Abstract
This review concentrates on studies into the behaviour of natural articular cartilage under boundary lubrication. This includes investigations into the chemical composition at the surface of cartilage, carried out as a means of identifying the boundary lubricant. Studies on the friction of cartilage sliding against cartilage and cartilage sliding steel or glass under conditions expected to be in the boundary regime are described. Additionally, model studies on the possible mechanisms of boundary lubrication using well-defined artificial surfaces are also discussed. Although there appears to be some contradiction between the results of friction measurements, an explanation can, at least in part, be given in terms of the layer of cartilage that is being measured. The different chemical nature and lubricating behaviour of the layers found at or near the surface are discussed in relation to the various results given in the literature.
Similar content being viewed by others
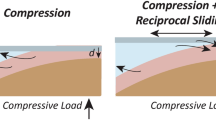
Avoid common mistakes on your manuscript.
1 Introduction
The mechanical, chemical, and biochemical properties of natural articular joints are widely studied for a variety of reasons. On the one hand, it is hoped that a thorough characterisation of natural joints will lead to improved treatments for damage or degenerative disease, such as osteoarthritis and rheumatoid arthritis. Additionally, studies of the lubrication mechanisms of synovial fluid in natural joints often aim to gather sufficient information to mimic these systems either in artificial cartilage or in the aqueous lubrication of man-made systems. A wide range of disciplines and expertise are represented in the field of biotribology and it is, therefore, not realistic to cover all of the literature on natural cartilage in a single review. The excellent tribological properties of natural cartilage can be attributed to many modes of lubrication during reciprocating sliding. Depending on the conditions, lubrication can be in the elastohydrodynamic, mixed or boundary regimes [1–5]. This review concentrates on studies of the boundary lubrication of natural articular cartilage.
2 Structure of Natural Articular Cartilage
The most abundant component of cartilage is water, which makes up about 80% of its weight. The bulk is composed of chondrocytes trapped in an extra-cellular matrix (ECM) [6–8]. This ECM consists of a network of collagen, mainly type II, interdispersed with the proteoglycan aggregate as well as interstitial fluid. Collagen makes up approximately 50–75% of the dry weight of articular cartilage whereas proteoglycans account for approximately 15–30% [7]. The biomechanical properties of cartilage are generally attributed to aggregate trapped in the collagen network due to its large size. Aggregate carries a high negative charge and draws in fluid creating a high osmotic pressure.
Articular cartilage has a hierarchical structure with the highest concentration of collagen in the superficial, or tangential, zone that is situated immediately below the articular surface (Fig. 1) [8]. The collagen in the superficial zone is orientated tangential to surface, imparting a resistance to shear stress [9]. In the transitional, or middle, zone, the collagen is arranged obliquely and the major component is proteoglycan. The radial, or deep zone, is generally believed to be responsible for the good resistance to compression and the distribution of load. In this zone, the collagen is distributed vertically. The lowest zone, called the calcified zone, contains collagen type 10 and spans the calcified and non-calcified regions [8].
3 Surface of Natural Articular Cartilage
Although the surface plays a crucial role in determining the friction properties of a tribological system, not only the precise composition, but also the position of the surface of cartilage, is the subject of some controversy. The uppermost layer is often referred to as the superficial amorphous layer or lamina splendens; however, the nature of this layer has been variously reported in different studies.
The first observation of freshly removed cartilage is, generally, the hydrophobic nature of the surface [10]. Environmental scanning electron microscopy (ESEM) and atomic force microscopy (AFM) were used to demonstrate that this uppermost layer dries rapidly and becomes hydrophobic after removal of the synovial fluid [11]. Additionally, this layer becomes less hydrophobic and eventually dissolves in phosphate buffered saline (PBS). Removal of the uppermost layer allowed a further non-collageneous, granular, hydrophilic layer to be observed. AFM and ESEM showed a clear distinction between both of these layers and the collageneous bulk of the articular cartilage (Fig. 2). The ease of removal of the uppermost layer led to the definition of this as the superficial layer and the second, more robust, layer was defined as being the surface [11].
Sample preparation for scanning and transmission electron microscopy (SEM and TEM) often involves removal of the superficial layer and articular surface, resulting in imaging of the collageneous, tangential zone (Fig. 1). Various procedures have been used to remove the surface including ultrasonication, enzyme digestion and gauze scouring [12, 13]. Electron microscopy has also been carried out on the intact surface [14]. The samples were stored in Ringers solution, thus removing the superficial layer (Fig. 2a), and the surface was imaged with ESEM and TEM before and after treatment with sodium dodecylsulphate (SDS). It was found that SDS, a reagent used to reversibly denature proteins, resulted in exposure of the collagen fibres in the tangential zone that were not visible prior to treatment [14].
Cartilage begins to lose water as soon as it is removed from the synovial fluid and this dehydration leads to changes in the structure that are visible in electron microscopy [15–18]. Atomic force microscopy, therefore, is usually carried out in a physiological solution such as PBS. Samples imaged after briefly washing or incubating the samples with PBS have shown a non-fibrous, highly viscous layer that has been described as featureless and gel-like [11, 19–21]. Changes were observed in the mechanical properties of this gel-like layer over time when the cartilage was submersed in PBS [11]. A decrease in adhesion between the AFM tip and the cartilage sample was accompanied by a decrease in the effective modulus of the gel-like layer, which eventually dissolved in the PBS (Fig. 3). This behaviour was not observed when the sample was submersed in synovial fluid [11]. Sawae et al. observed a granular structure upon magnification of the images that appears similar to that observed when the gel-like layer was removed with PBS [11, 21].
AFM measurements have also been used to follow the effect of enzymes on the imaged layer [19–21]. In one study, treatment with chondroitinase resulted in exposure of the collageneous, tangential zone [21], whereas, in another, wiping the surface and treatment with chondroitinase did not completely remove the upper layer [20]. It is possible that in these studies different amounts of the superficial layer (Fig. 2a) are present on the articular surface. Kumar et al. found chondroitinase partially removed and that hyaluronidase had no effect on the imaged layer but that this was removed to expose collagen upon treatment with alkaline protease [19].
Two collagen-poor layers above the superficial, tangential zone were identified and investigated by Orford and Gardner [22]. The uppermost layer was found to be approximately 50 nm thick and highly anionic but did not contain the sulphated glycosaminoglycans chondroitin sulphate and keratin sulphate. This layer was shown not to contain hyaluronan by its resistance to hyaluronidase digestion. The second layer below this was found to be up to 400 nm thick and hyaluronidase labile. This layer contained chondroitin sulphate and it was, therefore, proposed that it was made up of proteoglycan forming a continuity between the bulk cartilage and the surface [22]. The anionic nature of the surface was also demonstrated by staining with cationic ferritin. It was found that this layer became thinner at 4–8 weeks after removal of the cartilage from the joint [12].
Biochemical analyses of the uppermost layer of articular cartilage found that this was made up of protein and glycosaminoglycan, suggesting the presence of proteoglycans, and phospholipid [14]. Hydroxyproline was not detected, thus indicating that collagen was not present in this layer. The samples were incubated in Ringer’s solution before the layer was removed, therefore, this layer is most probably the surface shown in Fig. 2b. The recovery of this layer in the absence of synovial fluid was investigated by applying a static load to the cartilage submersed in Ringer’s solution. A surface amorphous layer did reform, but this had a very different biochemical composition to the original surface [14].
4 Boundary Lubricating Components of Synovial Fluid
The observation that the uppermost layer of natural cartilage is hydrophobic led to the proposition that natural joints are lubricated by phospholipids [10, 23, 24]. The phospholipids at the surface of cartilage have been analysed in detail using gas chromatography and electrospray ionisation mass spectrometry [25]. The major components were found to be phosphatidylcholine, phosphatidylethanolamine and sphingomyelin; however, the cartilage samples were treated with mixtures of chloroform and methanol and, therefore, proteins or proteoglycans could not be detected with the techniques used [25].
Ogston and Stanier concluded that hyaluronan contributes to the lubricating properties of synovial fluid following investigations into flow-resistance [26–28]. A load was applied to the spherical surface of a glass lens on a glass plate and the interference fringes were measured with a microscope [28]. With air, buffer and serum that had been diluted to give the same protein concentration as synovial fluid, contact between the surfaces was observed at low loads. No significant difference in the diameter of the rings could be detected. In synovial fluid, a slow change in the rings was observed. An irreversible, final state was reached at all loads. It was concluded that synovial fluid had the capacity to form a layer with resistance to static compression that may be responsible for boundary lubrication [28].
In our laboratories, the superficial layer was removed mechanically from the articular surface of natural bovine cartilage and analysed for phospholipid, protein, glycosaminoglycan and hyaluronan [29]. It was found that the uppermost hydrophobic layer that forms on removal of the cartilage from synovial fluid was made up mostly of phospholipid. The superficial layer also contained protein and sulphated glycosaminoglycan but the major component was hyaluronan [29]. Investigations on the interaction of hyaluronan with di-palmitoyl phosphatidylcholine have provided evidence for the formation of a supramolecular complex. It was also shown that the presence of phospholipid in hyaluronan solutions caused a significant decrease in viscosity [30]. Therefore, the formation of a low-shear strength film with the two components is possible.
The strongest evidence for involvement in the tribological system in natural joints lies with proteoglycan 4 (PRG4). PRG4 is widely known as lubricin, which is homologous to superficial zone protein (SZP) [31–34]. SZP has a molecular weight of approximately 345 kDa and lubricin of approximately 230–280 kDa. Differences in the protein molecular weight of lubricin and SZP are due to the splicing of exons, nucleic acid sequences in RNA. Chondroitin sulphate was not detected in lubricin whereas this was found, in small amounts, in SZP [33, 35]. The lubricating ability of lubricin has been attributed to the high carbohydrate content of approximately 50 wt.% [36].
It is widely believed that lubricin acts as a boundary lubricant by attaching to the articular surface [37]. Lubricin has been extracted from the surface of cartilage with PBS containing a high salt concentration (1.5 M NaCl). Staining of the extracted tissue confirmed that lubricin had been removed and also that this layer could reform upon incubation in synovial fluid [38, 39]. Disruption of disulphide bonds by reduction and alkylation reduced the ability of lubricin to bind to the cartilage [38]. Breakage of the disulphide bonds has also been shown to reduce the ability of lubricin to aggregate [40]. Lubricin-mutant mice were bred to demonstrate the importance of lubricin in protecting natural joints from wear [41]. With age, the joints of mice that lacked lubricin showed abnormal deposits of protein on the cartilage. It was concluded from these studies that lubricin not only protects the surface of cartilage, but also plays a role in the control of synovial cell growth. Additionally, the results suggested that lubricin is required to prevent the adsorption of the plasma proteins onto cartilage [41]. As well as preventing non-specific protein adsorption, lubricin has also been shown to reduce cartilage–cartilage integration [41, 42].
Incubating cartilage samples in 1.5 M NaCl solutions leads to removal of the articular surface to expose the tangential zone (Fig. 4). As this treatment has also been used to remove lubricin, it is possible that lubricin is not the boundary lubricant but actually constitutes the surface of the cartilage. The detection of lubricin and SZP, and the exposure of the collageneous tangential zone on removing these suggest that the lower of the two non-collageneous, surface layers detected by Orford and Gardner is SZP and the upper layer is lubricin (Fig. 5) [22]. The most abundant protein in synovial fluid is albumin and as this does not play a role in natural lubrication, the articular surface must have some degree of protein resistance. It would also be highly disadvantageous if opposing cartilage surfaces were capable of integrating with each other. Further properties that make lubricin and SZP ideal candidates to form the articular surface are their wear resistance, low friction and ability to aggregate and attach to the tangential zone. If this is the case, then the boundary lubricant in synovial fluid must be able to adsorb onto or attach to lubricin to form a low-shear strength layer. As lubricin is a hyaluronan-binding protein [43], this would provide further support for the proposal that the polysaccharide is involved in boundary lubrication.
5 Friction Measurements on Cartilage
Kawai et al. measured the friction coefficients for cartilage from the temporomandibular joint and found values in an intact joint of 0.0164 ± 0.0020. Washing the joint with PBS caused an increase in the friction coefficient to 0.0223 ± 0.0050 and scouring the surface of the cartilage with gauze resulted in a further increased to 0.0398 ± 0.0047 [13]. A similar friction behaviour was observed for cartilage sliding against cartilage in canine hip joints where the friction coefficient increased from 0.007 ± 0.004 in the intact joints to 0.020 ± 0.009 after washing [44]. Papain injection into rabbit stifles, the joint in the hindlimbs, in vitro also caused an increase in the friction coefficient from 0.0089 ± 0.0010 in the intact joints to 0.0131 ± 0.0024 following papain treatment [45]. Papain injection caused a slight degeneration of the articular surface and it was found that hyaluronan solutions could reduce the friction coefficients of the damaged samples. On the other hand, when the articular surface was further degenerated by transection of the anterior cruciate ligament, the friction coefficient increased to 0.020 ± 0.008 and hyaluronan solutions did not cause a reduction in the friction coefficient [45]. When the friction coefficient of bovine cartilage versus cartilage was measured first in PBS and then in synovial fluid, a drop was observed from approximately 0.05 to 0.01 [46]. It was, therefore, concluded that the synovial fluid acts as a boundary lubricant in natural articular joints.
Schmidt et al. investigated the influence of synovial fluid and individual components on friction between cartilage surfaces [47]. The samples were washed thoroughly with PBS before friction measurements, thus removing the superficial layer. An increase in the friction coefficient was observed with increasing dilution of the synovial fluid with PBS. The friction coefficient decreased from 0.29 ± 0.01 in PBS to 0.12 ± 0.01 on addition of hyaluronan and to 0.11 ± 0.01 on addition of PRG4 [47]. The largest drop in friction, to 0.066 ± 0.003, was observed when a mixture of hyaluronan and PRG4 was used as lubricant although this value is still higher than that found for synovial fluid (0.025 ± 0.005). Phospholipid, on its own and together with hyaluronan and PRG4, was found to have little effect on the friction coefficient. Phospholipids were found to have an effect on the lubrication of rabbits with experimental osteoarthritis induced by transection of the anterior cruciate and medial collateral ligament. Solutions of hyaluronan and phospholipid reduced the friction coefficient to a point where it was not significantly different from that in the healthy joint; however, the friction was also not significantly different to that of the osteoarthritic cartilage [48].
The static and kinetic friction coefficients for bovine cartilage sliding against cartilage in Ringer’s solution showed an increase with loading time. The initial kinetic friction coefficient rose to a value on approximately 0.04 after 5 min but then showed a small, gradual increase. The static friction rose to high values of approximately 0.4 after loading for 45 min. Interestingly, treatment of the articular surface with SDS, exposing the collageneous tangential zone, did not cause a significant difference to either the static or kinetic friction coefficients [14]. This was attributed to the ability of the cartilage to maintain a fluid-film when sliding against cartilage. However, when the friction coefficients are compared to those measured by others, it appears that not only the superficial layer, but also the articular surface may be removed during sliding in Ringer’s solution [13, 14, 44, 45, 49].
Measurements on cartilage sliding against cartilage are complicated and often steel or glass sliding against cartilage are measured to provide a well-defined contact or facilitate further measurements at the surface [32, 50–56]. Friction measurements on steel sliding against cartilage in Ringer’s solution and synovial fluid show a strong dependence on time with friction coefficients rising to approximately 0.5 after 2 h [50]. The friction in synovial fluid was only slightly lower than that in Ringer’s solution and it was proposed that under the harsh conditions used, the synovial fluid was not able to maintain or replenish the boundary lubricating layer. Alternatively, it was possible that the articular surface was removed both in the synovial fluid and Ringer’s solution exposing the tangential layer [50]. This would suggest that synovial fluid is only effective when interacting with the surface and not when in contact with the collageneous bulk of the cartilage. The effect of loading time and intermittent contact on the friction coefficient of steel sliding against cartilage in physiological saline also showed an increase in friction. It was found that intact samples could initially recover the low friction coefficient when the experiment was paused for 60 s, but this effect became weaker with time. The intact samples were washed with PBS in an ultrasonic bath and gave a significantly lower friction coefficient than samples that had been wiped at the surface [50]. The influence of resting time between tests on the friction coefficient was attributed to rehydration of the cartilage. Steel sliding against cartilage has also been used to study the influence of phospholipids on lubrication [56]. The friction coefficient of propylene glycol was compared to that of solutions of dipalmitoyl phosphatidylcholine in propylene glycol. At ambient temperatures, a slight decrease in the friction coefficient was observed at high phospholipid concentration, however, at 35°C no significant change in friction was observed [56].
An optical apparatus has been used to allow the measurement of the attenuation of reflectance during experiments on a glass prism sliding against cartilage in physiological saline [53, 54]. As in experiments on steel sliding against cartilage, an increase in the friction coefficient with time was observed accompanied by an increase in the attenuation of reflectance. It was concluded from this that an increase in collagen or a decrease in water or saline at the surface of the sample was responsible for increasing friction [54]. However, this could also be viewed as evidence that the non-collageneous, articular surface does play a role in determining the lubrication properties of cartilage. Enzymatic digestion of the cartilage with collagenase was used to demonstrate the importance of the integrity of the collagen network in the friction behaviour [54]. As the collagen network is partially responsible for the mechanical properties, it can be assumed that damaging this will have a fundamental effect on the tribological system.
6 Lubrication of Model Systems
Uncertainties in the degree of degradation of natural cartilage upon removal from the joint, and the complexity of the system, have resulted in the need to investigate the lubricating ability and mechanisms of components of synovial fluid in model systems. Studies on potential lubrication mechanisms at a molecular level have been carried out with the surface forces apparatus (SFA), which allows normal and shear forces to be measured to a very high degree of accuracy [57–62]. Studies on hyaluronan, a negatively charged polyelectrolyte, showed that this was not only squeezed out of the contact between the negatively charged mica surfaces, but additionally, at high loads, it was also squeezed out of the contact between positively charged, modified surfaces [59, 61, 62]. The poor compressibility of the polysaccharide was attributed to strong repulsive forces between negative charges on the polymer chain. It was concluded that hyaluronan could only function as a boundary lubricant if forces between the polymer and the surface were stronger than ionic bonding [59].
The SFA has also been used to investigate the lubrication mechanism of lubricin [40, 58]. It was found that lubricin formed dense layers of thicknesses of 60–100 nm on the negatively charged mica as well as on positively charged and hydrophobic, chemically modified surfaces. The anti-adhesive properties of lubricin were attributed to repulsive normal forces between two layers of lubricin and steric entropic forces similar to those between layers of polymer brushes. At low pressures, low friction coefficients were measured for layers of lubricin adsorbed onto hydrophilic surfaces. The friction increased significantly at pressures above 6 atm; however, the lubricin layer was still able to protect the substrate from damage. The increase in friction at higher pressures was accompanied by a rearrangement of the lubricin layer [40]. Reduction and alkylation of lubricin resulted in the formation of layers that showed a higher friction coefficient at low and high pressures [40].
A widely used model system for cartilage sliding against cartilage is latex sliding against glass [31, 36, 63–65]. The justification for this is generally that glass is often used in studies on the lubrication of cartilage and latex provides a compliant, hydrophobic surface. While cartilage is undoubtedly compliant, the uppermost layer becomes hydrophobic only upon removal of the sample from synovial fluid. Additionally, the superficial layer is often removed from the articular surface before tribological measurements are carried out. The articular surface has been shown to be negatively charged and as albumin, the most abundant protein in synovial fluid, has not been implicated in lubrication, it can be assumed that the surface is either protein resistant or shows specific protein adsorption behaviour. While artificial surfaces can be helpful in determining possible mechanisms of lubrication of individual components of synovial fluid, they suffer a major drawback in studies on synovial fluid due to adsorption of the plasma proteins. Albumin adsorbs readily onto hydrophilic and hydrophobic surfaces, whereas other plasma proteins may be more sensitive to the surface chemistry. Therefore, although the friction between artificial surfaces sliding in synovial fluid may be primarily determined by the adsorption behaviour of albumin, it will also be influenced by the chemical nature of the other, co-adsorbed plasma proteins. As shown by Zappone et al., the chemistry not only determines the relative proportions of proteins on the surface, but it also influences the structure of the adsorbed protein, which in turn influences the friction coefficient [40, 58].
7 Conclusions
A number of conclusions concerning the role of synovial fluid and cartilage components on friction behaviour appear contradictory; however, it is possible that no single component is responsible for lubrication in natural joints. It is important to bear in mind that the whole tribological system contributes to friction behaviour in boundary lubrication. Not only the lubricating ability of synovial fluid but also, for example, the mechanical properties of the bulk and the surface as well as the chemical properties of the surface contribute to determining the friction between the sliding surfaces. Therefore, the integrity of the whole system plays a role in maintaining the good tribological properties of cartilage. That changing any single component leads to an increase in the friction coefficient only indicates that it is a determining part of the system and does not indicate that it is the boundary lubricant. Studies on cartilage sliding against cartilage tend to indicate that removal of the surface has very detrimental effect on friction, suggesting that interactions between the lubricant and the tangential zone do not mimic those between lubricant and surface. A better understanding of natural lubrication may, therefore, be achieved if the chemical nature of the surface was known. Some evidence points to the possibility that this is made up of lubricin. Additionally, the loss of good tribological properties in diseased natural joints may be due to loss of integrity of the bulk cartilage.
References
Dowson, D., Jin, Z.-M.: Micro-elastohydrodynamic lubrication of synovial joints. Eng. Med. 15(2), 63–65 (1986). doi:10.1243/EMED_JOUR_1986_015_019_02
Murakami, T.: The Lubrication in Natural Synovial Joints and Joint Prostheses. JSME Int. J. Ser. III Vibrat. Control Eng. Eng. Ind. 33(4), 465–474 (1990)
Jin, Z.M., Dowson, D.: Elastohydrodynamic Lubrication in Biological Systems. Proc. Inst. Mech. Eng. J. J. Eng. Tribol. 219(J5), 367–380 (2005). doi:10.1243/135065005X33982
Murakami, T., Higaki, H., Sawae, Y., Ohtsuki, N., Moriyama, S., Nakanishi, Y.: Adaptive multimode lubrication in natural synovial joints and artificial joints. Proc. Inst. Mech. Eng. H J. Eng. Med. 212(H1), 23–35 (1998). doi:10.1243/0954411981533791
Graindorge, S., Ferrandez, W., Jin, Z.M., Ingham, E., Fisher, J.: The natural synovial joint: a finite element investigation of biphasic surface amorphous layer lubrication under dynamic loading conditions. Proc. Inst. Mech. Eng. J. J. Eng. Tribol. 220(J8), 671–681 (2006). doi:10.1243/13506501JET95
Ateshian, G.A., Hung, C.T.: The natural synovial joint: properties of cartilage. Proc. Inst. Mech. Eng. J. J. Eng. Tribol. 220(J8), 657–670 (2006). doi:10.1243/13506501JET86
Neville, A., Morina, A., Liskiewicz, T., Yan, Y.: Synovial joint lubrication—does nature teach more effective engineering lubrication strategies? Proc. Inst. Mech. Eng. C J. Eng. Mech. Eng. Sci. 221(10), 1223–1230 (2007)
Dumbleton, J.H.: Tribology of Natural and Artificial Joints. Elsevier, Amsterdam (1981)
Oconnor, P., Orford, C.R., Gardner, D.L.: Role of proteoglycan and collagen in the mechanical-behavior of articular-cartilage. Br. J. Rheumatol. 24(2), 200 (1985)
Hills, B.A.: Boundary lubrication in vivo. Proc. Inst. Mech. Eng. H J. Eng. Med. 214(H1), 83–94 (2000). doi:10.1243/0954411001535264
Crockett, R., Roos, S., Rossbach, P., Dora, C., Born, W., Troxler, H.: Imaging of the surface of human and bovine articular cartilage with ESEM and AFM. Tribol. Lett. 19(4), 311–317 (2005). doi:10.1007/s11249-005-7448-2
Maniwa, S., Nishikori, T., Furukawa, S., Kajitani, K., Ochi, M.: Alteration of collagen network and negative charge of articular cartilage surface in the early stage of experimental osteoarthritis. Arch. Orthop. Trauma Surg. 121(4), 181–185 (2001). doi:10.1007/s004020000203
Kawai, N., Tanaka, E., Takata, T., Miyauchi, M., Tanaka, M., Todoh, M., van Eijden, T., Tanne, K.: Influence of additive hyaluronic acid on the lubricating ability in the temporomandibular joint. J. Biomed. Mater. Res. A 70A(1), 149–153 (2004). doi:10.1002/jbm.a.30078
Graindorge, S., Ferrandez, W., Ingham, E., Jin, Z., Twigg, P., Fisher, J.: The role of the surface amorphous layer of articular cartilage in joint lubrication. Proc. Inst. Mech. Eng. H J. Eng. Med. 220(H5), 597–607 (2006). doi:10.1243/09544119JEIM122
Kirk, T.B., Wilson, A.S., Stachowiak, G.W.: The effects of dehydration on the surface-morphology of articular-cartilage. J. Orthop. Rheumatol. 6(2–3), 75–80 (1993)
Kirk, T.B., Wilson, A.S., Stachowiak, G.W.: The morphology and composition of the superficial zone of mammalian articular-cartilage. J. Orthop. Rheumatol. 6(1), 21–28 (1993)
Bloebaum, R.D., Wilson, A.S.: The structure of the surface of articular-cartilage. J. Anat. 132(Mar), 295 (1981)
Bloebaum, R.D., Wilson, A.S.: The morphology of the surface of articular-cartilage in adult-rats. J. Anat. 131(Sep), 333–346 (1980)
Kumar, P., Oka, M., Toguchida, J., Kobayashi, M., Uchida, E., Nakamura, T., Tanaka, K.: Role of uppermost superficial surface layer of articular cartilage in the lubrication mechanism of joints. J. Anat. 199, 241–250 (2001). doi:10.1046/j.1469-7580.2001.19930241.x
Jurvelin, J.S., Muller, D.J., Wong, M., Studer, D., Engel, A., Hunziker, E.B.: Surface and subsurface morphology of bovine humeral articular cartilage as assessed by atomic force and transmission electron microscopy. J. Struct. Biol. 117(1), 45–54 (1996). doi:10.1006/jsbi.1996.0068
Sawae, Y., Murakami, T., Matsumoto, K., Horimoto, M.: Study on morphology and lubrication of articular cartilage surface with atomic force microscopy. J. Jpn. Soc. Tribol. 45(2), 150–157 (2000)
Orford, C.R., Gardner, D.L.: Ultrastructural histochemistry of the surface lamina of normal articular-cartilage. Histochem. J. 17(2), 223–233 (1985). doi:10.1007/BF01003221
Hills, B.A., Crawford, R.W.: Normal and prosthetic synovial joints are lubricated by surface-active phospholipid—a hypothesis. J. Arthroplasty 18(4), 499–505 (2003). doi:10.1016/S0883-5403(03)00072-X
Hills, B.A., Monds, M.K.: Enzymatic identification of the load-bearing boundary lubricant in the joint. Br. J. Rheumatol. 37(2), 137–142 (1998). doi:10.1093/rheumatology/37.2.143
Sarma, A.V., Powell, G.L., LaBerge, M.: Phospholipid composition of articular cartilage boundary lubricant. J. Orthop. Res. 19(4), 671–676 (2001). doi:10.1016/S0736-0266(00)00064-4
Ogston, A.G., Stanier, J.E.: On the state of hyaluronic acid in synovial fluid. Biochem. J. 46(3), 364–376 (1950)
Ogston, A.G., Stanier, J.E.: The dimensions of the particle of hyaluronic acid complex in synovial fluid. Biochem. J. 49(5), 585–590 (1951)
Ogston, A.G., Stanier, J.E.: The physiological function of hyaluronic acid in synovial fluid—viscous, elastic and lubricant properties. J. Physiol. 119(2–3), 244–252 (1953)
Crockett, R., Grubelnik, A., Roos, S., Dora, C., Born, W., Troxler, H.: Biochemical composition of the superficial layer of articular cartilage. J. Biomed. Mater. Res. A 82A(4), 958–964 (2007). doi:10.1002/jbm.a.31248
Crescenzi, V., Taglienti, A., Pasquali-Ronchetti, I.: Supramolecular structures prevailing in aqueous hyaluronic acid and phospholipid vesicles mixtures: an electron microscopy and rheometric study. Colloids Surf. A Physicochem. Eng. Asp. 245(1–3), 133–135 (2004). doi:10.1016/j.colsurfa.2004.06.030
Jay, G.D., Lane, B.P., Sokoloff, L.: Characterization of a bovine synovial-fluid lubricating factor. 3. The Interaction with hyaluronic-acid. Connect. Tissue Res. 28(4), 245–255 (1992). doi:10.3109/03008209209016818
Swann, D.A., Silver, F.H., Slayter, H.S., Stafford, W., Shore, E.: The molecular-structure and lubricating activity of lubricin isolated from bovine and human synovial-fluids. Biochem. J. 225(1), 195–201 (1985)
Jay, G.D., Tantravahi, U., Britt, D.E., Barrach, H.J., Cha, C.J.: Homology of lubricin and superficial zone protein (SZP): products of megakaryocyte stimulating factor (MSF) gene expression by human synovial fibroblasts and articular chondrocytes localized to chromosome 1q25. J. Orthop. Res. 19(4), 677–687 (2001). doi:10.1016/S0736-0266(00)00040-1
Jay, G.D., Britt, D.E., Cha, C.J.: Lubricin is a product of megakaryocyte stimulating factor gene expression by human synovial fibroblasts. J. Rheumatol. 27(3), 594–600 (2000)
Swann, D.A., Hendren, R.B., Radin, E.L., Sotman, S.L., Duda, E.A.: The lubricating activity of synovial-fluid glycoproteins. Arthritis Rheum. 24(1), 22–30 (1981). doi:10.1002/art.1780240104
Jay, G.D., Harris, D.A., Cha, C.J.: Boundary lubrication by lubricin is mediated by O-linked beta(1–3)Gal-GalNAc oligosaccharides. Glycoconj. J. 18(10), 807–815 (2001). doi:10.1023/A:1021159619373
Jay, G.D., Torres, J.R., Rhee, D.K., Helminen, H.J., Hytinnen, M.M., Cha, C.J., Elsaid, K., Kim, K.S., Cui, Y.J., Warman, M.L.: Association between friction and wear in diarthrodial joints lacking lubricin. Arthritis Rheum. 56(11), 3662–3669 (2007). doi:10.1002/art.22974
Jones, A.R.C., Gleghorn, J.P., Hughes, C.E., Fitz, L.J., Zollner, R., Wainwright, S.D., Caterson, B., Morris, E.A., Bonassar, L.J., Flannery, C.R.: Binding and localization of recombinant lubricin to articular cartilage surfaces. J. Orthop. Res. 25(3), 283–292 (2007). doi:10.1002/jor.20325
Nugent-Derfus, G.E., Chan, A.H., Schumacher, B.L., Sah, R.L.: PRG4 exchange between the articular cartilage surface and synovial fluid. J. Orthop. Res. 25(10), 1269–1276 (2007). doi:10.1002/jor.20431
Zappone, B., Greene, G.W., Oroudjev, E., Jay, G.D., Israelachvili, J.N.: Molecular aspects of boundary lubrication by human lubricin: Effect of disulfide bonds and enzymatic digestion. Langmuir 24(4), 1495–1508 (2008). doi:10.1021/la702383n
Schaefer, D.B., Wendt, D., Moretti, M., Jakob, M., Jay, G.D., Heberer, M., Martin, I.: Lubricin reduces cartilage–cartilage integration. Biorheology 41(3–4), 503–508 (2004)
Englert, C., McGowan, K.B., Klein, T.J., Giurea, A., Schumacher, B.L., Sah, R.L.: Inhibition of integrative cartilage repair by proteoglycan 4 in synovial fluid. Arthritis Rheum. 52(4), 1091–1099 (2005). doi:10.1002/art.20986
Jay, G.D., Torres, J.R., Warman, M.L., Laderer, M.C., Breuer, K.S.: The role of lubricin in the mechanical behavior of synovial fluid. Proc. Natl. Acad. Sci. USA 104(15), 6194–6199 (2007). doi:10.1073/pnas.0608558104
Mabuchi, K., Tsukamoto, Y., Obara, T., Yamaguchi, T.: The effect of additive hyaluronic-acid on animal joints with experimentally reduced lubricating ability. J. Biomed. Mater. Res. 28(8), 865–870 (1994). doi:10.1002/jbm.820280805
Obara, T., Mabuchi, K., Iso, T., Yamaguchi, T.: Increased friction of animal joints by experimental degeneration and recovery by addition of hyaluronic acid. Clin. Biomech. (Bristol, Avon) 12(4), 246–252 (1997). doi:10.1016/S0268-0033(97)00004-1
Schmidt, T.A., Sah, R.L.: Effect of synovial fluid on boundary lubrication of articular cartilage. Osteoarthr. Cartil. 15(1), 35–47 (2007). doi:10.1016/j.joca.2006.06.005
Schmidt, T.A., Gastelum, N.S., Nguyen, Q.T., Schumacher, B.L., Sah, R.L.: Boundary lubrication of articular cartilage—role of synovial fluid constituents. Arthritis Rheum. 56(3), 882–891 (2007). doi:10.1002/art.22446
Kawano, T., Miura, H., Mawatari, T., Moro-Oka, T., Nakanishi, Y., Higaki, H., Iwamoto, Y.: Mechanical effects of the intraarticular administration of high molecular weight phospholipid on synovial joint articular cartilage degeneration hyaluronic acid plus lubrication and prevention of in experimental osteoarthritis. Arthritis Rheum. 48(7), 1923–1929 (2003). doi:10.1002/art.11172
Mabuchi, K., Obara, T., Ikegami, K., Yamaguchi, T., Kanayama, T.: Molecular weight independence of the effect of additive hyaluronic acid on the lubricating characteristics in synovial joints with experimental deterioration. Clin. Biomech. (Bristol, Avon) 14(5), 352–356 (1999). doi:10.1016/S0268-0033(98)00084-9
Forster, H., Fisher, J.: The influence of continuous sliding and subsequent surface wear on the friction of articular cartilage. Proc. Inst. Mech. Eng. H J. Eng. Med. 213(H4), 329–345 (1999). doi:10.1243/0954411991535167
Gleghorn, J.P., Bonassar, L.J.: Lubrication mode analysis of articular cartilage using Stribeck surfaces. J. Biomech. 41(9), 1910–1918 (2008). doi:10.1016/j.jbiomech.2008.03.043
Krishnan, R., Caligaris, M., Mauck, R.L., Hung, C.T., Costa, K.D., Ateshian, G.A.: Removal of the superficial zone of bovine articular cartilage does not increase its frictional coefficient. Osteoarthritis Cartilage 12(12), 947–955 (2004). doi:10.1016/j.joca.2004.08.009
Naka, M.H., Hattori, K., Ikeuchi, K.: Evaluation of the superficial characteristics of articular cartilage using evanescent waves in the friction tests with intermittent sliding and loading. J. Biomech. 39(12), 2164–2170 (2006). doi:10.1016/j.jbiomech.2005.06.015
Naka, M.H., Hattori, K., Ohashi, T., Ikeuchi, K.: Evaluation of the effect of collagen network degradation on the frictional characteristics of articular cartilage using a simultaneous analysis of the contact condition. Clin. Biomech. (Bristol, Avon) 20(10), 1111–1118 (2005). doi:10.1016/j.clinbiomech.2005.06.009
Naka, M.H., Morita, Y., Ikeuchi, K.: Influence of proteoglycan contents and of tissue hydration on the frictional characteristics of articular cartilage. Proc. Inst. Mech. Eng. H J. Eng. Med. 219(H3), 175–182 (2005). doi:10.1243/095441105X34220
Ozturk, H.E., Stoffel, K.K., Jones, C.F., Stachowiak, G.W.: The effect of surface-active phospholipids on the lubrication of osteoarthritic sheep knee joints. Friction. Tribol. Lett. 16(4), 283–289 (2004). doi:10.1023/B:TRIL.0000015204.41674.d3
Klein, J.: Molecular mechanisms of synovial joint lubrication. Proc. Inst. Mech. Eng. J J. Eng. Tribol. 220(J8), 691–710 (2006). doi:10.1243/13506501JET143
Zappone, B., Ruths, M., Greene, G.W., Jay, G.D., Israelachvili, J.N.: Adsorption, lubrication, and wear of lubricin on model surfaces: polymer brush-like behavior of a glycoprotein. Biophys. J. 92(5), 1693–1708 (2007). doi:10.1529/biophysj.106.088799
Tadmor, R., Chen, N.H., Israelachvili, J.: Normal and shear forces between mica and model membrane surfaces with adsorbed hyaluronan. Macromolecules 36(25), 9519–9526 (2003). doi:10.1021/ma030379k
Tadmor, R., Chen, N.H., Ismelachvili, J.N.: Normal AND shear forces BETWEEN MODEL MEMBRANE SURFACES WITH weakly adsorbed hyaluronan. In: 47th Annual Meeting of the Biophysical Society, 2003 Mar 01–05, San Antonio, Texas, 2003. p. 510A–A
Tadmor, R., Chen, N.H., Israelachvili, J.N.: Thin film rheology and lubricity of hyaluronic acid solutions at a normal physiological concentration. J. Biomed. Mater. Res. 61(4), 514–523 (2002). doi:10.1002/jbm.10215
Tadmor, R., Chen, N.H., Israelachvili, J.N.: Thin film rheology and lubricity of hyaluronan solutions. Biophys. J. 82(1), 799 (2002)
Chang, D.P., Abu-Lail, N.I., Guilak, F., Jay, G.D., Zauscher, S.: Conformational mechanics, adsorption, and normal force interactions of lubricin and hyaluronic acid on model surfaces. Langmuir 24(4), 1183–1193 (2008). doi:10.1021/la702366t
Elsaid, K.A., Jay, G.D., Warman, M.L., Rhee, D.K., Chichester, C.O.: Association of articular cartilage degradation and loss of boundary-lubricating ability of synovial fluid following injury and inflammatory arthritis. Arthritis Rheum. 52(6), 1746–1755 (2005). doi:10.1002/art.21038
Jay, G.D., Cha, C.J.: The effect of phospholipase digestion upon the boundary lubricating ability of synovial fluid. J. Rheumatol. 26(11), 2454–2457 (1999)
Author information
Authors and Affiliations
Corresponding author
Rights and permissions
About this article
Cite this article
Crockett, R. Boundary Lubrication in Natural Articular Joints. Tribol Lett 35, 77–84 (2009). https://doi.org/10.1007/s11249-009-9430-x
Received:
Accepted:
Published:
Issue Date:
DOI: https://doi.org/10.1007/s11249-009-9430-x