Abstract
An approach to the synthesis of novel group of negative photochromes has been developed based on the interaction of the tricycanofuran TCF or hydroxytricyanopyrrole HTCP acceptors with methoxy-substituted 2-hydroxybenzaldehydes in ethanol in the presence of ammonium acetate. An essential part of the obtained photochromes is a combination of a phenol fragment and a butadienetricarbonitrile BDTC acceptor connected via a vinylene bridge. The synthesized compounds are sensitive to visible light T-type photochromes. A transformation degree of the initial form, as well as the rate of the reverse dark reaction, can be varied by changing both the position of a methoxy group at aromatic ring and the type of the heterocycle with the BDTC fragment.
Similar content being viewed by others
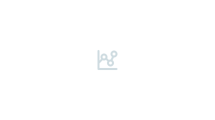
Avoid common mistakes on your manuscript.
Introduction
Photochromic compounds are intensively studied objects because of the great opportunities for their use as photo-switching components for biological research [1,2,3,4,5], various materials [6,7,8], nanosystems [9] and supramolecular chemistry [10, 11]. Most of the works in this field of research are related to positive photochromism. This is the phenomenon when the photo-induced form of a molecule has a deeper color than the original one. The reverse (negative) photochromism, when the colored initial form of a molecule reversibly becomes less colored under the action of irradiation (mostly by visible light), is much less studied.
An obvious advantage for the practical use of negative photochromes is the possibility to use visible light, which is less destructive for organic compounds and living systems than ultraviolet radiation. In this regard, research on new groups of reverse photochromes with widely varying practically significant properties is an important task.
There are several known groups of negative photochromes such as spiropyrans [12,13,14,15], 1,1′-binaphthyl-bridged imidazole dimers [16,17,18], dihydropyrenes [19,20,21] and Stenhouse adducts [22,23,24,25]. Results of the research on the reverse photochromes from all over the world have been recently summarized and collected in two review articles in 2017 and 2018 [26, 27]. However, there is only a little information about a new type of reverse photochrome A, which consists of a tricyanofuran (TCF) moiety (Fig. 1).
Only two examples of compounds A have been described, and an interaction of 2-(3-cyano-4-methylfuran-2(5H)-ylidene)malononitriles (TCF) with salicylic aldehyde was used for the synthesis [28, 29]. During the photochromic transformation, a colored form, A, becomes colorless under the action of visible light due to the formation of a spiro derivative A*, which is able to thermally transform back into the original form A. Based on these single data, it can be assumed that a system C consisting of a butadienetricarbonitrile (BDTC) acceptor and a phenolic fragment, bridged by a double bond, is necessary for photochromic transformations (Fig. 2).
We believe that an investigation of compound C is promising for discovering of a new wide group of negative photochromes, due to a variety of substituents being possible in the phenolic fragment. The literature on the chemistry of photochromes of this series does not reveal the effect of the substituent at the aryl moiety on the photochromic behavior and other physicochemical properties of compound A, since only unsubstituted salicylic aldehyde was used. It is also important that only the TCF fragment is reported as the BDTC acceptor, and there is no information about the use of other acceptors in structures of type C.
In this regard, the purpose of this work is to show the possibility of varying the position of the substituent at the aryl ring, as well as changing the nitrile-rich acceptor, to synthesize novel negative photochromes with easily adjustable physicochemical characteristics.
Experimental
Materials and methods
The progress of the reactions and the purity of the products were monitored by TLC on Sorbfil plates (spots were visualized under UV light, by treatment with iodine vapor, or by heating). The IR spectra were recorded on a FSM-1202 spectrometer with Fourier transform from samples dispersed in mineral oil. The NMR spectra were measured in DMSO-d6 on a Bruker DRX-500 spectrometer using tetramethylsilane as an internal reference. Elemental analyses were performed using a Perkin–Elmer 2400 CHN elemental analyzer. The mass spectra were obtained on a gas chromatograph mass spectrometer (Shimadzu GCMS-QP2010S). The UV spectra were recorded on an Agilent Cary 60 UV–Vis Spectrophotometer. Melting points were determined on an OptiMelt MPA100 device.
Synthesis and spectral data
Typical procedure for the preparation of methoxy substituted (E)-2-(3-cyano-4-(2-hydroxystyryl)-5,5-dimethylfuran-2(5H)-ylidene)malononitriles 2a – d.
To a suspension of 0.199 g (1 mmol) of TCF acceptor 1a in 2 mL of 96% ethanol 1.05 mmol of an appropriate methoxy substituted 2-hydroxybenzaldehyde and 0.077 g (1 mmol) of dry ammonium acetate were added. The resulting mixture was stirred at 40–50 °C for 10 min, then for 1–2 h at room temperature (TLC controlled). After the reaction completion, the mixture was cooled to 0–5 °C then the precipitated product was filtered, washed with cold ethanol and dried in a vacuum desiccator over CaCl2 to constant weight.
(E)-2-(3-cyano-4-(2-hydroxy-3-methoxystyryl)-5,5-dimethylfuran-2(5H)-ylidene)malononitrile 2a. Mp 244–245 °C (dec.).
1H NMR (500.13 MHz, DMSO-d6): δ 1.76 (6H, s, 2CH3), 3.84 (3H, s, OCH3), 6.89 (1H, t, J = 8.0 Hz, C6H3), 7.12 (1H, dd, J = 8.0, 1.2 Hz, C6H3), 7.33 (1H, d, J = 16.5 Hz, CH=), 7.45–7.48 (1H, m, C6H3), 8.27 (1H, d, J = 16.5 Hz, CH=), 10.04 (1H, s, OH). 13C NMR (125.67 MHz, DMSO-d6): δ 25.09, 53.88, 56.19, 97.31, 99.22, 111.36, 112.01, 112.88, 115.09, 115.38, 119.52, 121.02, 121.50, 143.49, 147.92, 148.15, 176.07, 177.45. MS, (EI, 70 eV): m/z (%) 333 [M]+ (100), 318 [M–Me]+ (17), 248 (76). IR (mineral oil, cm−1): 3224 (OH), 2228 (CN). 1631 (C=C). Anal. Calcd for C19H15N3O3: C, 68.46; H, 4.54; N, 12.61. Found: C, 68.32; H, 4.59; N, 12.75.
(E)-2-(3-cyano-4-(2-hydroxy-4-methoxystyryl)-5,5-dimethylfuran-2(5H)-ylidene)malononitrile 2b. Mp 234–235 °C (dec.).
1H NMR (500.13 MHz, DMSO-d6): δ 1.75 (6H, s, 2CH3), 3.81 (3H, s, OCH3), 6.48 (1H, d, J = 2.4 Hz, C6H3), 6.57 (1H, dd, J = 8.9, 2.5 Hz, C6H3), 7.27 (1H, d, J = 16.3 Hz, CH=), 7.83 (1H, d, J = 8.9 Hz, C6H3), 8.16 (1H, d, J = 16.3 Hz, CH=), 11.07 (1H, s, OH). 13C NMR (125.67 MHz, DMSO-d6): δ 25.34, 52.54, 55.47, 94.72, 98.53, 100.86, 107.64, 111.47, 112.02, 112.11, 112.97, 115.07, 132.48, 144.54, 160.81, 164.72, 176.62, 177.28. MS, (EI, 70 eV): m/z (%) 333 [M]+ (100), 318 [M–Me]+ (23), 248 (100). IR (mineral oil, cm−1): 3246 (OH), 2226 (CN). 1624 (C=C). Anal. Calcd for C19H15N3O3: C, 68.46; H, 4.54; N, 12.61. Found: C, 68.39; H, 4.62; N, 12.70.
(E)-2-(3-cyano-4-(2-hydroxy-5-methoxystyryl)-5,5-dimethylfuran-2(5H)-ylidene)malononitrile 2c. Mp 258–259 °C (dec.).
1H NMR (500.13 MHz, DMSO-d6): δ 1.77 (6H, s, 2CH3), 3.76 (3H, s, OCH3), 6.98 (1H, d, J = 8.9 Hz, C6H3), 7.01 (1H, dd, J = 8.9, 3.1 Hz, C6H3), 7.39 (1H, d, J = 3.1 Hz, C6H3), 7.43 (1H, d, J = 16.4 Hz, CH=), 8.18 (1H, d, J = 16.4 Hz, CH=), 10.42 (1H, s, OH). 13C NMR (125.67 MHz, DMSO-d6): δ 25.20, 53.74, 55.74, 97.24, 99.16, 111.34, 112.04, 112.91, 113.08, 115.26, 117.57, 121.40, 121.67, 143.96, 152.45, 153.04, 176.28, 177.41. MS, (EI, 70 eV): m/z (%)333 [M]+ (72), 318 [M–Me]+ (18), 248 (100). IR (mineral oil, cm−1): 3290 (OH), 2222 (CN). 1607 (C=C). Anal. Calcd for C19H15N3O3: C, 68.46; H, 4.54; N, 12.61. Found: C, 68.30; H, 4.64; N, 12.77.
(E)-2-(3-cyano-4-(2-hydroxy-6-methoxystyryl)-5,5-dimethylfuran-2(5H)-ylidene)malononitrile 2d. Mp 282–283 °C (dec.).
1H NMR (500.13 MHz, DMSO-d6): δ 1.76 (6H, s, 2CH3), 3.89 (3H, s, OCH3), 6.57–6.60 (2H, m, C6H3), 7.33 (1H, t, J = 8.3 Hz, C6H3), 7.63 (1H, d, J = 16.5 Hz, CH=), 8.15 (1H, d, J = 16.5 Hz, CH=), 11.04 (1H, s, OH). 13C NMR (125.67 MHz, DMSO-d6): δ 25.87, 53.48, 56.29, 97.23, 98.48, 102.43, 108.88, 110.77, 111.18, 112.11, 112.93, 116.60, 134.92, 140.09, 159.98, 160.62, 177.22, 177.28. MS, (EI, 70 eV): m/z (%) 333 [M]+ (93), 318 [M–Me]+ (20), 248 (100). IR (mineral oil, cm−1): 3226 (OH), 2231 (CN). 1611 (C=C). Anal. Calcd for C19H15N3O3: C, 68.46; H, 4.54; N, 12.61. Found: C, 68.42; H, 4.62; N, 12.73.
Typical procedure for the preparation of methoxy substituted (E)-2-(3-cyano-5-hydroxy-4-(2-hydroxystyryl)-5-methyl-1H-pyrrol-2(5H)-ylidene)malononitriles 2e – h.
To a suspension of 0.2 g (1 mmol) of 2-(3-cyano-5-hydroxy-4,5-dimethyl-1H-pyrrol-2(5H)-ylidene)malononitrile 1b HTCP in 2 mL of 96% ethanol 1.05 mmol of an appropriate methoxy substituted 2-hydroxybenzaldehyde and 0.077 g (1 mmol) of dry ammonium acetate were added. The resulting mixture was strirred at room temperature for 4–8 h (TLC controlled). After the reaction completion, the mixture was cooled to 0–5 °C then precipitated product was filtered, washed with cold ethanol and dried in a vacuum desiccator over CaCl2 to constant weight.
(E)-2-(3-cyano-5-hydroxy-4-(2-hydroxy-3-methoxystyryl)-5-methyl-1H-pyrrol-2(5H)-ylidene)malononitrile 2e. Mp 171–172 °C (dec.).
1H NMR (500.13 MHz, DMSO-d6): δ 1.67 (3H, s, CH3), 3.84 (3H, s, OCH3), 6.87 (1H, t, J = 8.0 Hz, C6H3), 7.09 (1H, dd, J = 8.0, 1.2 Hz, C6H3), 7.19 (1H, s, OH pyrrol), 7.23 (1H, d, J = 16.4 Hz, CH=), 7.28–7.31 (1H, m, C6H3), 8.17 (1H, d, J = 16.4 Hz, CH=), 9.80 (1H, s, OH), 10.53 (1H, s, NH). 13C NMR (125.67 MHz, DMSO-d6): δ 25.90, 44.17, 56.13, 93.61, 99.06, 111.92, 114.13, 114.58, 115.34, 115.96, 119.61, 120.02, 121.75, 141.69, 147.21, 148.12, 160.40, 171.27. MS, (EI, 70 eV): m/z (%) 334 [M]+ (4), 318 (100), 316 [M-H2O]+ (81). IR (mineral oil, cm−1): 3350–3220 (OH, NH), 2223 (CN). 1593 (C=C). Anal. Calcd for C18H14N4O3: C, 64.66; H, 4.22; N, 16.76. Found: C, 64.50; H, 4.31; N, 16.55.
(E)-2-(3-cyano-5-hydroxy-4-(2-hydroxy-4-methoxystyryl)-5-methyl-1H-pyrrol-2(5H)-ylidene)malononitrile 2f. Mp 163–164 °C (dec.).
1H NMR (500.13 MHz, DMSO-d6): δ 1.65 (3H, s, CH3), 3.79 (3H, s, OCH3), 6.49 (1H, t, J = 2.4 Hz, C6H3), 6.54 (1H, dd, J = 8.8, 2.4 Hz, C6H3), 7.11 (1H, s, OH pyrrol), 7.18 (1H, d, J = 16.2 Hz, CH=), 7.64 (1H, d, J = 8.8 Hz, C6H3), 8.07 (1H, d, J = 16.2 Hz, CH=), 10.36, 10.78 (2H, br s, NH, OH). 13C NMR (125.67 MHz, DMSO-d6): δ 26.50, 43.88, 55.80, 93.73, 97.01, 101.84, 107.66, 112.61, 113.65, 114.64, 115.58, 115.86, 131.58, 142.89, 160.29, 161.06, 164.15, 172.40. MS, (EI, 70 eV): m/z (%) 334 [M]+ (3), 318 (50), 316 [M-H2O]+ (85). IR (mineral oil, cm−1): 3355–3230 (OH, NH), 2221 (CN). 1590 (C=C). Anal. Calcd for C18H14N4O3: C, 64.66; H, 4.22; N, 16.76. Found: C, 64.54; H, 4.34; N, 16.60.
(E)-2-(3-cyano-5-hydroxy-4-(2-hydroxy-5-methoxystyryl)-5-methyl-1H-pyrrol-2(5H)-ylidene)malononitrile 2g. Mp 156–157 °C (dec.)
1H NMR (500.13 MHz, DMSO-d6): δ 1.68 (3H, s, CH3), 3.75 (3H, s, OCH3), 6.88 (1H, d, J = 8.9 Hz, C6H3), 6.96 (1H, dd, J = 8.9, 3.0 Hz, C6H3), 7.15 (1H, s, OH pyrrol), 7.20 (1H, d, J = 3.0 Hz, C6H3), 7.33 (1H, d, J = 16.4 Hz, CH=), 8.09 (1H, d, J = 16.4 Hz, CH=), 10.18 (1H, s, OH), 10.50 (1H, s, NH). 13C NMR (125.67 MHz, DMSO-d6): δ 25.87, 44.14, 55.69, 93.59, 98.83, 111.96, 112.08, 114.12, 115.35, 116.21, 117.49, 120.52, 121.73, 142.04, 152.29, 152.50, 160.47, 171.44. MS, (EI, 70 eV): m/z (%) 334 [M]+ (2), 318 (71), 316 [M-H2O]+ (35). IR (mineral oil, cm−1): 3340–3205 (OH, NH), 2226 (CN). 1586 (C=C). Anal. Calcd for C18H14N4O3: C, 64.66; H, 4.22; N, 16.76. Found: C, 64.48; H, 4.35; N, 16.58.
(E)-2-(3-cyano-5-hydroxy-4-(2-hydroxy-6-methoxystyryl)-5-methyl-1H-pyrrol-2(5H)-ylidene)malononitrile 2h. Mp 152–153 °C (dec.).
1H NMR (500.13 MHz, DMSO-d6): δ 1.67 (3H, s, CH3), 3.86 (3H, s, OCH3), 6.57 (2H, d, J = 8.3 Hz, C6H3), 7.27 (1H, t, J = 8.3 Hz, C6H3), 7.13 (1H, s, OH pyrrol), 7.72 (1H, d, J = 16.4 Hz, CH=), 8.27 (1H, d, J = 16.4 Hz, CH=), 10.45 (1H, s, OH), 10.78 (1H, s, NH). 13C NMR (125.67 MHz, DMSO-d6): δ 26.26, 43.69, 56.13, 93.36, 97.94, 102.37, 108.88, 111.07, 112.14, 114.27, 115.48, 118.13, 133.58, 138.72, 159.44, 160.27, 160.55, 172.93. MS, (EI, 70 eV): m/z (%) 334 [M]+ (2), 318 (15), 316 [M-H2O]+ (46). IR (mineral oil, cm−1): 3355–3160 (OH, NH), 2214 (CN). 1597 (C=C). Anal. Calcd for C18H14N4O3: C, 64.66; H, 4.22; N, 16.76. Found: C, 64.53; H, 4.29; N, 16.63.
Results and discussion
In this work, a methoxy group was used as a model substituent; its position was varied across the entire ring of the 2-vinylphenol fragment (Scheme 1). TCF 1a-based tricyanofuran derivative, as well as HTCP 1b-based hydroxytricyanopyrrole derivative, were used as a nitrile-rich acceptor. These two BDTC acceptors were chosen because the TCF derivatives had been already used for the synthesis of negative photochromes of the studied group [28, 29], and the HTCP molecules are the closest structural analogues of TCF from all the structures described in the literature [30]. The similarities between TCF and HTCP are the presence of the BDTC acceptor and the geometry of the substituents in heterocycles. The bigger reactivity is another reason to use the less-studied HTCP acceptors for the synthesis of negative photochromes. They can be used to adjust the physicochemical properties of chromophores if necessary [31].
The starting acceptors are synthetically readily available. TCF 1a was obtained in 52% yield from malononitrile and the hydroxyketone in pyridine in the presence of acetic acid [32], HTCP 1b was synthesized in 78% yield by the reaction of malononitrile dimer with diacetyl in aqueous ethanol in the presence of sodium hydroxide [30]. The synthesis of the desired chromophores 2a–h was carried out by the interaction of acceptors 1a, b with methoxy-substituted salicylic aldehydes in ethanol in the presence of ammonium acetate. The transformation occurred within 1–8 h and resulted in a series of unknown compounds 2a–h with moderate to good yields of 48–90% (Scheme 1; Table 1).
The structure of compounds 2 is considered with the data of IR, 1H and 13C NMR spectroscopy, mass spectrometry and elemental analysis. A characteristic feature of the 1H NMR spectra of compounds 2 is the presence of signals of two vicinal vinyl protons in the 7.27–8.27 ppm region with the coupling constant of 16.2–16.5 Hz, showing the E-configuration of a double bond. The presence of a phenolic hydroxyl is proved by the signal at 9.80–11.07 ppm, while the protons of a methoxy group appear at 3.75–3.89 ppm. The characteristic of 13C NMR spectra of compounds 2 is an appearance of signals of three cyano groups at 111.18–115.48 ppm and of small intense signals of C-atoms of C(CN)2 fragments at 43.69–53.88 ppm. The mass spectra are characterized by the presence of peaks of molecular ions [M]+ with 2–100% intensity.
The light absorption properties of all compounds 2 were studied in THF solutions at 2.5 × 10−5 M. Absorption bands were characterized with maxima in the range of 414–470 nm and the molar extinction coefficient about 2.98–4.59 × 104 (Fig. 3, Table 2). The shortest wavelength absorption (414–431 nm) was observed for the 3- and 6-MeO-derivatives, while 4- and 5-methoxy-substituted chromophores were absorbed at the longest wavelength region (450–470 nm). A type of the heterocycle also affected the absorption properties: the HTCP derivatives 2e–h have a hypsochromic shift of the absorption maxima by 8–18 nm in comparison with the corresponding TCF compounds 2a–d.
The behavior of the THF solutions of compounds 2a–h under irradiation was studied to evaluate the photochromic properties of the synthesized molecules. We found that all the compounds 2a–h show negative photochromism under non-filtered visible light irradiation at 20 °C (Scheme 2).
Colored solutions were irradiated with visible light for 3 min to reach a less-colored photo-stationary state (PSS) (Fig. 4). The observed decrease of the long-wave absorbance in the photostationary state varied from 29% for 5-MeOTCF 2c to 90% for 6-MeOHTCP 2h (Table 2). An initial absorption was restored in the dark at room temperature apparently due to a reverse thermal transformation of the photo-induced form 2* to the starting one 2. Therefore, all the compounds were found to be thermally unstable (T-type) negative photochromes. We analyzed an influence of the methoxy group position to the photo-transformation degree of compounds 2 and found that the largest decrease of an absorption band among two groups of the TCF 2a–d and HTCP 2e–h derivatives is observed for 6-MeO-substituted compounds 2d—52% and 2h—90%. Moreover, it can be noted that the HTCP derivatives generally have a better degree of photo-transformation of the initial form in comparison to TCF.
The kinetic curves of the reverse thermal reaction were recorded. It was found that the half-life period (τ1/2) of the photo-induced form 2* transformation (Scheme 2) depends on the position of a methoxy group and the type of the heterocycle. We found that τ1/2 is varied in the range from 3 s for 4-MeOTCF 2b to 52.5 min for 5-MeOHTCP 2g (Table 2). The smallest thermal stability was observed for a couple of 4-MeO substituted chromophores 2b and 2f (τ1/2 are 3 s and 40 s, respectively). A photo-induced form of 6-MeO-substituted compounds 2d and 2h showed a significantly larger half-life period of about 19–29 min. Accordingly, 3-MeO-substituted chromophores 2a and 2e are intermediate in τ1/2 values which are around 5 min. For these three couples of chromophores, the type of heterocycle affects τ1/2 insignificantly in comparison with the influence of the methoxy group position. The only exceptions are 5-MeO-substituted compounds 2c and 2g for which τ1/2 values differ dramatically—about 0.5 min and 52.5 min, respectively, and the latter is the highest τ1/2 that was observed for chromophores 2 (Table 2).
An interesting feature was the observed difference in the kinetics of the reverse thermal transformation. Assuming that there are only two forms in the solution (2 and 2*), the concentration of the photo-induced form 2* [C2] was evaluated as the difference between the initial concentration (C0 = 2.5 × 10−5 M) and the concentration of the form 2 [C1] during the reverse thermal reaction, in which [C1] was found using the optical density values and known molar absorptivity (ε) from the Beer–Lambert–Bouguer law. Thus, for the thermal coloration of compounds 2c, d, h, the concentrations of the 2* forms were fitted in a pseudo-zero-order kinetic equation (Fig. 5, e.g., compound 2d), whereas for chromophores 2a, b, e–g, the concentrations of the photo-induced forms 2* were fitted for first-order kinetics (Fig. 6; e.g. compound 2e).
A different rate of the reverse thermal reaction is probably related to the structural features of photo-induced forms 2*, which are affected by the position of a methoxy group at the aryl ring and the type of a five-membered heterocycle. It also should be noted that, for the known analogue of structures 2*, a CH-acidity and a dissociation were described [28]. THis probably affects the kinetics of the reverse reaction in the case of the compounds studied in this work.
An important characteristic of the photochromic compounds, which largely defines their practical use, is the cyclicity of the transformations. The HTCP acceptor has not previously been reported in any photochromic system, and therefore we studied the cyclicity of the photo-thermal transformations for chromophore 2f (Fig. 7).
It was found that THF solution of compound 2f after irradiation with visible light until PSS is reached, followed by keeping the solution in the dark for 6 min, restores the initial absorbance (Fig. 7). This process was repeated 10 times, and after that, the solution was kept in dark for about 10 min, and the final solution showed almost no decrease in the optical density in comparison with the initial solution. This result indicates a good fatigue resistance of compound 2f as well as a resistance to photodegradation.
Conclusion
Thus, a series of the TCF and HTCP chromophores have been synthesized, containing a methoxy-substituted 2-vinylphenol fragment. All the obtained compounds were found to be thermally reversible (T-type) negative photochromes in solution, for which a photo-induced form could be generated by the action of visible light. It was found that a degree of photochromic transformation, a thermal stability of the photo-induced form, and the kinetics of the reverse thermal reaction can be varied both by changing the position of the methoxy group at the aryl moiety, and by the type of a nitrile-rich acceptor.
References
W. Szymanski, J.M. Beierle, H.A.V. Kistemaker, W.A. Velema, B.L. Feringa, Chem. Rev. 113, 6114 (2013)
W.A. Velema, W. Szymanski, B.L. Feringa, J. Am. Chem. Soc. 136, 2178 (2014)
J. Broichhagen, J.A. Frank, D. Trauner, Acc. Chem. Res. 48, 1947 (2015)
M. Dong, A. Babalhavaeji, S. Samanta, A.A. Beharry, G.A. Woolley, Acc. Chem. Res. 48, 2662 (2015)
M.M. Lerch, M.J. Hansen, G.M. van Dam, W. Szymanski, B.L. Feringa, Angew. Chem. Int. Ed. 55, 10978 (2016)
M.M. Russew, S. Hecht, Adv. Mater. 22, 3348 (2010)
H. Tian, J. Zhang, Photochromic Materials: Preparation, Properties and Applications (Wiley-VCH, Weinheim, 2016)
R. Klajn, Chem. Soc. Rev. 43, 148 (2014)
L. Wang, Q. Li, Chem. Soc. Rev. 47, 1044 (2018)
M. Natali, S. Giordani, Chem. Soc. Rev. 41, 4010 (2012)
S. Erbas-Cakmak, D.A. Leigh, C.T. McTernan, A.L. Nussbaumer, Chem. Rev. 115, 10081 (2015)
T. Yamaguchi, A. Maity, V. Polshettiwar, M. Ogawa, Inorg. Chem. 57, 3671 (2018)
M.J. Feeney, S.W. Thomas, Macromolecules 51, 8027 (2018)
W. Tian, J. Tian, Dyes Pigm. 105, 66 (2014)
G. Copley, J.G. Gillmore, J. Crisman, G. Kodis, C.L. Gray, B.R. Cherry, B.D. Sherman, P.A. Liddell, M.M. Raquette, L. Kelbauskas, N.L. Frank, A.L. Moore, T.A. Moore, D. Gust, J. Am. Chem. Soc. 136, 11994 (2014)
S. Hatano, T. Horino, A. Tokita, T. Oshima, J. Abe, J. Am. Chem. Soc. 135, 3164 (2013)
T. Yamaguchi, Y. Kobayashi, J. Abe, J. Am. Chem. Soc. 138, 906 (2016)
I. Yonekawa, K. Mutoha, J. Abe, Chem. Commun. 55, 1221 (2019)
C. Bohne, R.H. Mitchell, J. Photochem. Photobiol. C Photochem. Rev. 12, 126 (2011)
K. Ayub, R. Li, C. Bohne, R.V. Williams, R.H. Mitchell, J. Am. Chem. Soc. 133, 4040 (2011)
K. Ayub, R.H. Mitchell, J. Org. Chem. 79, 664 (2014)
S. Helmy, F.A. Leibfarth, S. Oh, J.E. Poelma, C.J. Hawker, J. Read de Alaniz, J. Am. Chem. Soc. 136, 8169 (2014)
M.M. Lerch, M.D. Donato, A.D. Laurent, M. Medved, A. Iagatti, L. Bussotti, A. Lapini, W.J. Buma, P. Foggi, W. Szymański, B.L. Feringa, Angew. Chem. Int. Ed. 57, 1863 (2018)
M.D. Donato, M.M. Lerch, A. Lapini, A.D. Laurent, A. Iagatti, L. Bussotti, S.P. Ihrig, M. Medved, D. Jacquemin, W. Szymański, W.J. Buma, P. Foggi, B.L. Feringa, J. Am. Chem. Soc. 139, 15596 (2017)
M.M. Lerch, W. Szymański, B.L. Feringa, Chem. Soc. Rev. 47, 1910 (2018)
S. Aiken, R.J.L. Edgar, C.D. Gabbutt, B.M. Heron, P.A. Hobson, Dyes Pigm. 149, 92 (2018)
V.A. Barachevsky, Rev. J. Chem. 7, 334 (2017)
C. Yang, T. Khalil, Y. Liao, RSC Adv. 6, 85420 (2016)
V.K. Johns, P. Peng, J. DeJesus, Z. Wang, Y. Liao, Chem. Eur. J. 20, 689 (2014)
M.Yu. Belikov, S.V. Fedoseev, M.Yu. Ievlev, O.V. Ershov, Synth. Commun. 48, 2850 (2018)
S.V. Fedoseev, M.Yu. Belikov, M.Yu. Ievlev, O.V. Ershov, V.A. Tafeenko, Res. Chem. Intermed. 44, 3565 (2018)
W.E. Moerner, R.J. Twieg, D.W. Kline, M. He, Patent US 2007:134737
Acknowledgements
The research was performed under the financial support of the Russian Science Foundation (Grant No. 18-73-10065).
Author information
Authors and Affiliations
Corresponding author
Additional information
Publisher's Note
Springer Nature remains neutral with regard to jurisdictional claims in published maps and institutional affiliations.
Electronic supplementary material
Below is the link to the electronic supplementary material.
Rights and permissions
About this article
Cite this article
Belikov, M.Y., Ievlev, M.Y., Fedoseev, S.V. et al. Novel group of negative photochromes containing a nitrile-rich acceptor: synthesis and photochromic properties. Res Chem Intermed 45, 4625–4636 (2019). https://doi.org/10.1007/s11164-019-03853-w
Received:
Accepted:
Published:
Issue Date:
DOI: https://doi.org/10.1007/s11164-019-03853-w