Abstract
Alzheimer’s disease (AD) is an age-related neurodegenerative disorder. The exact mechanism for the AD pathogenesis is not clearly understood. However, a number of hypotheses have been proposed to explain the pathogenesis of AD. One the hypotheses is the oxidative stress hypothesis that is supported by a number of studies which reported an increase in the levels of reactive oxygen/reactive nitrogen species and their products with a concomitant decrease in the levels of antioxidant enzymes in AD brain. In the present study, we measured in AD brain the expression levels of different forms (monomer, dimer and tetramer) of the pro-apoptotic protein, p53, and observed greater levels of p53 monomer and dimer in AD brain compared to control. In addition, we also showed the selective glutathionylation of monomeric and dimeric form of p53 in AD brain. We propose that glutathionylation of p53 may prevent the formation of tetramer, an aggregate form required for effective action of p53, and may be involved in oxidative stress conditions and neurodegeneration observed in this dementing disorder.
Similar content being viewed by others
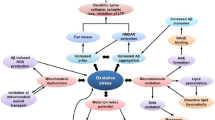
Avoid common mistakes on your manuscript.
Introduction
Alzheimer’s disease (AD) is an age-related neurodegenerative disorder characterized pathologically by senile plaques, neurofibrillary tangles (NFT), and brain atrophy [1]. Some of the major risk factors for AD are age, head injury, low intellectual levels [1], the presence of ApoE-4 alleles in both sporadic and familial AD (FAD) [2], and genetic factors, such as mutation in amyloid precursor protein (APP) and presenilin-1 in FAD [3] among others. The exact mechanism (s) responsible for AD pathogenesis is (are) not clearly understood, which limits the development of faithful animal models and the development of therapeutics to treat or delay the onset of AD. Large numbers of studies have proposed various hypotheses to explain the pathogenesis or progression of AD that include the amyloid hypothesis, oxidative stress hypothesis, APOE genotype, etc. [4–6]. Further, the use of vitamin E in cell culture diminished Aβ (1-42)-induced toxicity, which supports the role of oxidative damage in AD pathology [7, 8].
One of the most important antioxidants present in the brain is glutathione (GSH) [9–11]. GSH maintains the cellular redox balance depending upon the pH of the cellular compartment and is involved in various biosynthetic processes as well [12]. GSH act as a oxyradical scavenger by scavenging NO and other oxidants, thereby protecting the cells against oxidative damage by reducing oxidized or nitrosylated protein thiols [12–16]. In addition, GSH is involved in the elimination of toxic oxidation products such as 4-hydroxy-2-nonenal (HNE), a product of lipid peroxidation [10, 17]. In AD brain, the levels of GSH are reduced with a concomitant increase in glutathione disulfide (GSSG) levels [18, 19]. Normally the ratio of GSH to GSSG is maintained extensively towards the more reduced state, and any alteration in this ratio may induce reversible formation of mixed disulfides between protein sulfhydryl groups (PSH) and glutathione (S-glutathionylation) of a large number of proteins [10, 11]. Moreover, activation of several signaling pathways, including protein kinase B, calcineurin, nuclear factor κB, and mitogen-activated protein kinase, result from changes in redox status of neurons following oxidation of GSH [20].
S-glutathionylation is a reversible posttranslational modification from which the release of GSH can be catalyzed enzymatically by glutaredoxin, a thioltransferase [21–23]. The role of S-glutathionylation during oxidative stress has not been completely elucidated and several hypotheses have been proposed. Glutathionylation may represent a reversible way of protection from oxidation of cysteine residues of proteins, preventing permanent damage [10, 11, 24, 25]. Glutathionylation may also serve as a storage mechanism for glutathione inside the cell preventing its rapid extrusion from the cell [11, 26]. S-glutathionylation also occurs under basal conditions, suggesting its possible involvement in cellular signaling and redox regulation of protein functions [22, 27, 28].
p53 is a tumor-suppressor protein that plays an important role in maintaining genomic integrity [29] during the exposure of cells to radiation, genotoxic chemicals, hypoxia or oxidative stress [30, 31]. p53 protein has five domains: N-terminus, C-terminus, proline rich domain, DNA binding domain, and homo-oligomerization domain. The homo-oligomerization domain is essential for tetramerization of p53, a state that is important for DNA binding activity of p53, required for activation of the transcription of several genes involved in cell-cycle control, DNA repair, and apoptosis [32].
Recent studies from our laboratory showed increased expression of p53 in AD brain [30]. Further, p53 was shown to be oxidatively modified in brains from subjects with AD and arguably the earliest form of AD, mild cognitive impairment (MCI) [30, 31]. In the present study, we measured the amount of monomer, dimer and tetramer of p53 using Western blot analysis, and we determined the amount of glutathionylation of each form of p53 in control and AD inferior parietal lobule. The results suggest increased levels of monomeric and dimeric forms of p53, with no change in the level of tetrameric p53 in AD samples compared to that of control. Further, the level of glutathionylation of p53 is increased in monomer and dimeric forms of p53 in AD samples compared to controls.
Materials and Methods
Most chemicals used in this study were purchased from Sigma–Aldrich (St. Louis, MO, USA), while nitrocellulose membranes and the electrophoretic transfer system trans-blot semi-dry transfer cell were purchased from Bio-Rad (Hercules, CA, USA). Anti-p53 monoclonal antibody used for immunoprecipitation and Western blotting was obtained from Calbiochem (LA Jolla, CA, USA), while anti-GSH mouse monocolonal antibody was purchased from Virogen (Watertown, MA, USA). An anti-mouse IgG alkaline phosphatase secondary antibody was obtained from Chemicon International (Temulca, CA, USA).
Subjects
Frozen inferior parietal lobule (IPL) samples from AD and age-matched controls were obtained from the University of Kentucky rapid autopsy program of the Alzheimer’s disease clinical center (UK ADC). The diagnosis of probable AD was made according to criteria developed by the National Institute of Neurological and Communicative Disorders and Stroke (NINCDS) and the Alzheimer’s disease and Related Disorders Association (ADRDA) [33]. All AD patients displayed progressive intellectual decline. Control subjects were without history of dementia or other neurological disorders and underwent annual mental status testing and semi-annual physical and neurological exams as part of the UK ADC normal volunteer longitudinal aging study. Samples and demographics used for the AD study were described previously [34]. Of note, the post mortem interval was approximately 3 h for both control and AD subjects.
Sample Preparation
The brain tissues (IPL) from control and AD were sonicated in lysis buffer (pH 7.4) containing 10 mM HEPES buffer, 137 mM NaCl, 4.6 mM KCl, 1.1 mM KH2PO4, and 0.6 mM MgSO4, as well as proteinase inhibitors leupeptin (0.5 mg/ml), pepstatin (0.7 mg/ml), type II S soybean trypsin inhibitor (0.5 mg/ml), and PMSF (40 mg/ml). Homogenates were centrifuged at 14,000×g for 10 min to remove debris [35]. Protein concentration in the supernatant was determined by the Pierce BCA method (Pierce, Rockford, IL, USA).
Immunoprecipitation Analysis
For immunoprecipitation of glutathione-conjugated p53 and to study the p53 isoforms levels in controls and AD IPL, 150 μg of protein extracts were dissolved in 500 μl of RIPA buffer (10 mM Tris, pH 7.6; 140 mM NaCl; 0.5% NP40 including protease inhibitors) and then incubated with 1 μg of the conformation-specific antibody PAb1620 (wild-type specific) at 4°C overnight. Immunocomplexes were collected by using protein A/G suspension for 2 h at 4°C and washed five times with immunoprecipitation buffer. Immunoprecipitated p53 was recovered by resuspending the pellets in non-reducing SDS buffers and subjected to electrophoresis on 10% gels followed by Western blot analysis using as a primary antibody the polyclonal anti-p53 antibody CM1 at a 1:1,000 dilution or monoclonal anti-GSH antibody at a 1:1,000 dilution for 4 h at room temperature. After three washes for 5 min with wash blot, the membranes were incubated for 1 h at room temperature with an anti-rabbit or anti-mouse IgG alkaline phosphatase secondary antibodies, respectively, diluted 1:2,000 in wash blot and developed using 5-bromo-4-chloro-3-indolyl-phosphate/nitroblue tetrazolium (BCIP/NBT) color developing reagent. Blots were dried and scanned with Adobe Photoshop and quantitated with Scion Image (PC version of Macintosh-compatible NIH Image) software.
Gel Staining
To measure p53 levels the gels were fixed in a solution containing 10% (v/v) methanol and 7% (v/v) acetic acid for 20 min and then stained overnight at room temperature with agitation in 50 ml Sypro Ruby gel stain (Bio-Rad, Hercules, CA, USA). The gels were scanned with a UV transilluminator (excitation wavelength: 470 nm, emission wavelength: 618 nm; Molecular Dynamics, Sunnyvale, CA), and quantitated with Scion Image (PC version of Macintosh-compatible NIH Image) software.
Statistics
All statistical analysis was performed using a two-tailed Student’s t-test. P < 0.05 was considered significantly different from control.
Results
Monomer, Dimer and Tetramer Forms of p53 Protein in AD IPL
In the present study the protein extracts from controls and AD IPL were immunoprecipitated with a conformational specific antibody against p53 (wild type specific Ab 1620) and then subjected to Western blotting analysis in non-denaturing conditions to preserve p53-GSH interaction. Fig. 1 (a, b) shows a 2-fold increase of p53 monomer (P < 0.04), and 1.2-fold (P < 0.02) increase of p53 dimer, while there was no change in the level of tetrameric p53 in AD samples compared to that of controls.
a Representative gel of the levels of p53 in AD IPL and age-matched control (CTR) IPL. 150 μg of proteins from N = 6 AD and CTR samples were immunoprecipitated with p53 antibody and then electrophoresed using non-reducing SDS–PAGE. Gel was fixed and stained using Sypro Ruby. b The densitometric analyses of the levels of the three forms of p53 in AD IPL and CTR IPL specimens are presented. The control value was set to 100%, to which experimental values were compared. Ba: tetrameric form CTR = 100 ± 14%, AD = 95 ± 17%. Bb: dimeric form CTR = 100 ± 12.6%, AD = 119.3 ± 5.5% p < 0.02. Bc: monomeric form CTR = 100 ± 33.3%, AD = 196 ± 12% p < 0.04
Glutathionylation Levels in p53 Protein in AD IPL
As is showed in the Fig. 2 (a, b) we found that GSH reacts with three forms of p53, the monomeric form, the dimeric form, and the tetrameric form. Our data show that in AD brain compared with control, the increase in glutathionylation is about 1.3-fold for the dimeric form and about 1.7-fold for the monomeric form, with both values being statistically significant (P < 0.05). Tetrameric p53 was not differentially bound by GSH in AD vs. control.
a Representative blot of the amount of p53 S-glutathionylation in AD IPL and CTR IPL age matched sample. 150 μg of proteins from N = 6 AD and CTR samples were immunoprecipitated with p53 antibody and then electrophoresed using non-reducing SDS–PAGE. Proteins were transferred to nitrocellulose membranes and probed with the primary monoclonal anti-GSH antibody. b The densitometric analyses of S-glutathionylation of the three forms of p53 in AD IPL and CTR IPL specimens are shown
Discussion
Oxidative stress has been demonstrated in the IPL of AD brain as indexed by an increased number of oxidized proteins. The proteomics-identified oxidatively modified and dysfunctional brain proteins by our laboratory were reported to be involved in various cellular functions such as energy metabolism, mitochondrial function, cell cycle, synaptic plasticity, excitotoxicity, proteasomal dysfunction, lipid abnormalities, neuritic abnormalities, tau hyperphosphorylation, etc. [34, 36–39]. The oxidative modification and dysfunction of these proteins could lead to altered cell functions and eventually lead to neuronal death [40–42], plausibly providing mechanism(s) for neurodegeneration observed in the AD brain.
A majority of redox-sensitive proteins have one or more cysteines that exist as thiolate anions, also called reactive cysteines, which play a crucial role in redox signaling [43]. The reactive cysteines are more nucleophilic and, therefore, are highly susceptible to attack by reactive oxygen and reactive nitrogen species (ROS and RNS, respectively) [44]. Considerable evidence showed the involvement of cysteines in the redox modulation and DNA binding function of p53 [45–47]. Human p53 has ten cysteine residues localized in its DNA binding domain, between amino acids 100 and 300 [45]. These cysteines are really important to stabilize the tertiary structure of p53 core domain and consequently its functionality.
p53 binds the DNA as a symmetric tetramer consisting of two head-to-head dimers, with each dimer occupying a half-site of sequence target [48]. Although a single dimer is sufficient for DNA binding, the interaction is enhanced (>50-fold) by the interaction of second dimer that also stabilizes the tetramer [48, 49]. In native condition p53 is localized in the cytoplasm as a monomer, and it is unable to bind DNA.
In the present study we demonstrated significantly increased levels of p53 dimeric and monomeric forms in AD IPL compared to control samples, but not of p53 tetrameric form. Recent work from our laboratory showed that the total expression of p53 in AD and MCI IPL compared to control was significantly increased [30]. The data of the present report confirm these previous results, and further suggest that the increase in the p53 levels mainly is due to increased levels of monomeric and dimeric forms of p53. Further, we also found that GSH binds to three different structures of p53, the tetramer, the dimer and the monomer. The most abundant S-glutathionylated form is the tetrameric form that is observed in both the control and AD sample. However, an overall increase in the levels of glutathionylation was observed only for monomeric and dimeric forms. This increase in glutathionylation of monomeric and dimeric forms, if it occurs in the DNA-binding domain of p53, may prevent the formation of tetramer, thereby preventing the interaction of p53 with DNA [45, 46].
Because glutathionylation of p53 may prevent the formation of p53 tetramer, this may cause a negative regulation of p53 tetramerization and probably the binding to DNA. Interestingly, a recent study, employing theoretical considerations, hypothesized the Cys182 of p53 could be a target of glutathionylation, and this would inhibit the tetramerization of p53 [46]. Gluthionayltion may be considered as a protective mechanism for critical and conserved cysteine residues against irreversible oxidation [11]. In the case of p53, glutathionylation also can represent a way to protect the integrity of its sulfhydryl groups from irreversible modification that occurs in the progression of AD, leading to permanent inactivation and degradation of the protein. S-glutathionylated p53 conceivably may represent a small pool of inactive protein, which could become useful after the reversion of GSH binding, in response to a critical situation [47]. Another interesting speculation that can be made about the p53 glutathionylation is the suppression of the apoptotic signals generated in stressed cells [47] as an adaptation strategy. This defensive response may facilitate a reprogramming of gene expression for cytoprotection and/or prevent cell death [50, 51]. S-glutathionylation of p53 may be considered a redox-dependent posttranslational modification with potential relevance to signal transduction.
Further, monomeric and dimeric forms of p53 might have the reactive cysteines of the core domain of p53 exposed to the external environment, which would make them more susceptible to oxidative stress attack and consequently to GSH conjugation. On the contrary, in the tetrameric isoform, the reactive cysteines perhaps are hidden inside the quaternary structure, and hence may not be accessible to GSH. Further studies are required to identify the specific cysteine residue(s) that is (are) glutathionylated. A previous study reported that glutathionylation could occur on Cys124 or Cys141 in a single p53 molecule, but not together due to their closeness and the resulting steric hindrance. The energy-minimized model of S-glutathionated p53 suggested that the modification would result in retraction of the recognition loop from DNA, and a dimer interface would not form [47].
The sensitivity of p53 to oxidative and nitrosative stress was reported in our previous studies [30, 31]. Further, several studies on cell lines culture suggested that p53 is highly prone to oxidative inactivation. For example, genes targeted for transactivation by p53 in human cells are affected by pharmacological oxidizing and reducing agents [52]. Hypoxia and nitric oxide induce inactivation of p53 [53, 54]. The functions of p53 are also sensitive to metal cations and Cu2+/Cu+ redox cycling [55].
A recent proteomics study from our laboratory identified a number of proteins that are S-glutathionylated in AD brain, which are involved in different cellular processes such as glucose metabolism or energy metabolism showing partial loss of their functions. These results indicate the involvement of S-glutathionylation in AD brain, suggesting a crucial role for glutathionylation during the progression of the disease [56].
In summary, the present study for the first time showed the glutathionylation of p53 in AD brain is elevated specifically on the monomeric and dimeric forms of p53, suggesting that post-translational modification of p53 could be involved in neurodegeneration and in oxidative stress conditions. Additional investigations are in progress in our laboratory to study the ability of p53 to bind the DNA-sequence targets and its activity under oxidative stress in neurodegenerative diseases such as Alzheimer disease and after S-glutathionylation.
References
Katzman R, Saitoh T (1991) Advances in Alzheimer’s disease. FASEB J 5:278–286
Namba Y, Tomonaga M, Kawasaki H, Otomo E, Ikeda K (1991) Apolipoprotein E immunoreactivity in cerebral amyloid deposits and neurofibrillary tangles in Alzheimer’s disease and kuru plaque amyloid in Creutzfeldt–Jakob disease. Brain Res 541:163–166
Lee MK, Borchelt DR, Kim G, Thinakaran G, Slunt HH, Ratovitski T, Martin LJ, Kittur A, Gandy S, Levey AI, Jenkins N, Copeland N, Price DL, Sisodia SS (1997) Hyper accumulation of FAD-linked presenilin 1 variants in vivo. Nat Med 3:756–760
Butterfield DA (2002) Amyloid beta-peptide (1-42)-induced oxidative stress and neurotoxicity: implications for neurodegeneration in Alzheimer’s disease brain. A review. Free Radic Res 36:1307–1313
Butterfield DA, Reed T, Newman SF, Sultana R (2007) Roles of amyloid beta-peptide-associated oxidative stress and brain protein modifications in the pathogenesis of Alzheimer’s disease and mild cognitive impairment. Free Radic Biol Med 43:658–677
Huebbe P, Schaffer S, Jofre-Monseny L, Boesch-Saadatmandi C, Minihane AM, Muller WE, Eckert GP, Rimbach G (2007) Apolipoprotein E genotype and alpha-tocopherol modulate amyloid precursor protein metabolism and cell cycle regulation. Mol Nutr Food Res 51:1510–1517
Yatin SM, Aksenov M, Butterfield DA (1999) The antioxidant vitamin E modulates amyloid beta-peptide-induced creatine kinase activity inhibition and increased protein oxidation: implications for the free radical hypothesis of Alzheimer’s disease. Neurochem Res 24:427–435
Boyd-Kimball D, Mohmmad Abdul H, Reed T, Sultana R, Butterfield DA (2004) Role of phenylalanine 20 in Alzheimer’s amyloid beta-peptide (1-42)-induced oxidative stress and neurotoxicity. Chem Res Toxicol 17:1743–1749
Cooper J (1997) In: Barchi R, Kunk L (eds) Glutathione in the brain: disorders of glutathione metabolism. Butterworth–Heinemann, Boston
Klatt P, Lamas S (2000) Regulation of protein function by S-glutathiolation in response to oxidative and nitrosative stress. Eur J Biochem 267:4928–4944
Dalle-Donne I, Rossi R, Giustarini D, Colombo R, Milzani A (2007) S-glutathionylation in protein redox regulation. Free Radic Biol Med 43:883–898
Schulz JB, Lindenau J, Seyfried J, Dichgans J (2000) Glutathione, oxidative stress and neurodegeneration. Eur J Biochem 267:4904–4911
Meister A (1995) Mitochondrial changes associated with glutathione deficiency. Biochim Biophys Acta 1271:35–42
Darley-Usmar V, Halliwell B (1996) Blood radicals: reactive nitrogen species, reactive oxygen species, transition metal ions, and the vascular system. Pharm Res 13:649–662
Sies H (1999) Glutathione and its role in cellular functions. Free Radic Biol Med 27:916–921
Hansen JM, Go YM, Jones DP (2006) Nuclear and mitochondrial compartmentation of oxidative stress and redox signaling. Annu Rev Pharmacol Toxicol 46:215–234
Watson WH, Chen Y, Jones DP (2003) Redox state of glutathione and thioredoxin in differentiation and apoptosis. Biofactors 17:307–314
Owen AD, Schapira AH, Jenner P, Marsden CD (1997) Indices of oxidative stress in Parkinson’s disease, Alzheimer’s disease and dementia with Lewy bodies. J Neural Transm Suppl 51:167–173
Aksenov MY, Markesbery WR (2001) Changes in thiol content and expression of glutathione redox system genes in the hippocampus and cerebellum in Alzheimer’s disease. Neurosci Lett 302:141–145
Wu G, Fang YZ, Yang S, Lupton JR, Turner ND (2004) Glutathione metabolism and its implications for health. J Nutr 134:489–492
Chrestensen CA, Starke DW, Mieyal JJ (2000) Acute cadmium exposure inactivates thioltransferase (Glutaredoxin), inhibits intracellular reduction of protein-glutathionyl-mixed disulfides, and initiates apoptosis. J Biol Chem 275:26556–26565
Cotgreave IA, Gerdes R, Schuppe-Koistinen I, Lind C (2002) S-glutathionylation of glyceraldehyde-3-phosphate dehydrogenase: role of thiol oxidation and catalysis by glutaredoxin. Methods Enzymol 348:175–182
Shenton D, Perrone G, Quinn KA, Dawes IW, Grant CM (2002) Regulation of protein S-thiolation by glutaredoxin 5 in the yeast Saccharomyces cerevisiae. J Biol Chem 277:16853–16859
Schafer FQ, Buettner GR (2001) Redox environment of the cell as viewed through the redox state of the glutathione disulfide/glutathione couple. Free Radic Biol Med 30:1191–1212
Jacob C, Knight I, Winyard PG (2006) Aspects of the biological redox chemistry of cysteine: from simple redox responses to sophisticated signalling pathways. Biol Chem 387:1385–1397
Sies H, Akerboom TP (1984) Glutathione disulfide (GSSG) efflux from cells and tissues. Methods Enzymol 105:445–451
Chai YC, Hoppe G, Sears J (2003) Reversal of protein S-glutathiolation by glutaredoxin in the retinal pigment epithelium. Exp Eye Res 76:155–159
Reynaert NL, Ckless K, Guala AS, Wouters EF, van der Vliet A, Janssen-Heininger YM (2006) In situ detection of S-glutathionylated proteins following glutaredoxin-1 catalyzed cysteine derivatization. Biochim Biophys Acta 1760:380–387
Ko LJ, Prives C (1996) p53: puzzle and paradigm. Genes Dev 10:1054–1072
Cenini G, Sultana R, Memo M. and Butterfield DA (2007) Elevated levels of Pro-apoptotic p53 and its oxidative modification by the lipid peroxidation product, HNE, in brain from subjects with amnestic mild cognitive impairment and Alzheimer’s disease. J Cell Mol Med. doi:10.1111/j.1582-4934.2007.00163.x
Cenini G, Sultana R, Memo M. and Butterfield DA (2008) Effect of oxidative and nitrosative stress in brain on p53 proapoptotic protein in amnestic mild cognitive impairment and Alzheimer disease. Free Radic Biol Med. doi:10.1016/j.freeradbiomed.2008.03.015
Almog N, Rotter V (1997) Involvement of p53 in cell differentiation and development. Biochim Biophys Acta 1333:F1–F27
McKhann G, Drachman D, Folstein M, Katzman R, Price D, Stadlan EM (1984) Clinical diagnosis of Alzheimer’s disease: report of the NINCDS–ADRDA work group under the auspices of Department of Health and Human Services Task Force on Alzheimer’s disease. Neurology 34:939–944
Sultana R, Boyd-Kimball D, Poon HF, Cai J, Pierce WM, Klein JB, Merchant M, Markesbery WR, Butterfield DA (2006) Redox proteomics identification of oxidized proteins in Alzheimer’s disease hippocampus and cerebellum: an approach to understand pathological and biochemical alterations in AD. Neurobiol Aging 27:1564–1576
Butterfield DA, Sultana R (2007) Redox proteomics identification of oxidatively modified brain proteins in Alzheimer’s disease and mild cognitive impairment: insights into the progression of this dementing disorder. J Alzheimers Dis 12:61–72
Castegna A, Aksenov M, Aksenova M, Thongboonkerd V, Klein JB, Pierce WM, Booze R, Markesbery WR, Butterfield DA (2002) Proteomic identification of oxidatively modified proteins in Alzheimer’s disease brain. Part I: creatine kinase BB, glutamine synthase, and ubiquitin carboxy-terminal hydrolase L-1. Free Radic Biol Med 33:562–571
Castegna A, Aksenov M, Thongboonkerd V, Klein JB, Pierce WM, Booze R, Markesbery WR, Butterfield DA (2002) Proteomic identification of oxidatively modified proteins in Alzheimer’s disease brain. Part II: dihydropyrimidinase-related protein 2, alpha-enolase and heat shock cognate 71. J Neurochem 82:1524–1532
Butterfield DA, Boyd-Kimball D (2004) Proteomics analysis in Alzheimer’s disease: new insights into mechanisms of neurodegeneration. Int Rev Neurobiol 61:159–188
Sultana R, Boyd-Kimball D, Poon HF, Cai J, Pierce WM, Klein JB, Markesbery WR, Zhou XZ, Lu KP, Butterfield DA (2006) Oxidative modification and down-regulation of Pin1 in Alzheimer’s disease hippocampus: a redox proteomics analysis. Neurobiol Aging 27:918–925
Hensley K, Hall N, Subramaniam R, Cole P, Harris M, Aksenov M, Aksenova M, Gabbita SP, Wu JF, Carney JM et al (1995) Brain regional correspondence between Alzheimer’s disease histopathology and biomarkers of protein oxidation. J Neurochem 65:2146–2156
Lauderback CM, Hackett JM, Huang FF, Keller JN, Szweda LI, Markesbery WR, Butterfield DA (2001) The glial glutamate transporter, GLT-1, is oxidatively modified by 4-hydroxy-2-nonenal in the Alzheimer’s disease brain: the role of A beta 1-42. J Neurochem 78:413–416
Butterfield DA (2004) Proteomics: a new approach to investigate oxidative stress in Alzheimer’s disease brain. Brain Res 1000:1–7
Claiborne A, Mallett TC, Yeh JI, Luba J, Parsonage D (2001) Structural, redox, and mechanistic parameters for cysteine–sulfenic acid function in catalysis and regulation. Adv Protein Chem 58:215–276
Claiborne A, Yeh JI, Mallett TC, Luba J, Crane EJ 3rd, Charrier V, Parsonage D (1999) Protein-sulfenic acids: diverse roles for an unlikely player in enzyme catalysis and redox regulation. Biochemistry 38:15407–15416
Rainwater R, Parks D, Anderson ME, Tegtmeyer P, Mann K (1995) Role of cysteine residues in regulation of p53 function. Mol Cell Biol 15:3892–3903
Sun XZ, Vinci C, Makmura L, Han S, Tran D, Nguyen J, Hamann M, Grazziani S, Sheppard S, Gutova M, Zhou F, Thomas J, Momand J (2003) Formation of disulfide bond in p53 correlates with inhibition of DNA binding and tetramerization. Antioxid Redox Signal 5:655–665
Velu CS, Niture SK, Doneanu CE, Pattabiraman N, Srivenugopal KS (2007) Human p53 is inhibited by glutathionylation of cysteines present in the proximal DNA-binding domain during oxidative stress. Biochemistry 46:7765–7780
McLure KG, Lee PW (1998) How p53 binds DNA as a tetramer. EMBO J 17:3342–3350
Delphin C, Cahen P, Lawrence JJ, Baudier J (1994) Characterization of baculovirus recombinant wild-type p53. Dimerization of p53 is required for high-affinity DNA binding and cysteine oxidation inhibits p53 DNA binding. Eur J Biochem 223:683–692
Dandrea T, Bajak E, Warngard L, Cotgreave IA (2002) Protein S-glutathionylation correlates to selective stress gene expression and cytoprotection. Arch Biochem Biophys 406:241–252
Winyard PG, Moody CJ, Jacob C (2005) Oxidative activation of antioxidant defence. Trends Biochem Sci 30:453–461
Verhaegh GW, Richard MJ, Hainaut P (1997) Regulation of p53 by metal ions and by antioxidants: dithiocarbamate down-regulates p53 DNA-binding activity by increasing the intracellular level of copper. Mol Cell Biol 17:5699–5706
Cobbs CS, Whisenhunt TR, Wesemann DR, Harkins LE, Van Meir EG, Samanta M (2003) Inactivation of wild-type p53 protein function by reactive oxygen and nitrogen species in malignant glioma cells. Cancer Res 63:8670–8673
Hammond EM, Giaccia AJ (2005) The role of p53 in hypoxia-induced apoptosis. Biochem Biophys Res Commun 331:718–725
Wu HH, Thomas JA, Momand J (2000) p53 protein oxidation in cultured cells in response to pyrrolidine dithiocarbamate: a novel method for relating the amount of p53 oxidation in vivo to the regulation of p53-responsive genes. Biochem J 351:87–93
Newman SF, Sultana R, Perluigi M, Coccia R, Cai J, Pierce WM, Klein JB, Turner DM, Butterfield DA (2007) An increase in S-glutathionylated proteins in the Alzheimer’s disease inferior parietal lobule, a proteomics approach. J Neurosci Res 85:1506–1514
Acknowledgments
This research was supported in part by NIH grants to D.A.B. [AG-05119;AG-10836; AG-029839]. We thank the faculty of the UK ADC Neuropathology Core for providing the AD and control brain specimens used in this study.
Author information
Authors and Affiliations
Corresponding author
Additional information
Special issue article in Honour of Dr. Akitane Mori.
Fabio Di Domenico and Giovanna Cenini contributed equally.
An erratum to this article can be found at http://dx.doi.org/10.1007/s11064-009-9955-2
Rights and permissions
About this article
Cite this article
Domenico, F.D., Cenini, G., Sultana, R. et al. Glutathionylation of the Pro-apoptotic Protein p53 in Alzheimer’s Disease Brain: Implications for AD Pathogenesis. Neurochem Res 34, 727–733 (2009). https://doi.org/10.1007/s11064-009-9924-9
Accepted:
Published:
Issue Date:
DOI: https://doi.org/10.1007/s11064-009-9924-9