Abstract
Background and aims
Wetlands are important carbon sinks across the planet. However, soil carbon sequestration in tropical freshwater wetlands has been studied less than its counterpart in temperate wetlands. We compared carbon stocks and carbon sequestration in freshwater wetlands with various geomorphic features (estuarine, perilacustrine and depressional) and various plant communities (marshes and swamps) on the tropical coastal plain of the Gulf of Mexico in the state of Veracruz, Mexico. These swamps are dominated by Ficus insipida, Pachira aquatic and Annona glabra and the marshes by Typha domingensis, Thalia geniculata, Cyperus giganteus, and Pontederia sagittata.
Methods
The soil carbon concentration and bulk density were measured every 2 cm along 80 cm soil profiles in five swamps and five marshes. Short-term sediment accretion rates were measured during a year using horizontal makers in three of the five swamps and marshes, the carbon sequestration was calculated using the accretion rates, and the bulk density and the percentage of organic carbon in the surficial layer was measured.
Results
The average carbon concentration ranged from 50 to 150 gC kg−1 in the marshes and 50 to 225 gC kg−1 in the swamps. When the wetlands were grouped according to their geomorphic features, no significant differences in the carbon stock (P = 0.095) were found (estuarine (25.50 ± 2.26 kgC m−2), perilacustrine (28.33 ± 2.74 kgC m−2) and depressional wetlands (34.93 ± 4.56 kgC m−2)). However, the carbon stock was significantly higher (P = 0.030) in the swamps (34.96 ± 1.3 kgC m−2) than in the marshes (25.85 ± 1.19 kgC m−2). The average sediment accretion rates were 1.55 ± 0.09 cm yr−1 in the swamps and 0.84 ± 0.02 cm yr−1 in the marshes with significant differences (P = 0.040). The rate of carbon sequestration was higher (P = 0.001) in swamp soils (0.92 ± 0.12 kgC m−2 yr−1) than marsh soils (0.31 ± 0.08 kgC m−2 yr−1). Differences in the rates of carbon sequestration associated with geomorphic features were found between the swamp ecosystems (P < 0.05); i.e., higher values were found in the swamps than in the marshes in perilacustrine and estuarine wetlands (P < 0.05). However, no significant differences (P = 0.324) in carbon sequestration rates were found between the marsh and swamp areas of the depressional site.
Conclusions
Swamp soils are more important contributors to the carbon stock and sequestration than are marsh soils, resulting in a reduction in global warming, which suggests that the plant community is an important factor that needs to be considered in global carbon budgets and projects of restoration and conservation of wetlands.
Similar content being viewed by others
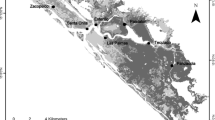
Explore related subjects
Discover the latest articles, news and stories from top researchers in related subjects.Avoid common mistakes on your manuscript.
Introduction
Anthropogenic activities have increased the atmospheric concentrations of greenhouse gases, such as carbon dioxide (CO2), methane (CH4), nitrous oxide (N2O) and water vapor. High concentrations of CO2 have been recognized as the main cause of global warming, which is a worldwide environmental concern due to the threat to life on the planet. According to an intergovernmental panel’s report on climate change, soil carbon sequestration is an inexpensive option for mitigating global warming (IPCC 2007). The majority of studies in which soil carbon sequestration was investigated have focused on forests and croplands (Post et al. 2004; Conant et al. 2011; Mbah and Idike 2011). However, carbon sequestration in wetland soils has been less studied, despite the important role that these ecosystems play in the carbon cycle.
Wetlands are transitional zones between terrestrial and aquatic ecosystems. They are characterized by the presence of standing water and/or saturated soils for a certain period of time during the growing season, a condition that enhances the development of aquatic vegetation and hydric soils (Lewis 2000; Mitsch and Gosselink 2007). Wetlands cover approximately 6 % of the Earth’s land surface (Mitsch and Gosselink 2007). Olmsted (1993) estimated that there are more than 3.3 million hectares of wetlands in Mexico, (approximately 0.6 % of the world’s total wetlands). Approximately 10 % of the country’s wetlands are located in the state of Veracruz, two-thirds of which are freshwater wetlands and the majority of which are on the flat coastal plain (Olmsted 1993; Moreno et al. 2009). The studies of coastal freshwater wetlands in Veracruz have focused primarily on floristic composition and biodiversity (Peralta-Peláez and Moreno-Casasola 2009; Infante et al. 2011). Coastal freshwater wetlands in this area have been classified as herbaceous wetlands (marshes), forested wetlands (swamps), and flooded pastures dotted with palms (Lot-Helgueras 2004; Moreno-Casasola et al. 2010).
Wetlands are one of the most productive ecosystems on Earth and play an important role in carbon (C) cycling (Conner and Day 1976; Pant et al. 2003). Carbon is captured by wetland plants that fix CO2 from the atmosphere and convert it to organic carbon (Adhikari et al. 2009). Aboveground biomass production ranges from 975 to 1,700 g m−2 yr−1 in swamps (Conner and Day 1976; Mitsch et al. 1991; Bernard et al. 1988; Chimner and Ewel 2005; Infante et al. 2012) and ranges from 290 to 1,900 g m−2 yr−1 in marshes (Neue et al. 1997; Thormann and Bayley 1997; Neubauer et al. 2000). In contrast, subsurface biomass production has been found to be higher in marshes (Schubauer and Hopkinson 1984; Giroux and Bedard 1988) than in swamps (1,181 to 3,200 versus 975 to 1,700 g m−2 yr−1, respectively) (Bernard and Solsky 1977; Symbula and Day 1988). When wetland plant senescence occurs, dead plant material falls on the ground and creates a layer of rich organic matter known as peat (Warner et al. 2005). Decomposition rates of organic material in wetland soils are low due to waterlogged conditions that create an anaerobic environment (Benner et al. 1984; Warner et al. 2005; Kayranli et al. 2010). Accumulation of organic matter in soils depends on the ratio of organic matter input to output (Adhikari et al. 2009; Kayranli et al. 2010). Organic matter input and output are a function of the productivity and hydrogeomorphic setting of the wetland. In wetlands connected to open water ecosystems, such as rivers, streams, lakes, and estuaries, organic matter is exported (Brinson et al. 1981), whereas in isolated ecosystems, such as depressional wetlands, organic material export during inundation is low (Craft and Casey 2000).
Temperature is another important factor that influences carbon accumulation. Depolymerization of biochemically complex compounds, enzyme production, and processes that limit the availability of organic matter in the soil are strongly affected by the soil temperature (Kirschbaum 1995; Davidson and Janssens 2006; Conant et al. 2011). Wetlands with high plant productivity and low rates of organic matter decomposition are locations of net carbon accumulation in the soil.
Carbon sequestration is an important environmental service that wetland ecosystems provide. In Mexico, studies of carbon sequestration in wetland soils have focused on brackish wetlands, such as mangrove swamps (Moreno et al. 2002; Moreno-May et al. 2010; Cerón-Bretón et al. 2011). However, soil carbon sequestration in tropical freshwater wetlands has received less attention. The objective of this study was to compare carbon accumulation in soils of freshwater wetlands with various geomorphic features (depressional, perilacustrine and estuarine) and plant community structures (marshes and swamps) in the coastal plain of Veracruz, Mexico. In this study, we defined the soil carbon profile as the distribution of carbon concentration (g C kg−1 soil) in the soil with depth. The soil carbon stock is the amount of carbon contained in the soil at a given depth in per unit area (kg C m−2). The carbon sequestration in the soil was calculated from estimates of its soil carbon stock and sediment accretion rate (kg C m−2 yr−1). The higher aboveground productivity of swamps compared with marshes has been described in the literature. Soil temperatures are lower in swamps than marshes due to tree shading; which tends to reduce the decomposition of organic material and therefore causes it to be stored. Certain authors (Benner et al. 1984; Lorenz et al. 2000) reported that woody vegetation contains a larger amount of recalcitrant compounds, such as lignin, lipids, waxes, cutin and suberin, than does herbaceous vegetation, and these types of compounds have low decomposition rates. We developed the following hypotheses:
-
Hypothesis 1:
We expect that soil carbon sequestration is higher in swamps than marshes.
In contrast, according to the literature, the majority of organic material in depressional wetlands is retained in the ecosystem due to the lower rate of export of organic materials during inundation, and this flooded condition is maintained for longer periods, enhancing the anaerobic conditions in the soil compared with estuarine or perilacustrine wetlands, which are connected to open water. As described previously, we developed a second hypothesis.
-
Hypothesis 2:
Soil carbon sequestration is higher in depressional wetlands than in perilacustrine or estuarine wetlands.
Materials and methods
Study site
The study was conducted in five freshwater marshes and swamps located on the coastal plain of the Gulf of Mexico in Veracruz. The sites were sorted based on their geomorphic characteristic into depressional, perilacustrine and estuarine wetlands (Table 1). The study sites from north to south consisted of Estero Dulce (Municipality of Tecolutla), Laguna Chica (Vega de Alatorre), Santander (Alto Lucero), Boquilla de Oro (Alto Lucero) and La Mancha (Actopan) (Fig. 1). The climate of the coastal plain of the Gulf of Mexico has three seasons: a rainy period (July to October), a period of cold fronts with strong winds and rain (November to February) and a dry season (March to June). The mean annual precipitation fluctuates between 1,200 and 1,650 mm. The mean annual temperature varies between 17 and 37 °C. The locations and description of the study sites are presented in Table 1.
Estero Dulce
The wetlands of Estero Dulce lie between an estuarine system produced by the bifurcation of rivers draining the mangrove swamp in the natural protected area of Ciénega del Fuerte. These freshwater wetlands are connected to a mangrove ecosystem and then to the sea, extending 3 km next to the community of Casitas and emptying into the Casitas estuary.
Laguna Chica
The wetlands of Laguna Chica border a coastal lagoon system. This area has a narrow channel with mangroves that joins two lagoons (Laguna Grande and Laguna Chica). The coastal lagoon system has intermittent communication with the sea, which is temporarily restricted by the formation of a semipermanent barrier during the winter (October to May), when strong winds and currents move sand to close off the opening. The freshwater sources to the lagoons are creeks and runoff particularly from the northern area and the rivers. Swamps and marshes surround the mangrove areas of both lagoons.
Santander
The wetlands of the Santander site are fed primarily by the San Agustín lagoon, located on the north side of the wetlands. The surroundings consist of swamp and marsh wetlands and areas of deforestation and cattle grazing.
Boquilla de Oro
The wetlands of the Boquilla de Oro site are supplied with freshwater flowing from the highlands during the rainy season. Groundwater is the main source of water at this site.
La Mancha
The freshwater marsh and swamp of the La Mancha site are located around an interdune lake, close to the La Mancha lagoon. Fresh groundwater is a major source of water for these ecosystems (Yetter 2004).
Soil sampling
In each type of wetland (marshes and swamps), three sampling plots (1 m2) were selected at random. At these plots, four soil cores (0.80 m deep × 0.05 m diameter) were taken using a Russian peat borer. This corer has thin, sharp-edged walls, providing a core without compaction, distortion, or disturbance. Each core was sectioned with a blade at depth intervals of 2 cm. One of the four cores from each sampling plot was used for bulk density analysis. The soil segments were placed in aluminum pans with a predetermined constant weight, transported to the laboratory and stored below 4 °C until they were dried in an oven.
Composite samples were made using three wet soil samples of the same depth from each sampling plot. The wet composited samples were packed in containers and stored below 4 °C until they were dried at room temperature and analyzed for carbon content.
Carbon analysis
In the laboratory, the wet soil composite samples were mixed again to reduce soil heterogeneity, and visible residues of vegetation were removed. The prepared samples were dried at room temperature, ground and sieved (2 mm). To quantify the amount of organic matter, approximately 2 g of the dried soil samples was placed in pre-weighed crucibles and pretreated with 10 M HCl until no bubbles were observed (Hernandez and Mitsch 2007) to avoid possible carbonate interferences in the soil samples. The samples were then dried at 105 °C until they displayed a constant weight and then burned at 450 °C for 4 h (Craft et al. 1988; Bernal and Mitsch 2008). The organic matter percentage (OM%) was calculated using this formula: OM% = (Constant weight at 105 °C – constant weight at 450 °C)/constant weight at 105 °C.
The organic carbon percentage was calculated as a portion of organic matter, using Van Bemmelen’s factor (0.58) (Post et al. 1982; Wang et al. 2003).
The bulk density was obtained by drying a known volume of sediment at 105 °C (19.64 cm−3) until it reached a constant weight and cooling it to room temperature in desiccators.
The amount of carbon stock (kg C m−2) was calculated using the equation (Moreno et al. 2002; Cerón-Bretón et al. 2011) kg C m−2 = [soil dry weight] * [% CO], where the dry soil weight (kg m−2) = [sampled soil depth] * [bulk density] and the % CO = organic carbon content.
The total carbon storage to a depth of 80 cm was calculated by adding the carbon stored in each of the analyzed soil segments (Moreno et al. 2002; Bernal and Mitsch 2008).
Vertical accretion rate
To assess the annual carbon accumulation in the wetland soils, it is necessary to know the soil accretion rate. This quantity is the thickness of soil accumulated during a defined period of time (cm yr−1). The accretion includes mineral sediment accumulations, organic matter and residual space taken up primarily by gases and water (DeLaune et al. 1989).
Marker horizons in soil
The short-term vertical accretion rate was measured using artificial marker horizons. Three accretion plots (0.25 m2) were selected in each type of wetland in April 2010. The accretion rate was determined in freshwater marshes and swamps of Estero Dulce, Laguna Chica and Boquilla de Oro. La Mancha and Santander were not included in the accretion measurements because these sites were chosen for the study after the accretion plots were investigated. The marker horizons consisted of green glitter and blue sand because these materials stick easily to the soil and their appearance allows for easy detection (Harter and Mitsch 2003). Each sampling plot was divided into four squares (0.0625 m−2), and a layer of each marker was placed (~0.5 cm) until the sediment surface was completely covered in two opposite squares. In each square, two core samples were collected from each marker square one year after the marker horizons were placed. Sophisticated methods for taking soil profiles to determine the rate of soil accretion in wetlands have been described recently. These methods consist of collecting cryogenic soil profiles to obtain intact soil (Wolkotten 1976; Cahoon et al. 1996). The cryogenic method is a widely used but is expensive. In addition, the cryogenic agent tanks are difficult to manage in wetlands. Less sophisticated but economical methods have been used when wetland soils are not saturated with water (Hatton et al. 1983; Cahoon and Turner 1989). In this study, three different methods were used when the soil was wet (cryogenic coring device, corer (specifically Russian peat borer) and freezing). Below is a brief description of the methods used.
Method 1 (cryogenization)
Liquid CO2 was used as a cryogenic agent (Wolkotten 1976). The liquid CO2 was transported in fire extinguishers with two external copper manifolds (0.3 m long) connected to the extinguisher hose, which distributed the liquid CO2 through three nozzles over the area inside a second copper probe (0.4 m long) that covered each manifold. The second copper probe was pointed at the bottom, which helped the probe easily penetrate the soil. The liquid CO2 rapidly froze the surrounding soil where the probe was inserted. The cryogenization process took approximately 1.5 min. After visible soil cryogenization, the copper probes containing a frozen soil profile were removed from the soil. The marker depth in the frozen profile was recorded.
Method 2 (corer)
Soil profiles were obtained using a Russian peat borer. The borer was inserted upside down into the soil to a depth of 20 cm. The marker depth in the soil profile was recorded.
Method 3 (freezing)
Aluminum beverage cans were used (0.065 m diameter × 0.14 m height) in accordance with the method of Cahoon and Turner (1989). The tops of the cans were removed and the cans were inserted upside down into the soil. Once the cans were inserted to the maximum depth (0.06–0.14 m), they were extracted using a knife as a lever, the base was sealed with paraffin film (parafilm®) and the samples were stored vertically in an iced cooler. The cores were frozen at −10 °C for 24 h. The can’s walls were cut with scissors to measure the marker depth on four different sides of the frozen profile. These values were averaged to obtain a final datum for each core.
To obtain the soil accretion rate with each method used, the depths where artificial markers were found (cm yr−1) were averaged for each type of wetland and compared among the three methods.
The annual rate of accumulation of organic carbon was calculated using the formula (Hatton et al. 1983) C sequestration = AR × BD × OC × 102 (g m−2 yr−1), where AR = the accretion rate, BD = the mean bulk density and OC = the mean dry weight percentages of organic carbon. The BD and OC values used to estimate the C sequestration were the values obtained at a 2 cm depth.
Statistical analysis
Statistical analyses were performed using the software SPSS (2009), version 18, for Windows. A Kolmogrov-Smirnov test was used to check normality. The data fitted normal distributions. One-way analysis of variance (ANOVA) was used to find differences in the carbon concentration at various depth ranges in the same ecosystem and between wetlands with different plant communities. A two-way ANOVA with Tukey comparison was used to find differences in the carbon density among wetlands with different geomorphic features and plant communities. The amounts of carbon stock in the depressional and perilacustrine wetlands were the values obtained in marsh and swamp soils at two sites. The amounts of carbon stock in the estuarine wetland were the values obtained in marsh and swamp soils at one site. The same type of analysis was used to detect differences in the accretion and carbon sequestration rates associated with various types of plant communities and geomorphic features. When the variance was not homogeneous, a Games-Howell multiple comparison test was used. A p-value of 0.05 was used to reveal the statistical significance of all of the estimates.
Results
Carbon concentration in soil profiles
The carbon concentration in the wetland soils varied widely among the wetland types. These values ranged from 50 to 150 g C kg−1 in the marshes (Fig. 2) and from 50 to 225 g C kg−1 in the swamps.
Profile of carbon concentration in the soil (g C kg soil−1) on the two wetland communities: swamp (in black lines) and marsh (in gray lines) soils. Soil profiles were grouped according geomorphic features: Depressional wetlands (Boquilla de Oro and La Mancha), Perilacustrine wetlands (Laguna Chica and Santander) and estuarine wetland (Estero Dulce). Each point in the graph is the mean of three composite cores at 2 cm of depth; horizontal bars represent standard errors
The mean carbon concentration in the wetlands with different plant communities was significantly higher in swamps (87.59 ± 6.88 g C kg−1) than marshes (57.59 ± 3.23 g C kg−1) in perilacustrine (P = 0.000) wetlands and in estuarine wetlands (101.04 ± 9.23 and 54.6 ± 2.81 g C kg−1 in swamps and marshes, respectively; P = 0.000). However, in depressional wetlands, the mean carbon concentrations were not significantly different (P = 0.354) between swamp (115.66 ± 10.23 g C kg−1) and marsh soils (125.67 ± 3.06 g C kg−1).
Significant differences were observed with respect to the depth in wetlands with different geomorphic features (P < 0.05). The carbon concentration in the 0–20 cm layer in the depressional wetlands was higher in swamp soils than in marsh soils (P = 0.000), but in the 20–40 cm layer, the carbon concentration was lower in both wetland types (P = 0.617). In the same wetland ecosystem, the carbon concentrations in deeper layers of 40–60 cm (P = 0.016) and 60–80 cm (P = 0.000) were higher in marsh soils than swamp soils. In perilacustrine and estuarine wetlands, the top layer (0–60 cm) of the swamp soils had a higher carbon concentration than that of the marsh soils (P < 0.05), but in the deeper layers (60–80 cm), the pattern of soil carbon concentration were not significantly different in wetlands with different plant communities in both wetlands with different geomorphic features (P > 0.05).
The mean carbon concentration varied significantly between wetlands with different geomorphic features (P = 0.000). The highest carbon concentration was observed in depressional wetlands (120.67 ± 5.33 gC kg−1), followed by estuarine (77.81 ± 6.04 gC kg-1) and perilacustrine (72.59 ± 4.46 gC kg-1) wetland soils. Soils with more than 120 g C kg−1 are classified as organic soils (Richardson and Vepraskas 2000). Based on this criterion, the swamp soils in depressional, perilacustrine and estuarine wetlands contain a layer of organic soil in the top 24, 40 and 28 cm, respectively. In marshes, mineral soils dominate in the top 32, 80 and 80 cm in depressional, perilacustrine and estuarine wetlands, respectively.
Soil carbon stocks
The carbon stock in wetlands with different geomorphic features did not differ statistically (Fig. 3; P = 0.095). The depressional wetland soils contained 34.93 ± 4.56 kgC m−2, followed by perilacustrine (28.33 ± 2.74 kgC m−2) and estuarine wetlands (25.5 ± 2.26 kgC m−2). The type of plant community had no observed effect on the mean values of carbon stock in depressional (P = 0.093), perilacustrine (P = 0.249) and estuarine (P = 0.573) wetland ecosystems. In perilacustrine wetlands, the carbon stocks in marsh and swamp soils, respectively, were 32.62 ± 3.24 and 24.05 ± 2.05 kgC m−2, in estuarine wetlands 26.98 ± 4.58 and 24.02 ± 3.54 kgC m−2 and in depressional wetlands 41.3 ± 7.18 kgC m−2 and 28.56 ± 4.86 kgC m−2. When comparing the mean carbon stock between wetlands with different plant communities (Fig. 3), the carbon stock in swamp soils (34.96 ± 1.3 kgC m−2) was found to be significantly higher (P = 0.030) than the carbon stock in marsh soils (25.85 ± 1.19 kgC m−2).
Comparison of carbon stock (kg C m−2) of the top 80 cm of soil in study tropical wetland sites, classified according to geomorphic features: depressional, perilacustrine, and estuarine wetlands; and comparison of all the swamps vs. all the marshes. Twelve cores in depressional and perilacustrine wetlands and six cores in estuarine wetland were taken. Top and below line of the boxes represents the maximum and minimum values of carbon stock, respectively. Solid line middle of each box is the means value of carbon stock
Soil accretion rates
The soil that had accumulated above the artificial markers (Table 2), obtained by the three methods, was used to estimate the accretion rates. These rates were compared, and no significant differences were found between them (P = 0.937). To compare the accretion rates associated with the various plant communities and geomorphologic features, we used the average of the vertical accretion rate obtained with the three methods. The annual accretion rate of the wetland soils varied from 0.45 to 2.62 cm yr−1 (Table 2). There were no significant differences (P = 0.143) between the rates in the wetlands with different geomorphic features (Table 2). The highest average accretion rate was found in the perilacustrine swamp (2.62 ± 0.05 cm yr−1), followed by the estuarine swamp (1.50 ± 0.02 cm yr−1) and marsh (1.24 ± 0.02 cm yr−1), depressional marsh (0.83 ± 0.02 cm yr−1) and swamp (0.55 ± 0.03 cm yr−1) and perilacustrine marsh (0.45 ± 0.02 cm yr−1), where the lowest accretion rate was found. Significant differences in the annual accretion rates between the swamps (1.55 ± 0.09 cm yr−1) and marshes (0.84 ± 0.02 cm yr−1) (Table 3; P = 0.040) were observed. The combination of plant communities and geomorphic features caused significant differences in the annual rate of accretion (P = 0.031).
Carbon sequestration rates
The carbon sequestration rate varied significantly among the wetlands studied (Fig. 4; P = 0.003). The highest carbon sequestration rates were observed in the estuarine, depressional and perilacustrine swamps (1.18 ± 0.26, 0.82 ± 0.2 and 0.75 ± 0.09 kgC m−2 yr−1, respectively), and these values were nearly 5, 2 and 8 times more than the values found in the estuarine, depressional and perilacustrine marshes (0.25 ± 0.07, 0.57 ± 0.10 and 0.09 ± 0.03 kgC m−2 yr−1, respectively) (Fig. 4). When we compared the carbon sequestration rates between the two types of wetland plant communities (marshes vs. swamps) (Fig. 4), significant differences were observed (P = 0.001). The swamp soils sequestered 66.3 % more carbon (0.92 ± 0.12 kgC m−2 yr−1) than did the marsh soils (0.31 ± 0.08 kgC m−2 yr−1) (Table 3).
Average carbon sequestration rate (kg C m−2 yr−1) of the tropical wetland sites included in this study, grouped according to geomorphic features: depressional, perilacustrine, and estuarine wetlands; and comparison of all the swamps vs. all the marshes. Vertical lines on carbon sequestration values represent standard error of the means. Different letters on top of the bars indicate significant differences between averages
Discussion
Carbon content in soil profiles
In the perilacustrine and estuarine marsh soils, the rapid decrease in carbon concentration with depth indicates that only a small fraction of the carbon deposited with the soil remains there. Other studies described similar patterns of decreasing carbon concentration with depth in wetlands in tropical regions (Jobbágy and Jackson 2000; Donato et al. 2011). Wang et al. (2011) reported that the carbon concentration in marsh soils of the Yellow Delta in China were higher in the upper 0–20 cm; below this depth, the carbon concentration decreased rapidly, which is consistent with the findings of this study. The authors indicated that such results were associated with the moisture content, which also decreased below a depth of 20 cm. However, the swamp soils contained greater carbon concentrations above the middle depths than did the marsh soil profiles. In a palm swamp in Costa Rica, Bernal and Mitsch (2008) observed carbon concentrations between 150 and 230 g C kg−1 in the 0–24 cm depth interval. The carbon concentration decreased to 40 g C kg−1 in the depth range of 36–54 cm. Such values are lower that our results, most likely due to different hydroperiodicity and the differences in the types of trees, which produce a different quantity and quality of organic matter in the soil. Our study is one of the few studies of carbon in Mexican tropical freshwater swamps and indicates the importance of these ecosystems as carbon sinks. In a Micronesian mangrove forest, Kauffman et al. (2011) also reported that the soil carbon content decreases from 210 to 170 g C kg−1 between depths of 0–15 and 90 cm. In the depressional swamps and marshes of this study, the carbon concentration exceeded 100 g C kg−1 throughout the soil profile, suggesting that carbon is maintained in the soil, most likely due to the associated geomorphology, in which the organic material cycle is closed thus reducing export during inundation, compared with open riparian and floodplain wetland ecosystems (Brinson et al. 1981). In the depressional marshes, where no surface water was observed, the groundwater was at a shallow depth below the soil surface, based on saturation observed where we walked (personal observation). This characteristic most likely produced anaerobic conditions and the accumulation of organic carbon in the whole profile.
Carbon stock
Significant differences were found when we grouped the carbon stock values according to their associated plant communities. These differences may be related to the quality of the organic carbon that the plant communities provide. Forest plant communities produce woody tissues with a high amount of recalcitrant organic materials, such as lignin, waxes, cellulose, and hemicellulose, which decompose slowly (Fengel and Wegener 1984; Kögel-Knabner 2002). In contrast, herbaceous plants produce parenchymatic tissue composed of cellulose walls that are more labile (Kögel-Knabner 2002). This result supports our hypothesis 1.
The carbon stock values in the top 20 cm measured in this study are similar to carbon stock values obtained in the top 24 cm in marshes and swamps of Costa Rica (Table 4). However, in the Costa Rican swamps, the carbon stock at depths of 36 cm (11.66 kgCm−2) and 54 cm (15.27 kgCm−2) are nearly 50 % lower than the values found in this study at similar depths. In temperate wetland soils, others have reported carbon stock values similar to those found in this study at a depth of 36 cm depth in a freshwater marsh (14.27 kgCm−2) and in a combined ecosystem with herbaceous and forest plants (21 kgCm−2) (Bernal and Mitsch 2008). In a subtropical region in Japan, Khan et al. (2007) found 5.73 kgCm−2 in the swamp soils at a depth of 54 cm, a value nearly six times smaller than that found in this study at a 60 cm depth in swamp soils. Similarly, our results in marsh soils are higher (almost 25 times) than those reported for African marshes (1.2 kgCm−2) (Bernal and Mitsch 2013). When comparing the carbon stock in swamps obtained in this study with reports from mangrove swamps in Tabasco and Campeche, Mexico, the values were found to be similar at different ranges of depth (Moreno et al. 2002; Cerón-Bretón et al. 2011). The amount of carbon stock in marsh soils was also similar to that reported for marshes in the Mexican Caribbean (Adame et al. 2013). The carbon stock found in the soils of coastal wetlands in the southern Gulf of Mexico indicates that these ecosystems are important reservoirs of carbon and should be considered in estimates of global carbon budgets.
Sediment accretion rates
The highest average accretion rate, which was found in the perilacustrine swamp, might be related to the high productivity at these sites. In a previous study in tropical forested wetlands in Veracruz, Infante et al. (2012) compared litterfall productivity in five forested wetlands and found the highest productivity (1.5 kg m−2 yr−1) in Laguna Chica (site of the perilacustrine ecosystem in this study) compared with that of Ciénega (1.3 kg m−2 yr−1; area near estuarine ecosystem of this study) and other sites studied (0.93–1.2 kg m−2 yr−1). The authors observed that climbers Dalbergia browei (Jacq.) Schinz, and Hippocratea celastroides (Kunth) contributed 75 % of the litterfall productivity. In addition, the microtopography of this site favored the high accumulation of organic material produced by these species. Jeffries et al. (2003) explained that the patterns of accretion can be attributed to the combined effects of topography and organic material, which is similar to our results. Accretion rates found in this study (0.45–2.62 cm yr−1) are higher than values obtained with similar methods in swamps of New Zealand (0.17 cm yr−1; Chapman and Ronalson 1958) and Florida (0.44 cm yr−1; Cahoon and Lynch 1997) and are similar to those found in the freshwater marshes of Spain using sediment traps (1.61–3.87 cm yr−1; Sánchez-Carrillo et al. 2001). In marshes of Louisiana, using radiometric techniques, accretion rates (0.47–1.3 cm yr−1; Hatton et al. 1983; DeLaune et al. 1989; Nyman et al. 2006) similar to those of this study were found.
Soil carbon sequestration
The high values of carbon sequestration observed in this study may be related to the high productivity linked to wetland hydrology, as all the wetlands studied are seasonally flooded. Certain studies have shown that wetlands with pulsing hydrology have higher productivity and retention of carbon in the soil than wetlands that are continuously flooded (Loucks 1990; Sharitz and Mitsch 1993; Middleton 2002). High carbon sequestration values observed in the soils of the perilacustrine swamps are most likely due to the high litterfall productivity of the lianas that grow at this site, as described earlier. The higher values of carbon sequestration in swamps than marshes observed in the perilacustrine and estuarine wetlands were not observed in the depressional wetlands. This finding contradicts hypotheses 2, and therefore this hypothesis was rejected. These results may be related to the hydrology and the accretion rate. The perilacustrine and estuarine swamps exhibited accretion rates higher than that of the depressional swamp. In addition, the soils of the estuarine and perilacustrine swamps are flooded 9 and 7 months of the year, respectively, while those of the depressional swamp are flooded only 5 months (Table 1). This characteristic most likely caused less input of sediment to the ecosystem by water and therefore a lower accretion rate.
The differences in the amounts of carbon sequestration between marsh and swamp soils are related primarily to the high productivity in forested wetlands, which caused more carbon input to the swamp than marsh soils, together with the high accretion rate observed in the forested wetlands. In addition, the high carbon sequestration in swamp soils compared with marsh soils may be due to the differences in the components of the plant tissues. For example, the litterfall of the liana Hippocratea celastroides, a species present in the swamps, has a higher lignin content and decomposes more slowly than Typha domingensis litterfall (unpublished data), a species present in the sampled marshes.
The rates of carbon sequestration in marshes and swamps reported in the literature using short-term accretion techniques were in the range of 0.14 to 0.71 kgC m−2 yr−1 (Table 5). The values obtained using radiometric techniques were in the range of 0.02 to 0.47 kgC m−2 yr−1 (Table 5). In this study, the average estimated rate of carbon sequestration in marsh soils was 0.31 ± 0.08 kgC m−2 yr−1 (Table 3), based on short-term accretion rates measured using artificial marker horizons, which is within the range reported (Table 3). In similar wetlands, certain authors using long-term accretion techniques (Table 5) reported values almost 20 % less that those found in this study. In marshes of coastal Louisiana, USA, Cahoon and Turner (1989) found accretion rates similar to those of this study using a feldspar marker horizon technique (0.50 kgC m−2 yr−1; Table 5). The average rate of carbon sequestration found in swamp soils in this study (0.92 ± 0.12 kgC m−2 yr−1) was two to six times higher than those described in the literature using artificial marker horizons and radiometric techniques, respectively (Table 5). The carbon sequestration rate in this study was calculated using the high accretion rates measured in the short term, and such values may be influencing the trend of high rates of carbon sequestration. However, the soil carbon stocks measured in the studied wetlands are also higher than those reported in the literature, as described above, which indicate that the tropical freshwater wetlands in the southern Gulf of Mexico are an important carbon sink for the planet.
Conclusions
These results indicated that swamp soils are more important carbon sinks than marsh soils, which suggest that the plant community is an important factor that needs to be considered in global carbon budgets and in projects of restoration and conservation of wetlands. Likewise, the hydrology is another characteristic that influences carbon sequestration in wetlands of various geomorphic settings in freshwater swamps. These findings suggest that plant communities in freshwater wetlands and the differentiation between wetlands with different geomorphic features need to be considered in global carbon budgets and included in projects of conservation and restoration of wetlands. The high carbon stocks and carbon sequestration rates measured in this study are evidence of the important role of these wetlands as carbon sinks and reveal the need for conservation of these ecosystems, as the wetlands are important means of regulating carbon levels in the atmosphere and therefore the mitigation of global warming.
References
Adame M, Kauffman J, Medina I, Gamboa J, Torres O, Caamal J, Reza M, Herrera-Silveira J (2013) Carbon stock of tropical coastal wetlands within the Karstic landscape of the Mexican Caribbean. PloS ONE 8:e56569. doi:10.1371/journal.pone.0056569
Adhikari S, Bajracharaya R, Sitaula B (2009) A review of carbon dynamics and sequestration in wetlands. J Wetl Ecol 2:42–46
Benner R, Maccubbin A, Hodson R (1984) Anaerobic biodegradation of the lignin and polysaccharide components of lignocellulose and synthetic lignin by sediment microflora. Appl Environ Microb 47:998–1004
Bernal B, Mitsch WJ (2008) A comparison of soil carbon pools and profiles in wetlands in Costa Rica and Ohio. Ecol Eng 34:311–323
Bernal B, Mitsch WJ (2012) Comparing carbon sequestration in temperate freshwater wetland communities. Global Change Biol 18:1636–1647
Bernal B, Mitsch WJ (2013) Carbon sequestration in freshwater wetlands in Costa Rica and Botswana. Biogeochemistry. doi:10.1007/s10533-012-9819-8
Bernard JM, Solsky BA (1977) Nutrient cycling in a Carex lacustris wetland. Can J Bot 55:630–638
Bernard JM, Solander D, Kvet J (1988) Production and nutrient dynamics in Carex wetlands. Aquat Bot 30:125–147
Brevik E, Homburg J (2004) A 500 year record of carbon sequestration from a coastal lagoon and wetland complex Southern California, USA. Catena 57:221–232
Brinson M, Lugo A, Brown S (1981) Primary productivity, decomposition and consumer activity in freshwater wetlands. Annu Rev Ecol Syst 12:123–161
Cahoon DR, Lynch J (1997) Vertical accretion and shallow subsidence in a mangrove forest of southwestern Florida, U.S.A. Mangroves Salt Marshes 1:173–186
Choon DR, Lynch J, Knaus R (1996) Improved cryogenic device for sampling wetland soils: research method paper. J Sediment Res 66:1025–1027
Cahoon DR, Turner RE (1989) Accretion and canal impacts in a rapidly subsiding wetland; II. Feldspar marker horizon technique. Estuaries 12:260–268
Cerón-Bretón JG, Cerón-Bretón RM, Rangel-Marrón M, Muriel-García M, Cordoba-Quiroz AV, Estrella-Cahuich A (2011) Determination of carbon sequestration rate in soil of a mangrove forest in Campeche, Mexico. Int J Energ Environ 3:328–336
Chapman VJ, Ronaldson JW (1958) The mangrove and salt-marsh flats of the Auckland Isthmus. N Z Dep Sci Ind Res Bull 125:1–79
Chimner RA, Ewel KC (2005) A tropical freshwater wetland: II. Production, decomposition, and peat formation. Wetl Ecol Manag 13:671–684
Conant R, Ryan M, Ågrin G et al (2011) Temperature and soil organic matter decomposition rates –synthesis of current knowledge and a way forward. Global Change Biol 17:3392–3404
Conner W, Day J (1976) Productivity and composition of a bald cypress-water tupelo site and a bottomland hardwood site in a Louisiana swamp. Am J Bot 63:1354–1364
Craft CB, Broome SW, Seneca ED (1988) Nitrogen, phosphorus and organic carbon pools in natural and transplanted marsh soils. Estuaries 11:272–280
Craft C, Casey W (2000) Sediment and nutrient accumulation in floodplain and depressional freshwater wetlands of Georgia, USA. Wetlands 20:323–332
Davidson E, Janssens I (2006) Temperature sensitivity of soil carbon decomposition and feedbacks to climate change. Nature 440:165–173
DeLaune RD, Whitcomb J, Jr P, Pardue J, Pezashki SR (1989) Accretion and canal impacts in a rapidly subsiding wetland. Techniques 137Cs and210 Pb. Estuaries 12:247–259
Donato D, Kauffman J, Murdiyarso D, Kurnianto S, Stidham M, Kanninen M (2011) Mangroves among the most carbon-rich forests in the tropics. Nat Geosci 4:293–297
Fengel D, Weneger G (1984) Wood: chemistry ultrastructure, reactions. Walter de Gruyter, Berlin, 560 pp
Giroux JF, Bedard J (1988) Estimating above and belowground macrophyte production in Scirpus tidal marshes. Can J Bot 66:368–374
Harter S, Mitsch W (2003) Patterns of short-term sedimentation in freshwater created marsh. J Environ Qual 32:325–334
Hatton R, DeLaune R, Patrick J (1983) Sedimentation, accretion, and subsidence in marshes of Barataria Basin, Louisiana. Limnol Oceanogr 28:494–502
Hernández ME, Mitsch WJ (2007) Denitrification potential and organic matter as affected by vegetation community, wetland age, and plant introduction in created wetlands. J Environ Qual 36:333–342
Hupp C, Demas C, Kroes D, Day R, Doyle T (2008) Recent sedimentation within the central Atchafalaya Basin, Louisiana. Wetlands 28:125–140
Infante D, Moreno-Casasola P, Madero-Vega C, Castillo-Campos G, Warner B (2011) Floristic composition and soil characteristics of tropical freshwater forested wetlands of Veracruz on the coastal plain of the Gulf of Mexico. For Ecol Manag 262:1514–1531
Infante D, Moreno-Casasola P, Madero-Vega C (2012) Litterfall of tropical forested wetlands of Veracruz in the coastal floodplains of the Gulf of Mexico. Aquat Bot 98:1–11
Intergovernmental Panel on Climate Change (IPCC) (2007) Fourth assessment report: synthesis report. IPCC, Geneva, p 2007
Jeffries R, Darby S, Sear D (2003) The influence of vegetation and organic debris on flood-plain sediment dynamics: case study of a low-order stream in the new New Forest, England. Geomorphology 51:61–80
Jobbágy E, Jackson R (2000) The vertical distribution of soil organic carbon and its relation to climate and vegetation. Belowground Process Global Change 10:423–436
Kayranli B, Scholz M, Mustafa A, Hedmark A (2010) Carbon storage and fluxes within freshwater wetlands: a critical review. Wetlands 30:111–124
Kauffman JB, Heider C, Cole TG, Dwire KA, Donato DC (2011) Ecosystem carbon stocks of Micronesian mangrove forests. Wetlands 31:343–352
Khan N, Suwa R, Hagihara (2007) A Carbon and nitrogen pool in a mangrove stand of Kandelia obovata (S., L.) Yong: vertical distribution in the soil–vegetation system. Wetl Ecol Manag 15:141–153
Kirschbaum MUF (1995) The temperature dependence of soil organic matter decomposition and the effect of global warming on soil organic carbon storage. Soil Biol Biochem 27:753–760
Kögel-Knabner (2002) The macromolecular organic composition of plant and microbial residues as input to soil organic matter. Soil Biol Biochem 34:139–162
Lewis W (2000) Wetlands: characteristics and boundaries, 2nd edn. National Academy of Sciences, USA
Loomis M, Craft C (2010) Carbon sequestration and nutrient (Nitrogen, phosphorus) accumulation in river-dominated tidal marshes, Georgia, USA. Soil Sci Soc Am J 74:1028–1036
Lorenz K, Preston CM, Raspe S, Morrison IK, Feger KH (2000) Litter decomposition and humus characteristics in Canadian and German ecosystems: information from tannin analysis and 13C CPMAS NMR. Soil Biol Biochem 32:779–792
Lot-Helgueras A (2004) Flora and vegetation of freshwater wetlands in the coastal zone of the Gulf of Mexico. In: Caso M, Pisanty I, Ezcurra E (eds) Environmental analysis of the Gulf of Mexico. SEMARNAT - Instituto Nacional de Ecología - Instituto de Ecología, A.C/Harte Research Institute for Gulf of Mexico Studies - Texas A&M University, Mexico, pp 314–339
Loucks OL (1990) Restoration of the pulse control function of wetlands and 658 its relationship to water quality objectives. In: Kusler JA, Kentula ME (eds) Wetland creation and restoration: the status of science. USEPA, Corvallis, pp 467–477
Mbah CN, Idike FI (2011) Carbon storage in tropical agricultural soils of south eastern Nigeria under different management practice. Int Res J Agric Sci Soil Sci 1:053–057
Middleton BA (2002) The flood pulse concept in wetland restoration. In: Middleton B (ed) Flood pulsing in wetlands: restoring the natural hydrological balance. John Wiley and Sons, New York, pp 1–10
Mitsch WJ, Taylor R, Benson K (1991) Estimating primary productivity of forested wetland communities in different hydrologic landscapes. Landsc Ecol 5:75–92
Mitsch WJ, Gosselink JG (2007) Wetlands, 4th edn. John Wiley & Sons, New York, 582 pp
Mitsch W, Nahlik A, Wolski P, Bernal B, Zhang L, Ramberg L (2010) Tropical wetlands: seasonal hydrologic pulsing, carbon sequestration, and methane emissions. Wetl Ecol Manag 18:573–686
Moreno E, Guerrero A, Gutiérrez M, Ortiz C, Palma D (2002) Los manglares de Tabasco, una reserva natural de carbono. Madera Bosques 8:115–128
Moreno-Casasola P, López H, Infante D, Peralta L, Travieso-Bello AC, Warner BG (2009) Environmental and anthropogenic factors associated with coastal wetland differentiation in La Mancha, Veracruz, México. Plant Ecol 200:37–52
Moreno-Casasola P, Cejudo-Espinosa E, Infante-Mata D, López-Rosas H, Castillo-Campos G, Pale-Pale J, Campos-Cascaredo A (2010) Composición florística, diversidad y ecología de humedales herbáceos emergentes en la planicie costera central de Veracruz, México. Bol Soc Bot Méx 87:29–50
Moreno-May GJ, Cerón-Bretón JG, Cerón-Bretón RM, Guerra-Santos JJ, Amador del Ángel LE, Endañú-Huerta E (2010) Estimación del potencial de captura de carbono en suelos de manglar de isla del Carmen. Unacar Tecnociencia 4:23–39
Neubauer SC, Miller WD, Anderson IC (2000) Carbon cycling in a tidal freshwater marsh ecosystem: a carbon gas flux study. Mar Ecol Prog Ser 199:13–31
Neue HU, Gaunt LL, Wang LP, Becker-Heidmann P, Quijano C (1997) Carbon in tropical wetlands. Geoderma 79:163–185
Noe G, Hupp C (2009) Retention of riverine sediment and nutrient loads by coastal plain floodplains. Ecosystems 12:728–746
Noe G, Hupp C (2005) Carbon, nitrogen, and phosphorus accumulation in floodplains of Atlantic coastal plain rivers, USA. Ecol Appl 15:1178–1190
Nyman J, Walters R, Delaune R, Patrick W Jr (2006) Marsh vertical accretion via vegetative growth. Estuar Coast Shelf Sci 69:370–380
Olmsted I (1993) Wetlands of Mexico. In: Whigham DF, Dykyjová D, Hejnÿ S (eds) Wetlands of the world I: inventory, ecology and management. Handbook of vegetation science. Kluwer Academic Publishers, Dordrcht, pp 637–678
Pant H, Rechcigl J, Adjei M (2003) Carbon sequestration in wetlands: concept and estimation. Food Agr Environ 1:308–313
Peralta-Peláez LA, Moreno-Casasola P (2009) Composición florística y diversidad de la vegetación de humedales en los lagos interdunarios de Veracruz. Bol Soc Bot Méx 85:89–101
Post WM, Emanuel WR, Zinke PJ, Stangenberger AG (1982) Soil carbon pools and world life zones. Nature 298:156–159
Post W, Izaurralde C, Jastrow J, McCarl B, Amonette J, Bailey V, Jardine P, West T, Zhou J (2004) Enhancement of carbon sequestration in US soils. BioScience 54:895–908
Richardson JL, Vepraskas MJ (2000) Wetland soils: genesis, hydrology, landscapes and classification. Lewis Publishers, Boca Raton
Sánchez-Carrillo S, Álvarez-Cobelas M, Angeler D (2001) Sedimentation in the semi-arid freshwater wetland Las Tablas de Daimiel (Spain). Wetlands 21:112–124
Schubauer JP, Hopkinson CS (1984) Above and belowground emergent macrophyte production and turnover in a coastal marsh ecosystem, Georgia. Limnol Oceonogr 29:1052–1065
Sharitz RR, Mitsch WJ (1993) Southern floodplain forests. In: Martin WH, Boyce SG, Echternacht AC (eds) Biodiversity of the Southeastern United States, Lowland Terrestrial Communities. John Wiley & Sons, New York, pp 311–372
SPSS (2009) Statistical package for the social sciences. Rel. 18.0 for windows XP. SPSS Inc, Chicago
Symbula M, Day Jr (1988) Evaluation of two methods for estimating belowground production in a freshwater swamp forest. Am Midl Nat 120:404–415
Tormann M, Bayley S (1997) Aboveground net primary production along a bog-fen-marsh gradient in southern boreal Alberta Canada. Ecoscience 4:374–384
Warner B, Aravena R, Moreno-Casasola P (2005) Cambio climático y reciclaje de carbono en los humedales costeros. In: Moreno-Casasola P, Peresbarsoba R, Travieso-Bello AC (eds) Estrategias para el manejo costero integral: el enfoque municipal. Instituto de Ecología, A. C. y Gobierno del Estado de Veracruz-Llave Xalapa, Ver, Mexico, pp 297–318
Wang H, Wang R, Yu Y, Mitchell M, Zhang L (2011) Soil organic carbon of degraded wetlands treated with freshwater in the yellow river delta, China. J Environ Manage 92:2628–2633
Wang S, Tian H, Liu J, Pan S (2003) Pattern and change of soil organic carbon storage in China: 1960–1980s. Tellus 55:416–427
Walkotten WJ (1976) An improved technique for freeze sampling streambed sediments. Forestry Research Technician. USDA For Service Research Note. PNW-281, 11 pp. Pac. Northwest For. and Range Exp. Stn, Portland
Yetter JC (2004) Hydrology and geochemistry of freshwater wetlands on the Gulf coast of Veracruz, Mexico. MS Thesis. University of Waterloo, Ontario, 168 pp
Acknowledgments
Funding for this work was provided by the Mexican National Council for Science and Technology -CONACYT- through the basic science project No. 081942, Sectorial Fund SEMARNAT-CONACYT Project No. 107887 and the PhD. scholarship No. 35192. The authors thank Alejandro Hernández, Lamberto Aragón, Ranulfo Castillo, Monserrat Vidal, J. Alejandro Marín and Carmelo Maximiliano for their help in the field work. We are grateful to the local guides who accompanied us throughout the field work: Tomas León Rodríguez and Eduardo Lauranchet.
Author information
Authors and Affiliations
Corresponding author
Additional information
Responsible Editor: Zucong Cai.
Electronic supplementary material
Below is the link to the electronic supplementary material.
ESM 1
(DOCX 251 kb)
Rights and permissions
About this article
Cite this article
Marín-Muñiz, J.L., Hernández, M.E. & Moreno-Casasola, P. Comparing soil carbon sequestration in coastal freshwater wetlands with various geomorphic features and plant communities in Veracruz, Mexico. Plant Soil 378, 189–203 (2014). https://doi.org/10.1007/s11104-013-2011-7
Received:
Accepted:
Published:
Issue Date:
DOI: https://doi.org/10.1007/s11104-013-2011-7