Abstract
Soil microorganisms play vital roles in recovering and maintaining the health of ecosystems, particularly in fragile Karst ecosystems that are easily degraded after cultivation. We investigated the composition and diversity of soil bacterial communities, based on RFLP and 16S rDNA sequencing, in a cropland, a naturally revegetated land with former cultivation disturbance and a primeval forest in the subtropical Karst of southwest China. Our results illustrated that Proteobacteria accounted for 44.8% of the 600 tested clones, making it the most dominant phylum observed. This phylum was followed by Acidobacteria and Planctomycetes for the three Karst soils analyzed. Compared with the primeval forest soil, the proportions of Proteobacteria were decreased by 30.2 and 37.9%, while Acidobacteria increased by 93.9 and 87.9%, and the Shannon-Wiener diversity indices and the physicochemical parameters declined in the cropland and the revegetated land, respectively. Among the three soils, the proportion of dominant bacterial phyla and the diversity indices in the revegetated land were similar to the cropland, implying the bacterial community in the cropland was relatively stable, and the after-effects of cultivation were difficult to eliminate. However, similar distributions of the four Proteobacteria subphyla were observed between the revegetated land and the primeval forest soil. Furthermore, the proportion of Rhizobiales belonging to α-Proteobacteria was sharply decreased with cultivation compared to the primeval forest soil, while a small cluster of Rhizobiales recurred with vegetation recovery. These results indicated that although the subphyla of the dominant bacterial phylum had some positive responses to 20 years of vegetation recovery, it is a slow process. Our results suggest that priority should be given to conserve the primeval forest and inoculation of functional microorganisms on the basis of vegetation recovery may be more effective for the restoration of Karst ecosystems after cultivation.
Similar content being viewed by others
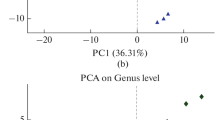
Explore related subjects
Discover the latest articles, news and stories from top researchers in related subjects.Avoid common mistakes on your manuscript.
Introduction
Karst terrains formed from carbonate minerals (e.g., limestone and dolomite) account for approximately 15% of the world’s land area and are a habitat for approximately 17% of the global population (Yuan and Chai 1988). Karst ecosystems are very fragile. The soil is typically thin, coarse, highly erosive and degenerative (He et al. 2008). Karst soil covering about 0.55 million km2 in southwest China, has been subjected to intensive anthropogenic disturbances, such as cultivation, deforestation, grazing and burning (Xiao and Weng 2007; Yao et al. 2001). The intensity of these disturbances rapidly expanded after 1970s due to pressure from the increasing population and overuse of land (Wang et al. 2004a, b). In the disrupted areas that have experienced long-term cultivation, the soil is no longer suitable for agriculture due to serious soil erosion and low yield that is partially caused by unbalanced fertilization. Overall, these factors accelerate Karst ecosystem degradation (Zhang et al. 2006a). Afterward, the land is often abandoned, leading to a natural recovery of vegetation. New cropland is then reclaimed from the forest with the Karst ecological environment rapidly deteriorating (Zhang et al. 2006b). Understanding the soil characteristics in this process, including physical, chemical and biological status, is a challenging task for sustainable agricultural development in subtropical Karst regions.
Soil microbial communities play an essential role in the recovery of ecosystems because microorganisms mediate many essential soil processes and functions (Chow et al. 2002; Bakermans and Madsen 2002; Mishra and Nautiyal 2009). These communities have been suggested to be indicators of health, sustainability and stability of ecosystems. For example, successful revegetation projects heavily depend on regeneration of the microbial community (DeGrood et al. 2005). Furthermore, by measuring the characteristics of the soil microbial community, the structure, status and quality of soil as well as the potential for successful vegetation recovery after soil degradation can be assessed (Badiane et al. 2001; Mummey et al. 2002). For degraded Karst ecosystems, existing studies have mainly focused on the changes of soil quality (including physical and biochemical properties) (Zhang et al. 2006a), plant diversity (He et al. 2008) and hydrological characteristics (Li et al. 2004). However, the effects of human disturbance on other ecological components, such as soil microbial communities, have not been well studied for Karst ecosystems. A previous study, based on DGGE analysis, found that soil bacterial diversity decreased with the process of land degradation in Karst (Zhang et al. 2006a), while the specific responses of bacterial community composition to cultivation disturbance were not elucidated.
The main objectives in the present study were to assess the influences of cultivation on the bacterial community of the Karst soils; to identify the after-effects of cultivation, including variations in bacterial communities from a cropland to a revegetated land, and the effectiveness of natural vegetation recovery on the restortion of bacterial community; and to analyze the reasons for the differences in soil bacterial communities, and give advice on effective restoration of disturbed Karst ecosystem.
Materials and methods
Sites and sampling
The current study was conducted at the Huanjiang Observation and Research Station for Karst Ecosystems in southwest China (24°44′ to 25°33′ N, 107°51′ to 108°43′ E). The area has a subtropical mountainous monsoon climate with a mean annual temperature of 18.5°C and an annual rainfall of 1,389 mm (He et al. 2008).
Three soil communities with a same calcareous soil were selected. The soil samples were collected from a cropland under a rotation system of maize/sweet potato or maize/soybean (KMS) which was reclaimed from primeval forest for approximately 100 years. Adequate N fertilizers were applied to this land with long-term application of 90–120 kg N ha−1 as urea and 10,000–15,000 kg ha−1 farmyard manure, while small amounts of P and K fertilizers were used. The soil samples from naturally revegetated land (KNR) were collected from a hillside that was previously a cropland with the same farming system as KMS 20 years prior. Currently, after 20 years of natural regrowth, it was covered by the dominant plant species of Miscanthus floridulus, Neyraudia reynaudiana, Microstegium nodosum and Imperata cylindrica. The above two ecosystems, with a distance of about 50 m, were located in the same landscape unit (24°50′ N, 107°55′ E). Due to the lack of primary forest in this landscape unit, an additional site was selected from the Mulun National Nature Reserve Park (25°07′ N, 108°00′ E), where primary forest (KPF) has grown over centuries without human disturbance (no farming record). Based on the field investigation, this ecosystem had higher plant diversity with dominant species including Zenia insignis Chun, Itoa orientalis Hemsley, Mallotus japonicus and Handeliodendron bodinieri in the tree layer; Bauhinia championii, Maesa japonica in the shrub layer; and Calocedrus macrolepis Kurz and Microlepia marginata in the herb layer.
Three 20 m × 20 m quadrats were selected from each ecosystem in November 2007. For each quadrat, five to eight soil cores with a diameter of 10 cm were collected from a depth of 0–20 cm and mixed to form one replicate. Each soil sample was then divided into two portions. One portion was placed into sterilized aluminum foil and then immediately submerged in liquid nitrogen for the analysis of the bacterial community, and the other was used for analysis of soil physicochemical properties. Meanwhile, three undisturbed soil samples were collected from each quadrat for soil aggregate analyses.
Analysis of the soil physicochemical properties
Soil organic matter, total nitrogen (N) and total phosphorus (P) were measured via a K2CrO7-H2SO4 oxidation procedure, the Kjeldahl method (Bremmer 1965) and the NaOH fusion method (Smith and Bain 1982), respectively. Soil pH was determined using a suspension of the sample in water at a ratio of 1:2.5 (w/v) and measured with a Mettler Toledo 320 pH meter (Mettler-Toledo Ltd., China). The composition of water-stable aggregates was determined by the previously published method of Kemper et al. (Kemper and Rosenau 1986).
DNA extraction, PCR, cloning and RFLP
Genomic DNA was directly extracted from the lyophilized soil samples according to the method of Chen et al. (2010). For each ecosystem, an equal amount of DNA from the three quadrats of an ecosystem were pooled into a mixture with three parallel DNA extractions for each soil sample. The 16S rDNA fragments (approximately 1,500 bp) were amplified with 8F and 1492R universal primers (Weisburg et al. 1991). Three parallel PCR reactions were processed for each DNA mixture, and the PCR products were pooled and then purified using the Wizard SV Gel and PCR Clean-Up System kit (Promega). The PCR products for each ecosystem were cloned into the pGEM-T vector (Promega) following the manufacturer’s protocol. After checking the inserted 16S rDNA fragments via PCR with the Sp6 and T7 primers, a total of 200 PCR products from positive clones in each clone library were then simultaneously digested with the Hind III and Hae III restriction enzymes (TaKaRa) according to the manufacturer’s instructions. The restriction fragments were then separated by horizontal gel electrophoresis of 2.5% agarose gels containing ethidium bromide (0.5 mg l–1) in 0.5 × TBE buffer (45 mM Tris, 45 mM boric acid, 1 mM EDTA (pH 8.3)). The generated Restriction Fragment Length Polymorphism (RFLP) banding profiles were initially visualized and then analyzed using LabWorks software (version 3.0). Fragments shorter than 150 bp were not taken into consideration because they were close to the DNA detection threshold using agarose gel electrophoresis and ethidium bromide staining (Izumi et al. 2003). Clones with similar RFLP patterns were referred to an operational taxonomic unit (OTU) (Zhou et al. 2003).
Sequencing and taxonomic assessment
One clone from each OTU was randomly selected for sequencing with the Sp6 and T7 primers (Songon Biotech, Shanghai). All sequences were assembled with GENtle 1.9.4 software and aligned using Clustal X 1.83. A phylogenetic tree was constructed using MEGA version 4.0 (Tamura et al. 2007). Taxonomic assignment of sequences was assigned using RDPquery (http://rdp.cme.msu.edu) with similarity cutoff values of 80, 85, 90 and 92% for phylum, class, order and family designations, respectively (DeSantis et al. 2007). All sequences were deposited into GenBank under the accession numbers, EU881234–EU881358 for KMS, EU881168–EU881233 for KNR and EU881088–EU881167 for KPF.
Statistical analysis
The Shannon-Wiener diversity index was used to measure the bacterial diversity (Shannon and Weaver 1949). Coverage of each clone library was estimated as previously described by Mullins et al. (1995). Rarefaction curves calculated by the aRarefactWin program were used to determine if the libraries were saturated (Holland 2004). To determine the likelihood that two bacterial communities were different, ∫-LIBSHUFF analysis (http://libshuff.mib.uga.edu/) (Singleton et al. 2001) was performed. For the ∫-LIBSHUFF analysis, if a p-value was at or below 0.05 there was a 95% confidence that the clone libraries differed in bacterial community at the nucleotide level. Simultaneously, the significant differences of soil properties were analyzed using SPSS 10.5 software for Windows with one-way ANOVA.
Results
Soil physicochemical properties
Soil physiochemical properties changed greatly after long-term cultivation (Table 1). The contents of soil organic matter, total N and total P were significantly higher in KPF when compared to KMS and KNR. There was no significant difference of pH levels between the soils. For the distribution of soil aggregates, large macroaggregates (>5 and 5–2 mm) dominated the KPF and KNR, while microaggregates (<0.25 mm) dominated the KMS; no > 5 mm aggregates were found in the KMS.
Composition of bacterial communities at phylum/subphylum, order and family levels
All the 600 tested clones were divided into 12 phylogenetic phyla (10, 10 and 7 phyla in KMS, KNR and KPF, respectively) including one unclassified bacteria group (Fig. 1). With 44.8% of the total clones, Proteobacteria represented the dominant phylum in each soil. With 16.5–32.0 and 8.0–12.5% of the clones in each soil, the second and third most abundant phyla in all three soils were Acidobacteria and Planctomycetes. Approximately 14.5% of all the clones were related to other bacterial phyla, including Verrucomicrobia, Actinobacteria, Bacteroidetes, Gemmatimonadetes, Nitrospira, Firmicutes, Chloroflexi and Cyanobacteria. Approximately 3.3% of the total clones remained unclassified.
Although Proteobacteria was the most abundant phylum in the three soils (covering 40.5, 36.0 and 58.0% for KMS, KNR and KPF, respectively), the distribution of subphyla was different (Fig. 1). δ-Proteobacteria (17.0%) was the dominant subphylum for KMS, followed by α-Proteobacteria (10.0%), while in KNR and KPF, the dominant subphylum was α-Proteobacteria (23.0 and 33.5%, respectively), followed by δ-Proteobacteria (10.5 and 15.5%, respectively). In total, 9.0 and 8.5% of the clones belonged to β-Proteobacteria subphylum in KMS and KPF, respectively, while 1.5% of the clones in KNR were clustered with this subphylum. Only 0.5–4.5% of the clones in each soil were grouped into γ-Proteobacteria. In addition, Verrucomicrobia comprised 11.5% of clones in KNR but were absent or sparse in KPF and KMS. Likewise, Chloroflexi (2.5%) and Cyanobacteria (1.0%) were found in KNR but not in KMS or KPF. Conversely, 1.0 and 1.5% of the clones from Firmicutes were found in KMS and KPF, respectively, while no clones of this type were found in KNR. Clones from Nitrospira were not detected in KNR and KPF, and clones from Bacteroidetes were not detected in KPF. Thus, the phyla with rare clones showed unbalanced distributions in this Karst area (Fig. 1).
For all of the clones, 79.7 and 67.5% could be identified to order and family, respectively (data not shown). Almost all of the Acidobacteria and Planctomycetes were further classified as Acidobacteriales (Acidobacteriaceae) and Planctomycetales (Planctomycetaceae), respectively; these were the abundant orders and families in all the three soils (Table 2). Interestingly, as the most abundant order in KPF (64 clones), the proportion of Rhizobiales belonging to α-Proteobacteria was approximately three to four times higher than that observed in KNR and KMS. Further classification showed that about half of the Rhizobiales were categorized as Hyphomicrobiaceae in the three soils. Moreover, 10.5% of the clones in KMS were identified as Polyangiaceae.
Bacterial diversity and comparison of clone libraries
In sum, 216 OTUs were screened in all the three soils. Comparing the RFLP patterns, the richness of OTUs among the soils varied from 66 to 80, which was the highest in KPF (Table 1). The Shannon-Wiener diversity index showed that KPF had the highest rating among the three clone libraries and there was no difference between KMS and KNR (Table 1). Coverage percentage ranged from 82.0 to 93.0% (Table 1), and the rarefaction curves for all clone libraries appeared to approach a plateau (Fig. 2). These results suggest that 200 clones were basically sufficient to estimate the bacterial diversity within one library in this study. Results of the ∫-LIBSHUFF program showed that all three bacterial communities were significantly different from each other (p = 0.001, data not shown).
Rarefaction curves of the 16S rDNA clone libraries. For sample abbreviations, see Fig. 1
Phylogenetic analysis of the dominant bacterial phylum—Proteobacteria
A phylogenetic tree was constructed using all represented sequences from the dominant bacterial phylum Proteobacteria, including 30, 18 and 39 OTUs from KMS, KNR and KPF, respectively (Fig. 3). All of the sequences were grouped into 11 clusters by combining the taxonomic classification of each sequence with the feature of branches in the dendrogram. Most of the OTUs were grouped into clusters I, VIII, IX and X, accounting for 65.1% of the total proteobacterial clones. In cluster I, the KNR clones accounted for 36.1% of the total whereas the proportions were 13.6 and 19.0% in KMS and KPF, respectively. Clusters VIII and X were dominated by proteobacterial clones from KMS, and cluster IX was dominated by KNR. The proteobacterial clones from KPF were widely distributed among all 11 clusters. Although clusters V–VII and XI contained only 16.0% of the total proteobacterial clones, their distribution in each soil was uneven. For instance, cluster V was only found in KPF. Furthermore, no clones originating from KMS grouped in cluster VI, and no clones retrieved from KNR were from clusters VII or XI.
Phylogenetic tree of the four subphyla of Proteobacteria based on the comparative analysis of 16S rDNA sequences from the three soils. Bootstrap values (n = 1,000 replicates) of ≥ 50% are reported as percentages. The scale bar indicates 0.05 inferred nucleotide substitutions per site. Methanococcus fervens (AF056938) was used as an outgroup. For sample abbreviations, see Fig. 1
Discussion
Responses of bacterial community composition and diversity to cultivation
The composition of soil bacterial communities were significantly altered by cultivation disturbance (soils from cropland and revegetated land) (Table 2, Figs. 1, 3). One of the great bacterial community discrepancies between the disturbed soils and the primeval forest soil appeared in the distributions of Proteobacteria and Acidobacteria. Compared with the primeval forest soil, the proportions of Proteobacteria were decreased by 30.2 and 37.9%, and Acidobacteria proportions were increased by 93.9 and 87.9% in the cropland and the revegetated land, respectively. These differences are primarily accounted for from the significant decrease in macroaggregates with cultivation (Table 1), because a high diversity of bacteria belonging to the Holophaga/Acidobacterium division presented in small particles while α-Proteobacteria dominated large particles (Sessitsch et al. 2001). Furthermore, as an extremely important factor, the discrepancies of soil nutrients may result in the predominance of Proteobacteria (especially for α-Proteobacteria) in the primeval forest soil but both Proteobacteria and Acidobacteria in the disturbed soil (Table 1, Fig. 1). It was because soil with a high content of readily available nutrients showed positive selection for α-Proteobacteria (named as r-selection), while soil with low-available nutrients or with high level of recalcitrant substrates contained more Acidobacteria (named as k-selection) (Smit et al. 2001).
The other significant discrepancy was the remarkably high proportion of the order Rhizobiales in the primeval forest soil, which plays an important role in N2-fixing and maintaining/improving soil fertility (O’Hara 2001). This large population likely occurred for two reasons. Firstly, abundant N2-fixing leguminous plants were found in the primeval forest soil, including Zenia insignis Chun and Bauhinia championii. Secondly, nitrogen fertilizer decreased the abundance of N2-fixing bacteria in the cropland soil (Kolb and Martin 1988). The conversion of the Karst forest to a cropping system was accompanied by the disappearance of clusters V and VI, which were related to Rhizobiales, while cluster VI was reproducing in the process of natural vegetation recovery (Fig. 3). Therefore, combining inoculation of native rhizobias from the primeval forest soil or application of N2-fixing microbial fertilizer with indigenous N2-fixing plants can be advocated for increasing the speed of ecological restoration of disturbed Karst ecosystems (Thrall et al. 2005). A similar suggestion has been proposed to recover desertified Mediterranean ecosystems (Herrera et al. 1993).
The bacterial diversity was lower in disturbed soils than in the primeval forest soil, which was in accordance with a report that bacterial diversity decreased with the intensity of tillage in sandy Norwegian soils (Øvreås and Torsvik 1998). During the conversion process from forest to cropland, soil nutrients (Table 1) and the diversity of flora and fauna were significantly decreased, which may influence microbial diversity (He et al. 2008; Ren 2005; Torsvik and Øvreås 2002). Moreover, the disproportionate distribution of OTUs affected bacterial diversity because the OTUs represented by only one clone were lower in the soil bacterial communities of cropland (30.0% of OTUs) and revegetated land (21.2%) when compared to the primeval forest (45.0%) (Table 3).
The after-effects of cultivation—similarities and differences of the bacterial communities between cropland and naturally revegetated land
In the present study, the general similarity of the soil bacterial communities and diversity between the cropland and naturally revegetated land indicated that the restoration of soil bacterial community is a very slow process. In part, this has been determined by the fact that the basic soil properties, one of the key factors determining the microbial diversity, did not change significantly after 20 years of vegetation recovery (Table 1) owing to the earlier farming practice (Knops and Tilman 2000). Compared to the non-Karst area, more time is likely required with a thin soil layer and a slow rate of soil formation to restore the soil bacterial community in the Karst area. Similar observations have been reported in sandy loam soil by Upchurch et al. (2008).
The distribution of four Proteobacteria subphyla between the revegetated land and the primeval forest soil was very similar. For example, the distribution relationships among the subphyla were α- > δ- > β- > γ-Proteobacteria for the revegetated land and the primeval forest soil, but δ- > α- > β- > γ-Proteobacteria for the cropland (Fig. 1). Moreover, clusters VII and XI, belonging to γ-Proteobacteria and δ-Proteobacteria, respectively, might not adapt to the vegetation recovery because no sequences grouped into these two clusters in the revegetated land, which itself became more similar to the primeval forest soil (Fig. 3). This indicated that vegetation recovery had positive effects on the subphyla of the dominant soil bacterial phylum (Proteobacteria), although there was limited effectiveness for restoration of the entire soil bacterial community.
It would be interesting to find that the number of bacterial phyla is not consistent with the bacterial diversity value in the tested soils. Similar relationships occurred in other agricultural soils (Roesch et al. 2007). Determining whether these results are a random phenomenon or a meaningful evolutionary mechanism for microorganisms is warranted.
In conclusion, this study provides the first evidence that the composition and diversity of soil bacterial communities has a complex response to cultivation disturbance in Karst ecosystems. Conservation of the primeval Karst forest is particularly important because eliminating the after-effects of cultivation on soil bacterial communities at the phylum level and physicochemical parameters is a slow process. Simultaneously, combining inoculation of N2-fixing microorganisms with indigenous N2-fixing plants may aid in restoring ecosystems after cultivation disturbance in the Karst region.
References
Badiane NNY, Chotte JL, Pate E, Masse D, Rouland C (2001) Use of soil enzyme activities to monitor soil quality in natural and improved fallows in semi-arid tropical regions. Appl Soil Ecol 18:229–238
Bakermans C, Madsen EL (2002) Diversity of 16S rDNA and naphthalene dioxygenase genes from coal-tar-waste-contaminated aquifer wasters. Microb Ecol 44:95–106
Bremmer JM (1965) Total nitrogen. In: Black CA et al (eds) Methods of soil analysis, 364, vol 2. American Society of Agronomy, Madison, pp 1149–1178
Chen Z, Luo X, Hu R, Wu M, Wu J, Wei W (2010) Impact of long-term fertilization on the composition of denitrifier communities based on nitrite reductase analyses in a paddy soil. Microb Ecol 60:850–861
Chow ML, Radomski CC, McDermott JM, Davies J, Axelrood PE (2002) Molecular characterization of bacterial diversity in Lodgepole pine (Pinus contorta) rhizosphere soils from British Columbia forest soils differing in disturbance and geographic source. FEMS Microbiol Ecol 42:347–357
DeGrood SH, Claassen VP, Scow KM (2005) Microbial community composition on native and drastically disturbed serpentine soils. Soil Biol Biochem 37:1427–1435
DeSantis TZ, Brodie EL, Moberg JP, Zubieta IX, Piceno YM, Andersen GL (2007) High-density universal 16S rRNA microarray analysis reveals broader diversity than typical clone library when sampling the environment. Microb Ecol 53:371–383
He XY, Wang KL, Zhang W, Chen ZH, Zhu YG, Chen HS (2008) Positive correlation between soil bacterial metabolic and plant species diversity and bacterial and fungal diversity in a vegetation succession on karst. Plant Soil 307:123–134
Herrera MA, Salamanca CP, Barea JM (1993) Inoculation of woody legumes with selected arbuscular mycorrhizal fungi and rhizobia to recover desertified Mediterranean ecosystems. Appl Environ Microbiol 59:129–133
Holland S (2004) Analytic rarefaction. http://www.uga.edu/~strata/software/
Izumi S, Aranishi F, Wakabayashi H (2003) Genotyping of Flavobacterium psychrophilum using PCR-RFLP analysis. Dis Aquat Organ 56:207–214
Kemper WD, Rosenau RC (1986) Aggregate stability and size distribution. In: Klute A (ed) Methods of soil analysis, Part 1. America Society of Agronomy, Monograph, WI, pp 425–442
Knops JMH, Tilman D (2000) Dynamics of soil nitrogen and carbon accumulation for 61 years after agricultural abandonment. Ecology 81:88–98
Kolb W, Martin P (1988) Influence of nitrogen on the number of N2-fixing and total bacteria in the rhizosphere. Soil Biol Biochem 20:221–225
Li YB, Wang SJ, Rong L (2004) Prospect of the study on rock desertification and its restoration in southwest Karst mountains. Chinese J Ecol 23:84–88
Mishra A, Nautiyal CS (2009) Functional diversity of the microbial community in the rhizosphere of chickpea grown in diesel fuel-spiked soil amended with Trichoderma ressei using sole-carbon-source utilization profiles. World J Microbiol Biotechnol 25:1175–1180
Mullins TD, Britschgi TB, Krest RL, Giovannoni SJ (1995) Genetic comparisons reveal the same unknown bacterial lineages in Atlantic and Pacific bacterioplankton communities. Limnol Oceanogr 40:148–158
Mummey DL, Stahl PD, Buyer JS (2002) Microbial biomarkers as an indicator of ecosystem recovery following surface mine reclamation. Appl Soil Ecol 21:251–259
O’Hara GW (2001) Nutritional constraints on root nodule bacteria affecting symbiotic nitrogen fixation: a review. Aust J Exp Agr 41:417–433
Øvreås L, Torsvik V (1998) Microbial diversity and community structure in two different agricultural soil communities. Microb Ecol 36:303–315
Ren H (2005) A review on the studies of desertification process and restoration mechanism of Karst rocky ecosystem. Trop Geogr 25:195–200
Roesch LFW, Fulthorpe RR, Riva A, Casella G, Hadwin AKM, Kent AD, Daroub SH, Camargo FAO, Farmerie WG, Triplett EW (2007) Pyrosequencing enumerates and contrasts soil microbial diversity. ISME J 1:283–290
Sessitsch A, Weilharter A, Gerzabek MH, Kirchmann H, Kandeler E (2001) Microbial population structures in soil particle size fractions of a long-term fertilizer field experiment. Appl Environ Microbiol 67:4215–4224
Shannon CE, Weaver W (1949) The mathematical theory of communication. University of Illinois Press, Urbana
Singleton DR, Furlong MA, Rathbun SL, Whitman WB (2001) Quantitative comparisons of 16S rRNA gene sequence libraries from environmental samples. Appl Environ Microbiol 67:4374–4376
Smit E, Leeflang P, Gommans S, Broek J, Mil S, Wernars K (2001) Diversity and seasonal fluctuations of the dominant members of the bacterial soil community in a wheat field as determined by cultivation and molecular methods. Appl Environ Microbiol 67:2284–2291
Smith BFL, Bain DC (1982) A sodium hydroxide fusion method for the determination of total phosphate in soils. Commu Soil Sci Plan 13:185–190
Tamura K, Dudley J, Nei M, Kumar S (2007) MEGA4: molecular evolutionary genetics analysis (MEGA) software version 4.0. Mol Biol Evol 24:1596–1599
Thrall PH, Millsom DA, Jeavons AC, Waayers M, Harvey GR, Bagnall DJ, Brockwel J (2005) Seed inoculation with effective root-nodule bacteria enhances revegetation success. J Appl Ecol 42:740–751
Torsvik V, Øvreås L (2002) Microbial diversity and function in soil: from genes to ecosystems. Curr Opin Microbiol 5:240–245
Upchurch R, Chiu CY, Everett K, Dyszynski G, Coleman DC, Whitman WB (2008) Differences in the composition and diversity of bacterial communities from agricultural and forest soils. Soil Biol Biochem 40:1294–1305
Wang SJ, Liu QM, Zhang DF (2004a) Karst rocky desertification in southwestern China: geomorphology, landuse, impact and rehabilitation. Land Degrad Dev 15:115–121
Wang SJ, Li RL, Sun CX, Zhang DF, Li FQ, Zhou DQ, Xiong KN, Zhou ZF (2004b) How types of carbonate rock assemblages constrain the distribution of Karst rocky desertified land in Guizhou Province, PR China: phenomena and mechanisms. Land Degrad Dev 15:123–131
Weisburg WG, Barns SM, Pelletier DA, Lane DJ (1991) 16S ribosomal DNA amplification for phylogenetic study. J Bacteriol 173:697–703
Xiao H, Weng Q (2007) The impact of land use and land cover changes on land surface temperature in a Karst area of China. J Environ Manage 85:245–257
Yao CH, Jiang ZC, Yuan DX (2001) Vegetation effects on Karst physiognomy in southwest China. Acta Geoscientia Sinica 22:159–164
Yuan DX, Chai GH (1988) The science of Karst environment. Chongqing Press, Chongqing
Zhang P, Li L, Pan G, Ren J (2006a) Soil quality changes in land degradation as indicated by soil chemical, biochemical and microbiological properties in a Karst area of southwest Guizhou, China. Environ Geol 51:609–619
Zhang W, Chen HS, Wang KL, Su YR, Zhang JG, Yi AJ (2006b) The heterogeneity of soil nutrients and their influencing factors in peak-cluster depression areas of Karst region. Scientia Agricultura Sinica 39:1828–1835
Zhou J, Xia B, Huang H, Treves DS, Hauser LJ, Mural RJ, Palumbo AV, Tiedje JM (2003) Bacterial phylogenetic diversity and a novel candidate division of two humid region, sandy surface soils. Soil Biol Biochem 35:915–924
Acknowledgments
This study was jointly supported by the Knowledge Innovation Program of the Chinese Academy of Sciences (KSCX2-YW-436 and KZCX2-XB2-08-01), Strategic Priority Research Program of the Chinese Academy of Sciences (XDA05070403) and the National Natural Science Foundation of China (40671104 and 30970538).
Conflict of interest
The authors declare that they have no conflict of interest.
Author information
Authors and Affiliations
Corresponding author
Rights and permissions
About this article
Cite this article
Chen, X., Su, Y., He, X. et al. Soil bacterial community composition and diversity respond to cultivation in Karst ecosystems. World J Microbiol Biotechnol 28, 205–213 (2012). https://doi.org/10.1007/s11274-011-0809-0
Received:
Accepted:
Published:
Issue Date:
DOI: https://doi.org/10.1007/s11274-011-0809-0