Abstract
Recently, there has been increasing concern about perfluorinated compounds, especially perfluorooctane sulfonate (PFOS) and perfluorooctanoic acid (PFOA) due to their biotic and abiotic persistence and chronic toxicity. To characterize the spatial distribution and seasonal variation of PFOS and PFOA in the aquatic and oceanic environment of Singapore, more than 100 water samples from reservoirs, rivers/canals, coastal waters, and treated effluents of wastewater treatment plants (WWTPs) were collected and analyzed in this study. Solid-phase extraction followed by high-performance liquid chromatography (HPLC) coupled with tandem MS (HPLC/MS/MS) was applied to quantitatively identify PFOS and PFOA. PFOS concentrations in surface waters, wastewaters and coastal waters were in the range of 2.2–87.3 ng/L, 5.8–532 ng/L, and 1.9–8.9 ng/L, respectively, while those of PFOA were 5.7–91.5 ng/L, 7.9–1,060 ng/L, 2.4–17.8 ng/L, respectively. Compared with surface waters and wastewaters, coastal waters had lower concentrations of PFOS and PFOA. Highest concentration of PFOA (532 ng/L) and PFOA (1,060 ng/L) were observed in treated effluents of two WWTPs. Our results suggest that coastal waters in the western area of Singapore are more heavily contaminated than those in the middle and eastern areas. The release of effluents from WWTPs is an important pathway by which perfluorinated compounds enter the oceanic environment. Between dry season and wet season, significant seasonal differences (p = 0.025) were observed in surface waters for PFOS only, while no discernable seasonal differences were found for both PFOS and PFOA in coastal waters and wastewaters.
Similar content being viewed by others
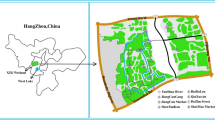
Explore related subjects
Discover the latest articles, news and stories from top researchers in related subjects.Avoid common mistakes on your manuscript.
1 Introduction
Perfluorinated compounds (PFCs) have been manufactured and used globally for over 50 years. These compounds, particularly perfluorooctane sulfonate (PFOS; C8F17SO −3 ) and perfluorooctanoic acid (PFOA; C7F15COO−), have emerged as a new class of environmentally persistent pollutants, which have been widely used in different applications, such as surfactants and surface protectors in carpets, leather, paper, food containers, fabric, upholstery, fire-fighting foams, floor polishes, and shampoos (Kissa 2001). PFOS and PFOA are known to cause acute and subchronic toxicity effects on animals in laboratory studies (Haughom and Spydevold 1992; Seacat et al. 2003). In response to these concerns, 3 M, a principal manufacturer, decided to phase out its production of PFOA, PFOS, and PFOS-related products in May 2000.
PFOS and PFOA, regarded as the terminal breakdown end-products of PFCs, have been detected in a wide array of environmental matrices including biota (Giesy and Kannan 2001; Kannan et al. 2002; Löfstrand et al. 2008), water (Taniyasu et al. 2003; Saito et al. 2003; Schultz et al. 2004; So et al. 2004; Sinclair et al. 2006), sediment and sludge (Higgins et al. 2005; Sinclair and Kannan 2006). They are ubiquitous in the environment because of their high persistence, resulting from their exceptionally thermal and chemical stability. They are stable to acids, bases, oxidants, and reductants and are generally not believed to undergo metabolic or other degradation in the environment (Prevedouros et al. 2006).
Surveys have been conducted to monitor the extent of PFOS and PFOA contamination in surface waters (Hansen et al. 2002; Boulanger et al. 2004; Loos et al. 2008), wastewaters (Boulanger et al. 2005; Sinclair and Kannan 2006; Loganathan et al. 2007; Becker et al. 2008; Yu et al. 2009), drinking waters (Harada et al. 2003; Takagi et al. 2008; Mak et al. 2009), groundwaters (Schultz et al. 2004) and coastal waters (So et al. 2004; Saito et al. 2003; Yamashita et al. 2005). In freshwaters, PFOS and PFOA are typically present at low ng/L levels up to several tens of ng/L. Elevated concentrations of PFOS (114 ± 19 ng/L) and PFOA (394 ± 128 ng/L) were detected downstream of the receiving water of the 3 M fluorochemical manufacturing facility at Decatur (3M Company 1999). In wastewaters, it was observed that PFOS and PFOA occurred in effluents of WWTPs (Sinclair and Kannan 2006; Loganathan et al. 2007; Schultz et al. 2006). In the multi-city study of the 3M Company, much higher concentrations of PFOS (48–4,980 ng/L) and PFOA (42–2,280 ng/L) were found in effluents of publicly owned treatment works (3M Company (2001) In coastal waters, low concentrations of PFOS and PFOA were observed in open ocean water samples from the Pacific and Atlantic Oceans, and from several Asian countries, while high concentrations of these compounds were found in offshore waters (Yamashita et al. 2005).
In order to investigate the distribution of PFOS and PFOA in different water matrices in Singapore, 138 water samples were collected from reservoirs, rivers/canals, wastewater treatment plants and coastal waters around the island over a year. The purpose of this study was to determine the magnitude and extent of PFCs' contamination and to provide an overview of the spatial distribution of PFOS and PFOA in the waters of Singapore. Moreover, surface water samples in the industrial districts, wastewater samples from all the six WWTPs in Singapore and coastal water samples were collected and analyzed in attempt to locate possible contamination sources within the island. In addition, seasonal variations between dry seasons and wet seasons were also studied. The results of this study would identify the sources and transportation pathways of PFCs in the aquatic and oceanic environment of Singapore.
2 Materials and Methods
2.1 Chemicals and Standards
Standards of perfluorooctane sulfonate potassium salt (PFOS, ≥98%), perfluorooctanoate acid (PFOA, 96%), methanol (99.8%) and ammonium acetate (97%) were purchased from Sigma-Adrich (Singapore). Oasis HLB (500 mg, 6 cm3) solid-phase extraction (SPE) cartridges were obtained from Waters (Milford, USA). Stock solutions were prepared in methanol to achieve a concentration of 1 mg/ml. From these stock solutions, working solutions were prepared by dilution with methanol. Stock solutions and working solutions were stored at −20ºC.
2.2 Sample Collection
Water samples from reservoirs, rivers, canals, effluents of WWTPs and coastal waters around the island were respectively collected in October 2006, December 2006, March 2007, September 2007 and December 2007. Due to the tropical climate in Singapore, November and December are the wet monsoon seasons, while other months are relatively dry seasons in Singapore (Ooi and Chia 1974). Monthly rainfall totals are 86, 788, 169, 197, and 413 mm for October 2006, December 2006, March 2007, September 2007, and December 2007, respectively (National Environment Agency 2008). For the sampling in December, it was performed during the second week while it was raining. During sampling, outside temperature ranged from 21°C to 32°C.
Western area of Singapore is a heavily industrial influenced area, while eastern and middle areas are predominantly commercial and light industrial areas. Some samples were collected from urban regions, while others were from industrial influenced districts (sampling sites illustrated in Fig. 1). Grab wastewater samples were collected from the secondary effluents of conventional activated sludge processes in the six WWTPs in Singapore. Characteristics of WWTPs are listed in Table 1. According to the composition of the influents, these WWTPs are categorized to domestic WWTPs and industrial WWTPs. Industrial WWTPs include W2 and W5, while the rest are domestic WWTPs. OW1, outfall of W2, are 1 Km away from the shore, by which effluent of W2 is partially discharged to the sea. Samples were collected and stored in high density polyethylene bottles. Sample bottles were kept on ice and brought back to the laboratory within 6 h of collection.
2.3 Sample Extraction
Immediately after the collection, water samples were filtrated by GF/B glass filter (Whatman, USA) and stored at −20°C until extraction. In most cases, samples were extracted within 24 h after collection. Extraction procedure used was similar to that described previously (So et al. 2004; Taniyasu et al. 2005) with minor modifications. Briefly, analytes were extracted using 500-mg hydrophilic-lipophilic balance (500 mg, 6 cm3 HLB) cartridges, which were sequentially preconditioned with 5 mL of methanol and 10 mL of Milli-Q water; 1 L filtrated water sample was loaded on cartridges at a fixed flowrate of 10 mL/min, after which the cartridges were rinsed with 5 mL of 40% methanol in Milli-Q water. Then the cartridges were completely dried by air on the manifold using the vacuum pump. Finally, the cartridges were eluted with 2 × 5 mL methanol into a polypropylene tube. The resulting extract was reduced to 1 mL under a gentle stream of pure nitrogen gas. Final extracts were filtrated by 0.2 μm nylon syringe filters to remove fine particles and then transferred to polypropylene vial for analysis.
2.4 Instrumental Analysis
High performance liquid chromatograph, composed of a HP1100 liquid chromatograph (Aligent Technologies, USA) interfaced with a triple quadrupole MS/MS (Applied Biosystems, USA) was applied to detect samples in the electrospray negative ionization mode. Separation of compounds was performed on a 150 × 2.1 mm (5 μm) Zobax Extend C18 column (Aligent Technologies, U.S.A). A 10-μL aliquot of the sample extract was injected into a guard column (XDB-C8, 2.1mmi.d.×12.5 mm, 5 μm; Agilent Technologies, U.S.A) connected sequentially to Zorbax Extend C18 column with 2 mM ammonium acetate aqueous solution (solvent A) and methanol (solvent B) as mobile phases, starting at 3% of solvent B. The flow rate was set at 300 μL/min. The gradient was held until 0.50 min, increased to 95% B until 6.00 min, held until 8.50 min, reverted to original conditions at 8.51 min and was held 3% B until 12.00 min. The column temperature was kept constant at 30°C.
MS/MS was operated under multiple reaction monitoring mode and mass transition mode was m/z 499.1 98.9 for PFOS and m/z 413.1 369.1 for PFOA. The mass spectrometer was operated using the TurboIonspray™ source in the negative mode. The ionization source-specific parameters were listed below: curtain gas, 30 psi; collision gas, 6 psi; ionspray voltage, −4,500 V; temperature of the turbo heater gas, 450°C; nebuliser gas (GS1), 40 psi.; and turbo gas (GS2), 90 psi. Nitrogen was used as the curtain gas, nebuliser gas and turbo gas. Other analyte-dependent parameters were optimized for each compound. Methanol was run between the water samples to prevent carryover effect.
Quantification was based on the response of the external standards. The calibration curve containing 0.25, 0.5, 1, 5, 10, 50, 100, and 250 ng/mL standard was used with correlation coefficients (r 2) exceeding 0.995 for PFOS and PFOA. Calibration curve was prepared routinely, to check for linearity. The curve covered a range equivalent to the concentration of the analytes after 1,000 mL water sample was concentrated to 1.0 mL extract (approximately 1,000-fold concentration). Sample extracts with concentrations exceeding the range of calibration curve were appropriately diluted by methanol and reinjected again.
2.5 Statistical Analysis
Statistical software Minitab (Minitab Inc, USA) was used to calculate the correlation between PFOS and PFOA as well as the correlations of concentrations between dry season and wet season. Statistical significance was accepted at p < 0.05.
3 Results and Discussion
3.1 Quality Assurance and Control
Because of the presence of the fluoropolymer in some laborotary equiments, precautions were taken to minimize the possible contamination during the analysis (Yamashita et al. 2004). For example, teflon bottles, Teflon-lined caps, and any suspected fluoropolymer materials were not utilized throughout the analysis.
In order to ensure the quality of the sampling and extraction, Milli-Q water was used as field blanks and procedural blanks to evaluate the possible contamination during the transportation and analysis for each batch of samples. They were extracted following the same procedure as the real water samples. For each field blank and procedural blank analyzed, PFOS and PFOA were below the detection limit, indicating that no discernable contamination occurred during sampling and analysis.
The analytical characteristics of the method, such as reproducibility and limits of quantification, were investigated to evaluate the efficiency of the method. Procedural recovery was evaluated by spiking mixture of external standards (100 ng/mL, 100 μL) to Milli-Q water. Procedural recoveries for PFOS and PFOA were in the range 95–103% (mean, 98.4%, n = 3) and 90–98% (mean: 93.8%, n = 3), respectively. The SPE extraction of spiked Milli-Q water were applied to determine the limit of detection (LOD) and limit of quantification (LOQ). LOD, defined as the concentration that yielded an S/N ratio of ≥ 3, was 0.1 ng/L for PFOS and 0.5 ng/L for PFOA. LOQ, defined as the concentration that yielded a S/N ratio of ≥10, was 0.25 ng/L for PFOS and 1.25 ng/L for PFOA. Furthermore, spiked additions were applied to identify the matrix suppression on the ion signals for each batch of samples based on the standard addition method. They were prepared by spiking mixtures of external standards (100 ng/ml, 100 μL) into the SPE extracts of the effluents obtained from W4. Recoveries of spiked additions for PFOS and PFOA were in the range of 80–93% (mean: 87.8%, n = 5) and 78–90% (mean, 83.9%, n = 5), respectively. Sufficient recoveries achieved for procedural blanks and spiked additions demonstrated the reliability and efficiency of the analysis method.
3.2 PFOS/PFOA Concentrations in Surface Water
Concentrations of PFOS and PFOA in surface waters, wastewaters and coastal waters from five batches of sampling campaigns are summarized in Figs. 2, 3, and 4, which show the spatial distribution of these two compounds in the western, middle, and eastern areas of Singapore. Overall, PFOS and PFOA concentrations in all samples were in the ranges of 2.2-532 ng/L and 2.4–1,060 ng/L, respectively.
The concentrations of PFOS and PFOA in surface waters ranged from 2.2 to87.3 ng/L and from 5.7 to 91.5 ng/L, respectively, which are comparable to but slightly higher than those observed in the Great Lakes (USA; PFOS, 21–70 ng/L; PFOA, 27–50 ng/L) (Boulanger et al. 2004). Lower PFOS (2.9–30 ng/L) and PFOA (18–34 ng/L) concentrations were reported in the Lake Ontario, USA (Sinclair et al. 2006), which suggests slightly higher PFCs contamination level in the surface waters of Singapore. Comparable PFOS concentration range was also observed in Guangzhou (0.9–99 ng/L) (So et al. 2007), one of most industrialized areas in China. The highest concentration of PFOS (87.3 ng/L) in surface waters was detected at S5, eastern area subjected to light industrial influence. This indicates potential PFCs contamination sources nearby. In comparison to other studies, however, the highest PFOS concentration was approximately half of that reported in Tama river in Japan (157 ng/L) (Saito et al. 2003) and in downstream of discharge of 3 M fluorochemical manufacturing facility (144 ng/L) (Hansen et al. 2002). The concentration of PFOS detected at S5 was also about seven times lower than the highest concentration (651 ng/L) measured in Lake Shihwa (south Korea) (Rostkowski et al. 2006) and 11 times lower than that (1090 ng/L) of Lake Onondaga (New York, USA) (Sinclair et al. 2006), both of which are heavily influenced by the industrial effluents.
Compared with this study, lower PFOA concentration ranges were observed in Guangzhou area (0.85–13 ng/L) So et al. (2007)) and Pearl River Delta (0.24–16 ng/L) (So et al. 2004), both of which are heavily associated with industrial and urban activities. In particular, the highest PFOA concentration (91.5 ng/L) in surface water was observed at S7, which was collected downstream of a canal that flows along the edge of an airport. It suggests that the airport may be a potential PFCs contamination source. In contrast, the PFOA level in this study was approximately 2 and 3 times lower than those observed in Tokyo Bay in Japan (Yamashita et al. 2004) and Yangtze River, the largest river in China (So et al. 2007).
The total PFCs (i.e., PFOS and PFOA) concentrations from five sampling campaigns for all surface waters are summarized in Fig. 5. It can be seen that S9, located at the western area, had the highest total PFCs concentration, which suggests the presence of potential PFCs contamination source in the surrounding area. However, S7 had the next highest total PFCs concentration among all surface waters even though its location is in the eastern area (urban region). This may be due to the leakage of perfluorinated surfactants, such as aqueous fire-fighting foams, gasoline, oil and lubricants (Moody and Field 2000), from the adjacent airport. Similarly, the highest total PFCs concentration in reservoir waters was detected in R8, which is the downstream of the S9. Furthermore, R2, R3, and R4, which are in the nature reserve area (middle area), had lower concentrations compared with other reservoirs, which are in the industrial or commercial influenced area. In contrast, the higher concentrations were observed in R7, R8, and R9 which are in the industrial area (western area). It suggests that factories, such as petrochemical, paints, coatings and surfactants manufacturing plants in the western region, may be the potential PFCs sources, thus causing this area to be the most contaminated area in Singapore. It is however worthy of pointing out that, even though the highest concentrations of PFCs in reservoirs were observed in R8 and R9, their PFOS and PFOA concentrations were a few order of magnitude lower than the lifetime drinking water health advisory for PFOS (1,000 ng/L) (Hansen et al. 2002) and PFOA (150,000 ng/L) (Poulsen et al. 2005). Overall, the concentrations of PFOS and PFOA in reservoirs (mean: 17.6 ng/L for PFOS, 24.9 ng/L for PFOA) were comparable to those of river water samples (mean, 20.2 ng/L for PFOS, 28.4 ng/L for PFOA). Reservoir waters however, had relatively lower variation in concentrations for both PFOS and PFOA in comparison with river/canal waters.
PFOA percentage in the total PFCs concentration varied from 29.5–74.6% in surface waters, indicating different contamination sources of perfluorinated compounds (Fig. 5). PFOA contribution to the PFCs, which were greater than 50% in all surface water samples except for R9 and S5, indicated that PFOA was predominant in most of surface waters. Predominance of PFOA over other PFCs was also observed in the water samples collected along the Yangtze River (So et al. 2007). The lowest PFOA percentage observed in S5 suggests that there may be significant PFOS contamination source upstream or in the surrounding area, which greatly increases PFOS contribution to the PFCs. In addition, even though the location of R9 is geographically close to R8, the lower PFOA percentage of R9 suggests that different contamination sources occur in its catchment area or its tributaries.
3.3 PFOS/PFOA Concentrations in Wastewater
The observed PFOS concentrations were in the range of 5.8–532 ng/L in the effluents of WWTPs, which are much higher than those of ten municipal WWTPs (1.1–130 ng/L) in USA (Schultz et al. 2006). The highest PFOS concentration (532 ng/L) was detected in the effluent of W1, one of domestic WWTPs. This is comparable to those measured in a WWTP in Cleveland with no known fluorochemical sources (417–454 ng/L) (3M Company (2001). With the exception of W1, PFOS concentrations in effluents of domestic WWTPs, in the range of 5.8–35.3 ng/L, were lower than those of 10 municipal WWTPs in USA (Schultz et al. 2006). The higher total PFOS concentration in W1 as compared to other domestic WWTPs suggested the occurrence of significant PFOS contamination sources in the served area (Fig. 6). The next highest PFOS concentration (432 ng/L) was observed in the effluent of W5, which receives 60% industrial wastewater.
PFOA concentrations in the effluents of WWTPs were observed in the range of 7.9–1,060 ng/L. PFOA concentrations in industrial WWTPs (22.6–1,060 ng/L) were much higher than those from domestic WWTPs (7.9–157 ng/L). In the effluents of domestic WWTPs, PFOA concentrations are higher than those of ten WWTPs in USA (PFOA: 2.5–97 ng/L) (Schultz et al. 2006) and comparable to two WWTPs in Kentucky and Georgia (PFOA: 6.7–183 ng/L) (Loganathan et al. 2007). In addition, the highest PFOA concentration (1,060 ng/L) was also observed in the effluent of W5. This is significantly higher than those from Cleveland (665–674 ng/L) but about two times lower than those from Decatur (2,140–2,420 ng/L) with influence from fluorochemical manufacturing (3M Company (2001). The industries in the service area of W5 include petrochemical intermediates, petroleum refining, paints, coatings and surfactants manufacturing. Our results suggest that discharges from these fluorochemical related plants may contain a large amount of PFOS and PFOA, thus leading to high PFCs concentrations in the effluents of WWTPs. It is evident that discharge of effluents from WWTPs is an important pathway through which PFCs enter the environment.
The highest total PFCs concentration were observed in the effluent of W5, indicating industrial influent significantly affect the PFCs' concentrations in the effluent of WWTPs (Fig. 6). However, much higher total PFCs concentration detected in W1, one of the domestic WWTPs, than that of W2 (industrial WWTP). It suggests that the composition of the industrial influent entering W2 may be different and may not be closely related to PFCs' contamination. Moreover, PFOA was predominant over PFOS in the WWTPs' effluents except for W1. The lowest PFOA percentage observed (22.5%) in the effluent of W1 indicates that the potential sources of PFCs in its service area are different from those of other WWTPs. Further research is needed to identify those potential contamination sources.
3.4 PFOS/PFOA Concentrations in Coastal Water
PFOS and PFOA were detected in all coastal waters and were in the range of 1.9–8.9 and 2.4–17.8 ng/L, respectively. This is relatively higher than those detected in Hongkong coastal waters (PFOS: 0.09–3.1 ng/L, PFOA: 0.7–5.5) (So et al. 2004), but much lower than those detected in Tokyo Bay (PFOS, 0.3–57.7 ng/L; PFOA, 1.8–192 ng/L) (Yamashita et al. 2005). The highest PFOS and PFOA concentration as well as total PFCs concentration in coastal waters were detected at C4 (Fig. 7), which is near the causeway connecting the Singapore Island and the Malay Peninsula across the Johor Strait. It suggests that Johor Straits is more heavily contaminated than the southern and eastern coastal waters. Industries in the northwestern area may be the significant contamination sources for Johor Straits since W2 discharges its effluent nearby. The next highest total concentration occurred in C2, which was collected from the confluence of rivers S2, S3 and S4 flowing through the commercial areas. PFOS and PFOA concentrations in S2, S3 and S4 were in the range of 4.8–28.3 and 7.4–36.6 ng/L, respectively, and were expected to be the major contributors for high level of PFOS and PFOA to C2. The lowest total PFCs concentration was observed at the location of C1, suggesting that eastern coastal waters is cleaner than other coastal waters in terms of PFCs contamination. It was observed that PFOA was predominant over PFOS in all coastal waters, which was indicated by the PFOA percentage. Such an interesting observation was also reported by other studies (So et al. 2004; Yamashita et al. 2005). The highest PFOA percentage (69.2%) was observed at the site of C2, suggesting that commercial activity may lead to high PFOA composition in waters.
As the OW1 is close to the location of C4, the correlation between coastal water C4 and wastewater W2 was investigated (Fig. 8). For both PFOS and PFOA, water samples at C4 could be significantly correlated to effluent of W2. Furthermore, PFOA percentage in C4 (60.6%) was in agreement with that of W2 (65%). This suggests that discharge of WWTPs may be the major source of perfluorinated compounds in the environment.
3.5 Seasonal Variation in the Concentrations of PFOS/PFOA
For surface waters, significant seasonal difference (p = 0.025) was observed for PFOS between dry seasons and wet seasons, while no significant difference (p = 0.616) was observed for PFOA. It seems that PFOS concentrations noticeably decreased during wet season, while there was no discernable decrease in PFOA concentrations in surface waters. The presence of PFCs in rainfall indicates rainfall significantly affect their concentrations in surface water (Prevedouros et al. 2006). PFOS have been observed at a low concentration (0.59 ng/L) in the precipitation, while significant higher PFOA concentrations were reported in rainwater. Kallenborn et al. (2004) reported that PFOA was the predominant PFCs measured in rainwater samples from Sweden and Finland with the greatest concentrations (11 and 17 ng/L, respectively). Scott et al. (2006) also reported relatively high PFOA concentrations (<0.1–89 ng/L) in the rainwater samples from USA and Canada. Based on the limited data, it seems that PFOS concentration in rainwater is lower than that of PFOA, which leads to their different seasonal variations in surface water. Furthermore, runoff could also be the potential PFCs sources during rainy weather. Rainfall may pick up and carry away PFCs pollutants (such as oil, fire-fighting foam) when it moves over and through the ground, which leads to the occurrence of nonpoint source pollution (NPS) of PFCs. Zushi et al. (2008) also observed that some PFCs concentrations in a river did not decrease with the increase of river flow rate during the rainy weather due to the nonpoint source pollution of PFCs. The predominance of PFOA over PFOS in most of surface waters indicated that PFOS is used less widely than PFOA in the island. Therefore, runoff contains lower amount of PFOS, which dilutes the surface waters. Unlike the surface waters, no discernable seasonal differences were found for both PFOS and PFOA in coastal waters and wastewaters. NPS of PFCs occurring during wet season may contribute to the indiscernible variations in PFCs concentrations from coastal waters and wastewaters. Moreover, it is possible that industrial activities lead to high concentration variations in the wastewaters, which override the seasonal differences between dry seasons and wet seasons. Since wastewaters are discharged into the coastal water, the same effect could subsequently apply to coastal waters. Similarly, no large-magnitude seasonal variations in concentrations of PFCs were found among spring, summer, fall and winter seasons in two municipal sewage treatment plants (Loganathan et al. 2007).
3.6 Correlation Between PFOS and PFOA Concentration
Correlations of PFOS and PFOA concentration in the surface waters, coastal waters, and effluents of WWTPs were examined. It was found that PFOS and PFOA in the coastal waters could be significantly correlated (r = 0.751, p = 0.001), while weak positive correlations were observed in surface waters (r = 0.444, p = 0.001) and wastewater (r = 0.531, p = 0.003). This suggests that the possibility of a common contamination source for these two compounds in coastal waters may be higher than those of surface waters and wastewaters. Such correlation between those two PFCs could be attributed to the production and application of related products as well as their subsequent release into the environment. Similarly, So et al. (2007) observed strong positive correlations between PFOS and PFOA in coastal waters collected from Hongkong and South China. In addition, Loganathan et al. (2007) found that PFOS were significantly correlated to PFOA in the wastewaters of one WWTP (r 2 = 0.772), while weak positive correlations were observed in those of another WWTP studied (r 2 = 0.084).
4 Conclusion
PFOS and PFOA were detectable in all 138 water samples from reservoirs, rivers/canals, coastal waters and treated effluents from WWTPs around the island. Ranges of PFOS concentrations in surface waters, wastewaters and coastal waters were 2.2–87.3, 5.8–532 and 1.9–8.9 ng/L, respectively, while those of PFOA concentrations were 5.7–91.5, 7.9–1,060, 2.4–17.8 ng/L, respectively. Overall, coastal waters had lower concentrations of PFOS and PFOA, compared with surface waters and wastewaters.
In surface waters, the highest total concentrations of PFOS and PFOA were observed in the western area because of the high levels of industrial activities in that area. This region was noted to be the most highly contaminated by PFCs. The next highest total concentration was observed at the location that is adjacent to the airport, indicating that leakages of perfluorinated surfactants, such as aqueous fire-fighting foams, gasoline, oil and lubricants (Moody and Field 2000) from the airport may be potential contamination sources.
In wastewaters, the highest total PFCs concentration and PFOA concentration (1,060 ng/L) were observed in W5, suggesting discharges of fluorochemical related factories in the service area of W5 may contain a large amount of PFOS and PFOA, thus resulting in high concentrations in the WWTPs effluents. The highest PFOS concentration (532 ng/L) was detected in the effluent of W1 treating mainly domestic and commercial wastewater. This indicates the presence of potential PFOS contamination sources in its service area. Compared with surface waters and coastal waters, much higher PFCs concentrations in wastewaters indicate that discharge of effluents of WWTPs is an important pathway by which PFCs enter the environment.
In coastal water, the high PFOS and PFOA concentrations at C4 suggest that Johor Straits is more heavily contaminated than the southern and eastern coastal waters. The high levels of industrial activities in the western area may be the significant contamination sources for Johor Straits. In addition, for both PFOS and PFOA water samples at C4 were significantly correlated with effluents of W2.
Between dry seasons and wet seasons, significant seasonal variation was observed in surface waters for PFOS only, while no discernable seasonal differences were found for both PFOS and PFOA in coastal waters and wastewaters. In addition, PFOS and PFOA were significantly correlated in the coastal waters, while weak positive correlations were observed in surface waters and wastewaters. It suggests that the possibility of a common contamination source for these two compounds in coastal waters is higher than those of surface waters and wastewaters.
References
3M Company (1999). Fluorochemical Use, Distribution and Release Overview; U.S. EPA Administrative Record AR226-0550; Washington, DC: U.S. Environmental Protection Agency.
3M Company (2001). Environmental Monitoring-Multi-City Study (Water, Sludge, Sediment, POTW Effluent and Landfill Leachate Samples); Docket AR-226-1030a; U.S. Environmental Protection Agency, Office of Pollution and Prevention and Toxic Substances: Washington, DC.
Becker, A. M., Gerstmann, S., & Frank, H. (2008). Perfluorooctane surfactants in waste waters, the major source of river pollution. Chemosphere, 72, 115–121.
Boulanger, B., Vargo, J., Schnoor, J. L., & Hornbuckle, K. C. (2004). Detection of perfluorooctane surfactants in Great Lakes water. Environmental Science and Technology, 38, 4064–4070.
Boulanger, B., Vargo, J. D., Schnoor, J. L., & Hornbuckle, K. C. (2005). Evaluation of perfluorooctane surfactants in a wastewater treatment system and in a commercial surface protection product. Environmental Science and Technology, 39, 5524–5530.
Giesy, J. P., & Kannan, K. (2001). Global distribution of perfluorooctane sulfonate in wildlife. Environmental Science and Technology, 35, 1339–1342.
Hansen, K. J., Johnson, H. O., Eldridge, J. S., Butenho, J. L., & Dick, L. A. (2002). Quantitative characterization of trace levels of PFOS and PFOA in the Tennessee River. Environmental Science and Technology, 36, 1681–1685.
Harada, K., Saito, N., Sasaki, K., Inoue, K., & Koizumi, A. (2003). Perfluorooctane sulfonate contamination of drinking water in the Tama River, Japan: Estimated effects on resident serum levels. Bulletin of Environmental Contamination and Toxicology, 17, 31–36.
Haughom, B., & Spydevold, O. (1992). The mechanism underlying the hypolipemic effect of perfluorooctanoic acid (PFOA), perfluorooctane sulphonic acid (PFOSA) and clofibric acid. Biochimica et Biophysica Acta, 1128, 65–72.
Higgins, C. P., Field, J. A., Criddle, C. S., & Luthy, R. G. (2005). Quantitative determination of perfluorochemicals in sediments and domestic sludge. Environmental Science and Technology, 39, 3946–3956.
Kallenborn, R., Berger, U., & Järnberg, U. (2004). Perfluorinated alkylated substances (PFAS) in the nordic environment (p. 107). Oslo: Norwegian Institute for Air Research.
Kannan, K., Newsted, J. L., Halbrook, R. S., & Giesy, J. P. (2002). Perfluorooctanesulfonate and related fluorinated hydrocarbons in mink and river otters from the United States. Environmental Science and Technology, 36, 2566–2571.
Kissa, E. (2001). Fluorinated surfactants and repellents (2nd ed.). New York: Marcel Dekker.
Löfstrand, K., Jörundsdóttir, H., Tomy, G., Svavarsson, J., Weihe, P., Nygård, T., et al. (2008). Spatial trends of polyfluorinated compounds in guillemot (Uria aalge) eggs from North-Western Europe. Chemosphere, 72, 1475–1480.
Loganathan, B. G., Sajwan, K. S., Sinclair, E., Kumar, K. S., & Kannan, K. (2007). Perfluoroalkyl sulfonates and perfluorocarboxylates in two wastewater treatment facilities in Kentucky and Georgia. Water Research, 41, 4611–4620.
Loos, R., Locoro, G., Huber, T., Wollgast, J., Christoph, E. H., De Jager, A., et al. (2008). Analysis of perfluorooctanoate (PFOA) and other perfluorinated compounds (PFCs) in the River Po watershed in N-Italy. Chemosphere, 71, 306–313.
Mak, Y. L., Taniyasu, S., Yeung, L. W. Y., Lu, G., Jin, L., Yang, Y., et al. (2009). Perfluorinated compounds in tap water from china and several other countries. Environmental Science and Technology, 43, 4824–4829.
Moody, C. A., & Field, J. A. (2000). Perfluorinated surfactants and the environmental implications of their use in fire-fighting foams. Environmental Science and Technology, 34, 3864–3870.
National Environmental Agency (2008). Daily rainfall at 5 standard stations for the period, January 2006 to December 2007
Ooi, J. B., & Chia, L. S. (1974). The climate of west Malaysia and Singapore. Oxford University Press, New York.
Poulsen, P. B., Jensen, A. A., & Wallström, E (2005) More environmentally friendly alternatives to PFOS-compounds and PFOA, Environmental Protection Agency, Danish Ministry of the Environment.
Prevedouros, K., Cousins, I. T., Buck, R. C., & Korzeniowski, S. H. (2006). Sources, fate and transport of perfluorocarboxylates. Environmental Science and Technology, 40, 32–44.
Rostkowski, P., Yamashita, N., So, I. M. K., Taniyasu, S., Lam, P. K. S., Falandysz, J., et al. (2006). Perfluorinated compounds in streams of the Shihwa industrial zone and lake Shihwa, South Korea. Environmental Toxicology and Chemistry, 25, 2374–2380.
Saito, N., Sasaki, K., Nakatome, K., Harada, K., Yoshinaga, T., & Koizumi, A. (2003). Perfluorooctane sulfonate concentrations in surface water in Japan. Archives of Environmental Contamination and Toxicology, 45, 149–158.
Schultz, M. M., Barofsky, D. F., & Field, J. A. (2004). Quantitative determination of fluorotelomer sulfonates in groundwater by LC MS/MS. Environmental Science and Technology, 38, 1828–1835.
Schultz, M. M., Barofsky, D. F., & Field, J. A. (2006). Quantitative determination of fluorinated alkyl substances by large-volume-injection liquid chromatography tandem mass spectrometry - Characterization of municipal wastewaters. Environmental Science and Technology, 40, 289–295.
Scott, B. F., Spencer, C., Mabury, S. A., & Muir, D. C. G. (2006). Poly and perfluorinated carboxylates in North American precipitation. Environmental Science and Technology, 40, 7167–7174.
Seacat, A. M., Thomford, P. J., Hansen, K. J., Clemen, L. A., Eldridge, S. R., Elcombe, C. R., et al. (2003). Sub-chronic dietary toxicity of potassium perfluorooctanesulfonate in rats. Toxicology, 183, 117–131.
Sinclair, E., & Kannan, K. (2006). Mass loading and fate of perfluoroalkyl surfactants in wastewater treatment plants. Environmental Science and Technology, 40, 1408–1414.
Sinclair, E., Mayack, D. T., Roblee, K., Yamashita, N., & Kannan, K. (2006). Occurrence of perfluoroalkyl surfactants in water, fish, and birds from New York State. Archives of Environmental Contamination and Toxicology, 50, 398–410.
So, M. K., Taniyasu, S., Yamashita, N., Giesy, J. P., Zheng, J., Fang, Z., et al. (2004). Perfluorinated compounds in coastal waters of Hong Kong, South China, and Korea. Environmental Science and Technology, 38, 4056–4063.
So, M. K., Miyake, Y., Yeung, W. Y., Ho, Y. M., Taniyasu, S., Rostkowski, P., et al. (2007). Perfluorinated compounds in the Pearl River and Yangtze River of China. Chemosphere, 68, 2085–2095.
Takagi, S., Adachi, F., Miyano, K., Koizumi, Y., Tanaka, H., Mimura, M., et al. (2008). Perfluorooctanesulfonate and perfluorooctanoate in raw and treated tap water from Osaka, Japan. Chemosphere, 72, 1409–1412.
Taniyasu, S., Kannan, K., Horii, Y., Hanari, N., & Yamashita, N. (2003). A survey of perfluorooctane sulfonate and related perfluorinated organic compounds in water, fish, birds, and humans from Japan. Environmental Science and Technology, 37, 2634–2639.
Taniyasu, S., Kannan, K., So, M. K., Gulkowska, A., Sinclair, E., Okazawa, T., et al. (2005). A method for the analysis of fluorotelomer alcohols, fluorotelomer acids, and short- and long-chain perfluorinated acids in water and biota. Journal of Chromatography A, 1093, 89–97.
Yamashita, N., Kannan, K., Taniyasu, S., Horii, Y., Okazawa, T., Petrick, G., et al. (2004). Analysis of perfluorinated acids at parts-per-quadrillion levels in seawater using liquid chromatography-tandem mass spectrometry. Environmental Science and Technology, 38, 5522–5528.
Yamashita, N., Kannan, K., Taniyasu, S., Horii, Y., Petrick, G., & Gamo, T. (2005). A global survey of perfluorinated acids in oceans. Marine Pollution Bulletin, 51, 658–668.
Yu, J., Hu, J. Y., Tanaka, S., & Fujii, S. (2009). Perfluorooctane sulfonate (PFOS) and perfluorooctanoic acid (PFOA) in sewage treatment plants. Water Research, 43, 2399–2408.
Zushi, Y., Takeda, T., & Masunaga, S. (2008). Existence of nonpoint source of perfluorinated compounds and their loads in the Tsurumi River basin, Japan. Chemosphere, 71, 1566–1573.
Acknowledgement
This research was financially supported by NUS&JSPS. The authors acknowledge the personnel in the sewage treatment plants who aided in sampling.
Author information
Authors and Affiliations
Corresponding author
Rights and permissions
About this article
Cite this article
Hu, J., Yu, J., Tanaka, S. et al. Perfluorooctane Sulfonate (PFOS) and Perfluorooctanoic Acid (PFOA) in Water Environment of Singapore. Water Air Soil Pollut 216, 179–191 (2011). https://doi.org/10.1007/s11270-010-0525-7
Received:
Accepted:
Published:
Issue Date:
DOI: https://doi.org/10.1007/s11270-010-0525-7