields. While yields for FCP were 7,125, 7,256, and 7,306 kg ha−1 in JS-2009, JS-2010, and SQ-2010, respectively, yields under AWD + SSNM increased significantly by 5.0 % (353 kg ha−1), 3.4 % (247 kg ha−1), and 3.1 % (229 kg ha−1), respectively. However, when AWD was implemented alone there were no differences in N surplus or rice grain yield compared to FCP (p > 0.05).
Discussion
Results from this study indicate that AWD irrigation practices contributed to considerable water savings relative to a continuous flood (Table 1; Fig. 3). Therefore, this technology represents a practical, cost-effective method to measure field water depths based on simple instrumentation and can easily be extended to rice growers as a tool to conserve water resources. This finding is consistent with previous reports and likely explains the widespread adoption of these practices that has occurred on farm in China (Li and Barker 2004). While in our study total irrigation inputs were reduced, approximately 8 ~ 15 % depending on the site, this value is lower than others where it has been shown that water savings of 24 ~ 38 % (Yao et al. 2012) or 40 % (Matsuo and Mochizuki 2009) are possible under AWD. This difference may be attributable to variation in precipitation during the growing season, which can represent a large fraction of total water inputs or differences in soil type, both of which can alter irrigation requirements. Nonetheless, the average reduction in water use in our study was similar to values of 13 % reported by Cabangon et al. (2004) and 15 ~ 18 % reported by Belder et al. (2004). Similar floodwater depths were only observed between AWD and FCP treatments during three unavoidable periods (Fig. 2): (1) the seedling recovery stage (days AT, 1 ~ 15), where field water depth was maintained in all treatments at approximately 50 mm to promote normal rice growth and crop establishment; (2) when heavy rainfall occurred continuously (for instance, during JS-2009 where precipitation events were concentrated within the period of 35 days AT to 60 days AT; and (3) the maturity stage where fields were drained at approximately 90 ~ 100 days AT as water was no longer required for crop growth. Outside of these periods, it may be possible to achieve greater water savings, especially if the water depth is allowed to drop 15 cm below the soil surface (e.g., Yao et al. 2012) and could be the focus of future work.
Water savings were achieved under AWD management in our study with simultaneous increases in environmental benefits (i.e., a reduction in nutrient losses) and no impact on yield. This is a significant finding and implies it may be possible to begin to address water quality concerns in the Yangtze River basin without negatively impacting the livelihood of farmers. The primary mechanism by which AWD irrigation reduced nutrient losses was by limiting surface runoff. This was generally possible because of reduced floodwater depths in rice fields (Fig. 2), which helped buffer against runoff events after heavy storms compared to a continuous flood. Consequently, the number of runoff events and total volume surface runoff under AWD was significantly lower than FCP treatments (Fig. 3). These results are in agreement with previous studies and indicate that field water management and corresponding volumes of runoff water are determining factors when assessing nutrient losses via surface runoff from crop fields (Buda et al. 2009; Krupa et al. 2011; Tuong et al. 2005). Both the profile of nutrient fluxes as well as the range of cumulative TN and TP losses via surface runoff fell within a similar range as previous work at the field scale (Jeon et al. 2005; Yoon et al. 2003), with cumulative TN losses in the FCP treatment being slightly higher than the average runoff value of 5 % estimated by Zhu and Chen (2002).
A primary drawback when AWD was implemented alone was that high nutrient concentrations were observed in surface runoff, especially within a short time after fertilizer application (Figs. 4 and 5). This can mainly be attributed to lower floodwater depths maintained under AWD which tended to concentrate nutrients in the shallow floodwater (Li et al. 2008). Although AWD was able to significantly reduce cumulative nutrient losses via surface runoff compared to FCP, high nutrient concentrations represent a potential trade-off of shallow water management (i.e., the risk of nutrient losses remains and may increase when precipitation events trigger surface runoff). Moreover, given the high temperatures observed during the rice growing season, nutrient losses through other pathways such as NH3 volatilization or nitrification–denitrification may also be intensified under AWD, which might ultimately hinder nutrient use efficiency (Dong et al. 2012).
In addition to water management, N and P losses were closely related to fertilizer application rates and timing. For all treatments, fertilizer applications were scheduled to avoid serious rainfall events, lowering the risk of high nutrient losses via surface runoff following heavy precipitation. While it is common practice in the study region for rice growers to apply about 60 % of the total fertilizer rate in the first 10 ~ 20 days to promote rice tillering (Liang et al. 2007; Wang et al. 2009), during this period the rate of nutrient uptake by rice is quite low, thus in this experiment the majority of N and P fertilizers were applied at 31 ~ 35 days AT. Despite these efforts, large precipitation events caused considerable nutrient flows into drainage trenches following fertilizer N and P applications (Fig. 3). However, SSNM practices helped decrease overall fertilizer rates and better synchronize nutrient supply with rice crop demand, thereby lowering nutrient concentrations in floodwater during the period immediately following fertilizer applications and for the remainder of the growing season. The reduction in fertilizer use achieved with SSNM practices in this study is supported by the findings of Wang et al. (2003) for a field experiment conducted in this region, where approximately 30 % less fertilizer was needed and a slight yield increase observed under SSNM practices. Thus, while total nutrient losses were reduced by roughly 20 ~ 30 % under AWD alone (as discussed above this was mainly due to decreased surface runoff), when SSNM was integrated with AWD: (1) surface runoff was effectively controlled following storm events, (2) fertilizer application rates were significantly reduced, and (3) nutrient concentrations of runoff and corresponding N and P losses were reduced by roughly 40 ~ 50 % compared to FCP. These results are noteworthy and to our knowledge the first to document the potential for AWD + SSNM practices to conserve water inputs and minimize nutrient losses while increasing yields. Given that fertilizer rates are known to be high and decreased fertilizer inputs will help protect water quality, these results have strong implications for rice crop management in this region (Huang and Zhu 2012; Ju et al. 2009; Qin et al. 2007).
As found elsewhere, our study indicates that nutrient losses via surface runoff are dependent on many factors including crop growth conditions, precipitation, soil type and related infiltration rate, and field management (Buda et al. 2009; Sigua et al. 2010; Wang et al. 2011). For example, at the same site (JS) with same soil and nutrient management, there was a significant difference in the losses of NH4 +–N, TN, PO4 3−, and TP in surface runoff from 2009 to 2010. This may be explained by slightly higher precipitation and drainage amounts in 2010, as well as slightly higher N and P rates for the AWD + SSNM treatment. In contrast, significant differences were not observed for losses of NH4 +–N, TN, PO4 3−, and TP between JS and SQ sites in 2010. This might be due to the interaction between precipitation and fertilizer rates at these sites, where precipitation at the SQ site was significantly higher than that at the JS site and contributed to 20 % more surface runoff (p < 0.01), whereas N and P fertilizer rates decreased by 16 ~ 18 %, potentially offsetting any increase due to increased runoff. In general, it has been shown that substantial surface runoff events will drive N and P losses more than an increase or decrease in fertilizer rate (Yoon et al. 2003).
In the present study, sufficient precipitation and good water retention by soil prevented water stress from occurring even when the water depth in dropped to −120 mm below the soil surface. As a result, rice grain yields for AWD treatments (AWD and AWD + SSNM) were not significantly different from FCP (Fig. 4). Previous findings have also shown that rice yield does not suffer and may even increase when the soil water potential at a shallow soil depth of 15 cm is maintained above −10 kPa (Matsuo and Mochizuki 2009; Yang et al. 2009). Most farmers focus their efforts on attaining yield and tend to overlook the benefits of fertilizer and water savings (Ju et al. 2009), let alone the adverse environmental impacts. Considering this, it is increasingly important to develop practical tools and promote farmer cooperation with researchers if water and fertilizer management practices to protect water quality are to be adopted (Albiac 2009; Liang et al. 2007). Based on our results, the integrated AWD + SSNM management provides a promising and practical method to control nutrient losses, reduce surplus N, and increase grain yields compared to FCP and AWD alone. Two reasons likely contribute to this effect. First, with AWD + SSNM the rice field received a remarkably lower N rate yet still had an N surplus of 43 ~ 74 kg N ha−1, indicating N was more than sufficient for rice growth. In contrast, high N surpluses under AWD and FCP likely increased ineffective tillering of rice rather than translated to grain yield (Fan et al. 2005). Second, although N losses by surface runoff in AWD were smaller than those from FCP, N losses by other pathways such as volatilization might have been intensified with shallow water management (Li et al. 2008). Thus, with SSNM practices the timing of fertilizer application was likely improved to maximize crop N uptake and minimize losses, thereby resulting in greater yield despite a reduction in total N inputs.
Based on our findings, we suggest some technical guidelines for implementing AWD and SSNM practices as follows. AWD commonly involves the steps: (1) maintaining a 3 ~ 5-cm layer of standing water in the field to facilitate seedling recovery for around 2 weeks after planting; (2) inserting a PVC pipe (about 20 cm in diameter and 40 cm in length) with holes (5 mm in diameter, spaced 2 cm apart) into the rice field (15 cm above and 25 cm below the soil surface) to monitor groundwater levels; (3) starting the AWD irrigation regime at tillering stage; (4) reflooding the field to a 5 ~ 7-cm layer of standing water when the groundwater drops to 8 ~ 12 cm below the soil surface; and (5) continuing AWD cycles after flowering until harvest. Meanwhile, we suggest that SSNM may include the following steps: (1) selecting a yield goal (Peng et al. (2010) indicated 85 % of the maximum possible grain yield potential considering climatic conditions and no other limitations to crop growth) based on the variety-specific potential yield; (2) estimating plant nutrient uptake requirements based on yield goal and the indigenous N supply, and then calculating fertilizer rates to overcome deficiencies between indigenous N supply and crop N demands; (3) adjusting fertilizer N application dynamically through the use of LCC to accommodate field- and season-specific conditions, which helps ensure N is applied at the time and in the amount needed by the rice crop. Evaluating potential interactions between water and fertilizer N management was not the direct intent of our study, thus these suggestions should be considered with a degree of caution. However, Cabangon et al. (2011) did not detect any water by N interaction effects on grain yield or fertilizer N recovery efficiency when using the integrated AWD and SSNM approach, suggesting that with sufficient practice these guidelines may be generally applicable in flooded rice systems.
Future research may focus on further decreasing field water depths below the soil surface to optimal levels which could promote the buffering capacity of AWD management and reduce surface runoff. Besides surface runoff, mineral fertilizer addition released N and P rapidly into the paddy soil making them susceptible to vertical leaching and/or lateral seepage. Particularly for N, large amounts could also be lost via ammonia volatilization and denitrification. Therefore, more information is needed concerning the effects of integrated AWD + SSNM management on alternative N and P loss pathways. Moreover, to develop best management practices that have the potential to further mitigate agricultural non-point pollution and increase nutrient use efficiency, other nutrient sources such as rural wastewater and controlled release fertilizers should be assessed under AWD + SSNM.
Conclusions
Management practices that conserve water and fertilizer inputs in rice systems may also limit nutrient losses and protect water quality in the Yangtze River delta. Our field experiments provide evidence that integrated AWD + SSNM management is an effective method to save water, reduce N and P losses via surface runoff from rice fields, and maintain yields. When implemented alone, AWD practices reduced the total amount of irrigation water and lowered field water depths which buffered large precipitation events and decreased the number and total volume of surface runoff events and corresponding N and P losses. However, high nutrient concentrations were observed in the surface runoff from AWD. On the contrary, when AWD was integrated with SSNM management, this decreased nutrient inputs which resulted in lower nutrient concentrations of surface runoff and substantial reductions in cumulative N and P losses. Importantly, the combination of AWD + SSNM significantly decreased N surpluses and increased rice grain yield compared to FCP, indicating that it may represent a win–win situation in terms of promoting agricultural production with minimal environmental impact.
References
Alam MM, Ladha JK, Khan SR, Foyjunnessa H-u-R, Khan AH, Buresh RJ (2005) Leaf color chart for managing nitrogen fertilizer in lowland rice in Bangladesh. Agron J 97:949–959
Albiac J (2009) Nutrient imbalances: pollution remains. Science 326(5953):665–665
Belder P, Bouman BAM, Cabangon R, Guoan L, Quilang EJP, Li YH, Spiertz JHJ, Tuong TP (2004) Effect of water-saving irrigation on rice yield and water use in typical lowland conditions in Asia. Agr Water Manage 65:193–210
Bouman BAM, Tuong TP (2001) Field water management to save water and increase its productivity in irrigated lowland rice. Agr Water Manage 49:11–30
Buda AR, Kleinman PJA, Srinivasan MS, Bryant RB, Feyereisen GW (2009) Effects of hydrology and field management on phosphorus transport in surface runoff. J Environ Qual 38(6):2273–2284
Cabangon RJ, Tuong TP, Castillo EG, Bao LX, Lu GA, Wang GH, Cui YL, Bouman BAM, Li YH, Chen CD, Wang JL (2004) Effect of irrigation method and N-fertilizer management on rice yield, water productivity and nutrient-use efficiencies in typical lowland rice conditions in China. Paddy Water Environ 2(4):195–206
Cabangon RJ, Castillo EG, Tuong TP (2011) Chlorophyll meter-based nitrogen management of rice grown under alternate wetting and drying irrigation. Field Crops Res 121:136–146
Chen DJ, Lu J, Wang HL, Shen YN, Kimberley MO (2010) Seasonal variations of nitrogen and phosphorus retention in an agricultural drainage river in East China. Environ Sci Pollut Res 17(2):312–320
De Vries ME, Rodenburg J, Bado BV, Sow A, Leffelaar PA, Giller KE (2010) Rice production with less irrigation water is possible in a sahelian environment. Field Crop Res 116:154–164
Deluca TH (2009) Nutrient imbalances: follow the waste. Science 326:665–665
Dobermann A, Witt C, Abdulrachman S, Gines HC, Nagarajan R, Son TT, Tan PS, Wang GH, Chien NV, Thoa VTK, Phung CV, Stalin P, Muthukrishnan P, Ravi V, Babu M, Simbahan GC, Adviento MAA (2003) Soil fertility and indigenous nutrient supply in irrigated rice domains of Asia. Agron J 95:913–923
Dong NM, Brandt KK, Sorensen J, Hung NN, Hach CV, Tan PS, Dalsgaard T (2012) Effects of alternating wetting and drying versus continuous flooding on fertilizer nitrogen fate in rice fields in the Mekong Delta, Vietnam. Soil Biol Biochem 47:166–174
Fan LC, Peng XL, Liu YY, Song TX (2005) Study on the site-specific nitrogen management of rice in cold area of northeastern China. Sci Agri Sin 38(9):1761–1766 (in Chinese)
Gao XJ, Hu XF, Wang SP, He BG, Xu SY (2002) Nitrogen losses from flooded rice field. Pedosphere 12(2):151–156
Huang Y, Zhu M (2012) The water quality of Lake Taihu and its protection. GeoJournal 40:39–44
Jeon JH, Yoon CG, Ham JH, Jung KW (2005) Model development for surface water drainage estimates from paddy rice fields. Paddy Water Environ 3:93–101
Ju XT, Xing GX, Chen XP, Zhang SL, Zhang LJ, Liu XJ, Cui ZL, Yin B, Christie P, Zhu ZL, Zhang FS (2009) Reducing environmental risk by improving N management in intensive chinese agricultural systems. PNAS 106:3041–3046
Krupa M, Tate KW, van Kessel C, Sarwar N, Linquist BA (2011) Water quality in rice-growing watersheds in a Mediterranean climate. Ag Ecosyst Environ 144:290–301
Li YH, Barker R (2004) Increasing water productivity for paddy irrigation in China. Paddy Water Environ 2(4):187–193
Li H, Liang XQ, Chen YX, Tian GM, Zhang ZJ (2008) Ammonia volatilization from urea in rice fields with zero-drainage water management. Agr Water Manage 95(8):887–894
Liang XQ, Chen YX, Li H, Tian GM, Ni WZ, He MM, Zhang ZJ (2007) Modeling transport and fate of nitrogen from urea applied to a near-trench paddy field. Environ Pollut 150:313–320
Liang XQ, Li H, Chen YX, He MM, Tian GM, Zhang ZJ (2008) Nitrogen loss through lateral seepage in near-trench paddy fields. J Environ Qual 37:712–717
Matsuo N, Mochizuki T (2009) Growth and yield of six rice cultivars under three water-saving cultivations. Plant Prod Sci 12:514–525
Pampolino MF, Manguiat IJ, Ramanathan S, Gines HC, Tan PS, Chi TTN, Rajendran R, Buresh RJ (2007) Environmental impact and economic benefits of site-specific nutrient management (SSNM) in irrigated rice systems. Agric Syst 93(1–3):1–24
Peng SB, Buresh RJ, Huang JL, Zhong XH, Zou YB, Yang JC, Wang GH, Liu Y, Hu R, Tang QY, Cui K, Zhang FS, Dobermann A (2010) Improving nitrogen fertilization in rice by site-specific N management. A review. Agron Sustain Dev 30:649–656
Pothig R, Behrendt H, Opitz D, Furrer G (2010) A universal method to assess the potential of phosphorus loss from soil to aquatic ecosystems. Environ Sci Pollut Res 17(2):497–504
Qin B, Xu P, Wu Q, Luo L, Zhang Y (2007) Environmental issues of Lake Taihu, China. Hydrobiologia 581:3–14
Shao W (2010) Effectiveness of water protection policy in china: a case study of jiaxing. Sci Total Environ 408:690–701
Sigua GC, Hubbard RK, Coleman SW (2010) Quantifying phosphorus levels in soils, plants, surface water, and shallow groundwater associated with bahiagrass-based pastures. Environ Sci Pollut Res 17(1):210–219
Tabbal DF, Bouman BAM, Bhuiyan SI, Sibayan EB, Sattar MA (2002) On-farm strategies for reducing water input in irrigated rice; case studies in the Philippines. Agr Water Manage 56:93–112
Timsina J, Connor DJ (2001) Productivity and management of rice-wheat cropping systems: issues and challenges. Field Crop Res 69:93–132
Tuong TP, Bouman BAM, Mortimer M (2005) More rice, less water-integrated approaches for increasing water productivity in irrigated rice-based systems in Asia. Plant Prod Sci 8:231–241
Wang GH, Dobermann A, Witt C, Sun QZ, Fu RX (2001) Performance of site-specific nutrient management for irrigated rice in southeast china. Agron J 93:869–878
Wang GH, Zhang QC, Huang CY (2003) SSNM-A new approach to increasing fertilizer N use efficiency and reducing N loss from rice fields. J Zhejiang Univ (Agr & Life Sci) 29:67–70 (In Chinese)
Wang GH, Zhang QC, Witt C, Buresh RJ (2007) Opportunities for yield increases and environmental benefits through site-specific nutrient management in rice systems of Zhejiang province, China. Agr Syst 94:801–806
Wang M, Yang JP, Xu W, Wang H, Sun JH (2009) Influence of nitrogen rates with split application on N use efficiency and its eco-economic suitable amount analysis in rice. J Zhejiang Univ (Agr & Life Sci) 35:71–76 (In Chinese)
Wang LQ, Liang T, Chong ZY, Zhang CS (2011) Effects of soil type on leaching and runoff transport of rare earth elements and phosphorous in laboratory experiments. Environ Sci Pollut Res 18(1):38–45
Witt C, Dobermann A (2002) A site-specific nutrient management approach for irrigated, lowland rice in Asia. Better Crops Interna 16:20–24
Xu H, Yang LZ, Zhao GM, Jiao JG, Yin SX, Liu ZP (2009) Anthropogenic Impact on Surface Water Quality in Taihu Lake Region, China. Pedosphere 19(6):765–778
Yang JC, Huang DF, Duan H, Tan GL, Zhang JH (2009) Alternate wetting and moderate soil drying increases grain yield and reduces cadmium accumulation in rice grains. J Sci Food Agr 89:1728–1736
Yao F, Huang J, Cui K, Nie L, Xiang J, Liu X, Wu W, Chen M, Peng S (2012) Agronomic performance of high-yielding rice variety grown under alternate wetting and drying irrigation. Field Crop Res 126:16–22
Yoon CG, Ham JH, Jeon JH (2003) Mass balance analysis in Korean paddy rice culture. Paddy Water Environ 1:99–106
Zhang WL, Wu SX, Ji HJ, Kolbe H (2004) Estimation of agricultural non-point source pollution in China and the alleviating strategies. I: estimation of agricultural non-point source pollution in China in early 21 century. Sci Agr Sin 37:1008–1017, In Chinese
Zhang H, Zhang S, Yang J, Zhang J, Wang Z (2008) Postanthesis moderate wetting drying improves both quality and quantity of rice yield. Agron J 100:726–734
Zhang H, Xue Y, Wang Z, Yang J, Zhang J (2009) An alternate wetting and moderate soil drying regime improves root and shoot growth in rice. Crop Sci 49:2246–2260
Zhao X, Zhou Y, Min J, Wang SG, Shi WM, Xing GX (2012) Nitrogen runoff dominates water nitrogen pollution from rice-wheat rotation in the Taihu Lake region of China. Agric Ecosyst Environ 158:1–11
Zhu ZL, Chen DL (2002) Nitrogen fertilizer use in China—contributions to food production, impacts on the environment and best management strategies. Nutr Cycl Agroecosyst 63:117–127
Zhu JG, Han Y, Zhang YL, Xing GX (2003) Nitrate distribution and denitrification in the saturated zone of paddy field under rice/wheat rotation. Chemosphere 50:725–732
Acknowledgments
We are grateful for grants from the National Natural Science Foundation of China (40901142, 21077088, and 40901111) and the National Key Science and Technology Project: Water Pollution Control and Treatment (no. 2012ZX07101-012). The authors would like to thank the technical assistance from the International Rice Research Institute (IRRI) and the anonymous reviewers for their valuable comments and suggestions during the manuscript modifications.
Author information
Authors and Affiliations
Corresponding author
Additional information
Responsible editor: Hailong Wang
Rights and permissions
About this article
Cite this article
Liang, X.Q., Chen, Y.X., Nie, Z.Y. et al. Mitigation of nutrient losses via surface runoff from rice cropping systems with alternate wetting and drying irrigation and site-specific nutrient management practices. Environ Sci Pollut Res 20, 6980–6991 (2013). https://doi.org/10.1007/s11356-012-1391-1
Received:
Accepted:
Published:
Issue Date:
DOI: https://doi.org/10.1007/s11356-012-1391-1