Abstract
Osteoporosis is characterized by a broken balance between bone formation and bone resorption. Mechanical stress has been considered to be an important factor in bone modeling and remodeling. However, biological responses of stromal cells in osteoporosis to mechanical stimuli remain unknown. To explore the correlation between mechanical stress and osteoblastic differentiation of bone mesenchymal stem cells (BMSCs) in osteoporosis, we built an osteoporosis model in ovariectomized (OVX) rats, and then investigated proliferation, alkaline phosphatase (ALP) activity, and the expression of osteoblastic genes in BMSCs under mechanical stress of 5 and 10 % elongation, using the Flexercell Strain system. The proliferation of BMSCs was detected using alamarBlue. The expression of osteoblastic genes was analyzed by real-time quantitative polymerase chain reaction. Protein expression was examined by Western blotting. BMSCs (OVX) and BMSCs (Sham-operated, Sham in short) proliferations were inhibited at 5 and 10 % elongation at day 3, compared with the un-stretched group, while BMSCs (OVX) proliferation was slower than BMSCs (Sham). ALP activity increased significantly at 10 % elongation in both cells, but it was less active in BMSCs (OVX) than BMSCs (Sham). At days 3 and 7, the mRNA expression of osteoblastic genes was unregulated by mechanical stretch (5 and 10 % elongation); however, osteoblastic gene expression in BMSCs (OVX) was less than that in BMSCs (Sham). The mRNA and protein expression of Runx2 showed similar trends in BMSCs (OVX) under mechanical stretch. These results indicate that the mechanical stretch stimulates osteoblastic differentiation of BMSCs (OVX); however, this differentiation was weaker than that of BMSCs (Sham).
Similar content being viewed by others
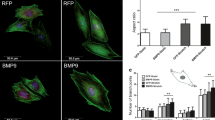
Avoid common mistakes on your manuscript.
Introduction
Osteoporosis is a serious worldwide disease, characterized by decreased bone mass and a progressive erosion of the microstructure. When such disease occurs, osteoblast activity reduces while osteoclast activity increases. As a result, the balance between bone formation and bone desorption is broken, leading to a loss of bone mass and fractures. Osteoporosis may be caused by several conditions, such as hormonal imbalances, chronic diseases, medications, or prolonged gravity-free conditions.
Estrogen plays a fundamental role in skeletal growth and bone homeostasis. The deficiency of Estrogen leads to a chronic inflammatory state because of an increase of the local production of various cytokines [1, 2]. The increased cytokines will then result in an expansion of the osteoclastic pool due to increased osteoclast formation [3].
Current treatments for osteoporosis typically involve pharmacological, hormonal, and mechanical strain interventions. All of these treatments aim at preventing osteoclastic bone resorption so as to maintain the bone mass and reduce fractures [3, 4]. However, hormonal and pharmacological interventions are often associated with adverse side effects, such as gastrointestinal intolerance with bisphosphonates, increased risks of breast, ovarian, and endometrial cancer [5, 6]. Meanwhile, they target the skeleton as a whole instead of targeting skeletal sites specifically, which increases the risk of failure. In contrast, mechanical strain intervention is non-invasive and has demonstrated promising results [7].
Mechanical loading is known to be an important factor in regulating bone growth and development, as well as maintaining the integrity of bone structure and function. It has been reported that physical exercise can improve bone mass, increase bone strength and elasticity, delay the progress of osteoporosis, and prevent osteoporotic bone loss and fractures [8–10]. It is also evidenced that a repeated application of shear stress can stimulate late phenotypic markers of osteoblastic differentiation in bone mesenchymal stem cells (BMSCs) [11–13]; bone is formed through BMSCs proliferation and differentiation into mature osteoblasts [14, 15]. However, little is known about the influence of mechanical stretch on the proliferation and osteoblastic differentiation of osteoporosis BMSCs.
To investigate the correlation between mechanical stretch and osteoblastic differentiation of osteoporosis BMSCs, we established a rat model of osteoporosis employing ovariectomy to mimic the conditions in postmenopausal women. Accordingly, ovariectomy has been demonstrated to elicit bone loss and increase bone turnover [16–19]. The ovariectomized (OVX) rat model of osteoporosis is thus considered to be appropriate for mimicking the conditions in postmenopausal women and has been widely used to evaluate potential therapeutics intended to prevent or treat osteoporosis [20].
In this study, we investigated the effects of various magnitudes of mechanical stretch on BMSCs (OVX) and BMSCs (Sham). In particular, we examined the cellular proliferation, mRNA levels of osteoblast-related genes, and ALP activity under different degrees of mechanical stretch.
Materials and methods
Animal and cell culture
The study was conducted in accordance with the regional Ethics Committee guidelines. Sixty female Sprague–Dawley rats, 3 months of age and weighing 220 ± 20 g, were obtained from Shanghai SLAC Experimental Animal Center (Shanghai, China). The animals underwent surgical ovariectomy (OVX group; n = 30) under anesthesia using chloral hydrate (Shenggong, Shanghai, China; 0.04 mL/kg body weight, intraperitoneally) or were sham-operated (Sham group, control, n = 30). The rats were then housed in a temperature-controlled room (21 °C) with the relative humidity at 60 % and a 12/12-h light/dark cycle.
Then, 12 weeks later, the rats of both groups were sacrificed. The humerus was isolated from the OVX and Sham rats. The bone marrow was flushed out with α-minimal essential medium (α-MEM, Hyclone, Thermo, USA), supplemented with 100 unit/mL penicillin, and 100 μg/mL streptomycin (Hyclone, Thermo, USA). To remove blood cells, whole washouts were collected and centrifuged (1800×g, 10 min). Next, the pellet was mixed with complete α-MEM supplemented with 10 % fetal bovine serum (FBS, Hyclone, Thermo, USA), 100 unit/mL penicillin, and 100 μg/mL streptomycin and then plated in a culture flask and maintained at 37 °C in 5 % CO2. Non-adherent cells were removed by changing the medium every 3 days for approximately 10 days. When large colonies formed and became confluent, the primary rat BMSCs were trypsinized with 10 % trypsin–EDTA (Hyclone, Thermo, USA) and passaged. rBMSCs from passages 2 to 5 were used for our experiments.
Mechanical tension load application
BMSCs (OVX) and BMSCs (Sham) were plated at a density of 1 × 105 cells/mL (unless stated otherwise) in 2 mL of medium on six-well flexible silicone rubber BioFlex™ plates coated with collagen type I (Flexcell International Corporation, Hillsborough, NC). The cells were cultured for 48 h to allow them to attach and reach 80–90 % confluence, at which time the growth medium was replaced, and then mechanical strain was applied. The cyclic mechanical strain with a 0.5 Hz sinusoidal curve set at 5 and 10 % elongation was separately applied twice every day, for 4 h for each treatment, using a FX-4000T™ Flexcell Tension PlusTM unit (Flexcell International Corporation, Hillsborough, NC). The cultures were incubated in a humidified atmosphere at 37 °C and 5 % CO2 while stretching. The BMSCs (OVX) and BMSCs (Sham) were harvested after 3 and 7 days of stretch stimulation.
Cell proliferation assay
For the cell proliferation assay, BMSCs were seeded on six-well flexible silicone rubber BioFlex™ Plates and allowed to reach about 80 % confluency, followed by intermittent traction stress treatment. According to the manufacturer’s instructions, cell viability and proliferation were assessed by alamarBlue assay (Invitrogen, Carlsbad, CA). The cells were incubated in medium supplemented with 10 % (v/v) alamar Blue fluorescent dye for 2 h, at time point 0, 1, 3, 5, and 7 days, respectively, upon intermittent traction stress at 37 °C and 5 % CO2.
Then a 200 μl sample of the medium was transferred and the absorbance at 570 nm measured in a 96-well plate (Corning, Corning, NY) using a Multiscan UV visible spectrophotometer (TECAN, Mannedorf, Switzerland). Nonseeded BioFlex™ plate with the same medium was used as blanks. Cells at baseline prior to stress loading served as control.
Cytoskeleton staining
The F-actin cytoskeleton in BMSCs (OVX) and BMSCs (Sham) was checked by staining. BMSCs (OVX) and BMSCs (Sham) were plated at the density of 1 × 105 cells/mL in 2 mL of medium on six-well flexible silicone rubber BioFlex™ plates. After cells attaching to the plates, mechanical tension was applied. After being subjected to mechanical stretching for 3 days, BMSCs (OVX) and BMSCs (Sham) were fixed with 4 % paraformaldehyde for 30 min, washed with PBS twice, then permeabilized with 0.1 % Triton X-100 for 5 min, and blocked with 1 % BSA for 30 min. Texas Red-X phalloidin at 210 nm (Invitrogen, Carlsbad, CA) was used to incubate with cells for 1 h at room temperature, washed with PBS three times. Then Hoechst (Invitrogen, Carlsbad, CA) at 1:1000 was used to incubate with cells for 5 min at room temperature, washed with PBS three times. Cells were visualized with a confocal microscope (LEICA TCSSP5, Wetzlar, Germany).
ALP staining
The presence of ALP in the cell layers was assessed according to the manufacturer’s instructions (Beyotime, Suzhou, China). The BMSCs (OVX) and BMSCs (Sham) from the control and stretch treatment groups were rinsed with PBS three times and fixed with 4 % paraformaldehyde for 15 min. The fixed cells were soaked in 0.1 % naphthol AS-MX phosphate and 0.1 % fast red violet LB salt in 56 mM 2-amino-2-methyl-1,3-propanediol for 45 min at 37 °C, washed with ddH2O, and then observed with a digital camera (ECLIPSETS 100, NIKON, Japan).
Real-time PCR
The total RNA of the cells was isolated using the Trizol reagent (Invitrogen, Carlsbad, CA), according to the manufacturer’s recommended protocol. The RNA concentrations were determined using a NanoDrop spectrophotometer (Thermo Scientific, Wilmington, DE). Complimentary DNA (cDNA) was synthesized by means of a cDNA Synthesis Reverse Transcription Kit (Fermentas, Thermo Scientific, Wilmington, DE). Real-time PCR was performed using a Light-Cycler system with SYBR Premix Ex TaqTM (Takara, Dalian, China), according to the manufacturer’s instructions. The conditions of the real-time PCR were as follows: denaturation at 95 °C for 10 s; 50 cycles at 95 °C for 10 s and 60 °C for 30 s; and a final dissociation stage (95 °C for 5 min) was added at the end of the amplification procedure. β-actin was used as an internal control. The data were analyzed using the comparative C t (2-ΔΔC t) method and expressed as a fold change respective to the control. Each sample was analyzed in triplicate. The primer sequences used in this study are listed in Table 1.
Western blotting
The cells were lysed on ice for 30 min in RIPA lysis buffer (Bocai, Shanghai, China) supplemented with protease inhibitors. For western blot analysis, 20 μg of the sample was resolved on a 10 % SDS-PAGE gel and electro-transferred onto nitrocellulose membranes (Whatman, Piscataway, NJ). The following primary antibodies were used at a dilution of 1:500:anti-Cbfα1 (Abcam, Cambridge, MA, USA). For the normalization of protein loading, a GAPDH (Cell Signaling Technology Inc., Danvers, MA) antibody was used at a 1:2000 dilution. HRP-conjugated secondary antibodies were used at a 1:5000 dilution. The antigen–antibody complexes were visualized using the Enhanced Chemiluminescence Detection System (Milipore, Billerica, MA), according to the manufacturer’s protocols. Protein band intensities on the scanned films were compared to their respective controls using Alpha Image software.
Statistical analysis
All experiments were performed at a minimum of three times. All measurements are expressed as mean ± SD. Significant differences between non-load and stretch groups were determined using ANOVA (p < 0.05 denotes statistical significance).
Results
Proliferation of BMSCs (OVX)
When BMSCs were subjected to mechanical stretch of 5 and 10 % elongation, proliferation in the OVX and Sham groups was inhibited significantly at day 3. Then, cell proliferation plateaued in both the loaded and non-loaded groups (Fig. 1a, b). Compared with the Sham group, a significant decrease in the proliferation of BMSCs (OVX) was induced by both 5 and 10 % elongation at days 3 and 5. Finally, both cells showed slight increase and maintained at the same level (Fig. 1c, d).
Effects of mechanical stretch on cell proliferation in BMSCs. a Proliferation in BMSCs (OVX) at different elongations. b Proliferation in BMSCs (Sham) at different elongations. c Comparison of proliferation in BMSCs (OVX) and BMSCs (Sham) with 0 % elongation. d Comparison of proliferation in BMSCs (OVX) and BMSCs (Sham) with 5 % elongation. e Comparison of proliferation in BMSCs (OVX) and BMSCs (Sham) with 10 % elongation
Orientation of BMSCs
After BMSCs were subjected to mechanical stretch of 10 % elongation for 3 days, an obvious re-orientation of the cytoskeleton in loaded BMSCs (OVX) was observed (Fig. 2a, b); the cells were aligned nearly perpendicularly to the stress direction, in contrast to non-loaded (control) cells which showed no particular orientation. There was also a clear re-orientation of the cytoskeleton in loaded BMSCs (Sham), compared with non-loaded cells (Fig. 2c, d).
Effects of mechanical stretch on orientation of the cytoskeleton. BMSCs (OVX) and BMSCs (Sham) were plated at 1 × 105 cells/mL and precultured for 1 day until 95 % confluent. Then, cells were subjected to 10 % intermittent elongation for 3 days. a Non-loaded BMSCs (OVX) oriented randomly. b BMSCs (OVX) that were subjected to stress (10 % elongation) oriented perpendicularly to the stretch axis. c Non-loaded BMSCs (OVX) oriented randomly. d BMSCs (OVX) that were subjected to stretch (10 % elongation) oriented perpendicularly to the stretch axis. Arrows show the direction of stretch field
Alkaline phosphatase activity
When BMSCs (OVX) and BMSCs (Sham) were intermittently subjected to mechanical stretch for 7 days, ALP activity was detected. Compared with the non-loaded group (0 % group), ALP activities of BMSCs in the loaded groups (5 and 10 % elongation groups) showed a significant increase at day 7 (Fig. 3). The ALP activity was larger in the 10 % elongation group than that in the 5 % elongation group. However, ALP activity in BMSCs (OVX) was relatively lower than that in BMSCs (Sham) at both 5 and 10 % elongation.
Effects of mechanical stretch on ALP activity in BMSCs (OVX) and BMSCs (Sham). ALP activity of BMSCs stretched with 5 and 10 % elongation increased significantly at day 7, and the larger the elongation, the more ALP activity. However, ALP activity in BMSCs (OVX) was less than in BMSCs (Sham) with 10 % elongation. No significant difference in ALP activity was observed with 5 % elongation at day 7. Scale bar 100 μm
Magnitude-related osteoblastic differentiation of BMSCs (OVX) and BMSCs (Sham)
To examine the initiation of osteogenesis as a result of mechanical stretching, we assessed the induction of the osteoblastic differentiation markers ALP, collagen I (Col I), and OCN at the mRNA level. Levels of mRNA were assessed by real-time PCR using a Light-Cycler. Both BMSCs (OVX) and BMSCs (Sham) were subjected to intermittent mechanical stretch.
Compared with the non-loaded group, both 5 and 10 % elongation stress promoted mRNA expression of ALP, Col I, and OCN in both BMSCs (OVX) and BMSCs (Sham) (Fig. 4a–f). The mRNA expression of ALP, Col I, and OCN in the 5 % elongation group was up-regulated at day 3 in both BMSCs (OVX) and BMSCs (Sham) (Fig. 4). The expression of these osteoblastic genes in the 10 % elongation group was also promoted at days 3 and 7. Moreover, expression of all these genes increased significantly at day 7 compared to that at day 3 (Fig. 4a–c).
Osteogenic expression in BMSCs (OVX) and BMSCs (Sham) was promoted by mechanical stretch. a ALP mRNA, b Collagen I mRNA, c OCN mRNA, d ALP mRNA, e Collagen I mRNA, f OCN mRNA, g 5 % elongation, and h 10 % elongation. Values are expressed relative to the control groups, normalized as 1.0. Results are presented as mean ± SD (* p < 0.05 and ** p < 0.01, unpaired Student’s t test; n = 3)
Comparison of osteoblastic differentiation between BMSCs (OVX) and BMSCs (Sham)
There was a significant difference in the expression of collagen I mRNA at 5 % elongation between BMSCs (OVX) and BMSCs (Sham) at day 3, while no significant difference was observed in the other osteoblastic genes at days 3 and 7 (p < 0.01, Fig. 4g). However, the osteoblastic gene expression in BMSCs (OVX) at 10 % elongation was lower than that in BMSCs (Sham) at both days 3 and 7 (p < 0.05, Fig. 4h). Moreover, the difference in osteoblastic gene expression between BMSCs (OVX) and BMSCs (Sham) was larger at day 7 than that at day 3.
Runx2 expression
Expression levels of Runx2 mRNA and protein were estimated by real-time PCR and Western blotting, respectively. The Runx2 mRNA level increased in both cells in the 5 and 10 % elongation groups. This increasing trend was more significant in the 10 % elongation group at day 7 (p < 0.05, Fig. 5a). Runx2 mRNA expression at 5 % elongation was up-regulated significantly at day 3 (p < 0.05), but fell back at day 7, though still higher than the unstressed group (p < 0.05) in both BMSCs (OVX) and BMSCs (Sham). Compared with BMSCs (Sham), lower Runx2 mRNA expression in BMSCs (OVX) was observed in the stress group, and the difference was more significant in the 10 % elongation group (p < 0.05, Fig. 5b). Runx2 protein expression was promoted by mechanical stretch in a similar way to the mRNA expression (Fig. 6).
Runx2 protein expression was promoted by mechanical stretch in both BMSCs (OVX) and BMSCs (Sham). a Runx2 protein expression was illustrated by Western blotting. Whole cell extracts (20 μg per lane for Runx2) were prepared at the indicated time points. b The densitometry of Runx2 protein expression was analyzed. c Comparison of Runx2 protein expression at 3 and 7 days in BMSCs (OVX) and BMSCs (Sham) with 5 % elongation and 10 % elongation, respectively. The values are mean ± SD. The differences were significant, * p < 0.05 and ** p < 0.01
Discussion
It is known that mechanical stress is an important regulatory factor in bone formation and remodeling [21]. Many researchers have demonstrated the osteogenic effects of mechanical stress both in vivo and in vitro studies. Treadmill exercise running was shown to preserve bone strength and to induce bone turnover changes in favor of bone formation [22]. Vibration at low magnitude and high frequency can stimulate new trabecular bone formation in sheep [23], expression of osteoblastic genes associated with bone formation in cultured osteoblasts [24], remodeling in mice [25], and new cortical bone formation in the mouse ulna [26]. A vibratory stimulus can also prevent bone loss and reductions in bone strength in ovariectomized rats [27, 28]. However, there are few reports on cultured cells in osteoporosis subjected to mechanical stimuli in vitro. In this study, we sought to explore the response of osteoporosis BMSCs to mechanical stimuli in vitro.
BMSCs, as stem cells, are capable of differentiating along multiple mesenchymal lineages, such as bone, cartilage, muscle, adipose tissue, and ligament. They can readily be expanded in vitro using routine cell culture techniques [14, 15, 29, 30]. As a result, BMSCs have been identified as an appropriate cell source for a wide variety of tissue engineering strategies.
Regarding the pathogenesis of osteoporosis, it is believed that in the disease, adipose accumulation and bone loss are due to a differentiation change in BMSCs—the progenitor of osteoblasts and adipocytes. We have demonstrated previously that the proliferation of BMSCs (OVX) was slower than that of BMSCs (Sham), and the osteoblastic differentiation ability of BMSCs (OVX) is weaker than that of BMSCs (Sham) (data not shown). In this study, we found that osteoblastic differentiation of BMSCs (OVX) was promoted significantly in response to stretch stimulation; however, proliferation was inhibited.
The effect of mechanical stretch on the proliferation of osteoblastic cells is still controversial. In some studies, mechanical stretch decreased the proliferation of osteoblastic cells [31, 32], whereas in others, it increased [33, 34]. In our study, mechanical stretching of 5 and 10 % elongation inhibited the proliferation of both BMSCs (OVX) and BMSCs (Sham), and this inhibition was even more significant at 10 % elongation (p < 0.05; Fig. 1). Moreover, the proliferation of BMSCs (OVX) was slower than that of BMSCs (Sham) at the same elongation. Application of mechanical stretch to BMSCs induced significant changes in their orientation. Compared with the non-loaded cells, both loaded BMSCs (OVX) and BMSCs (Sham) oriented perpendicular to the stress axis (Fig. 2). The orientation change was also reported by Buckley et al. [35]. Regarding the mechanism of stretch-dependent orientation, it has been suggested that cells opt for a nearly perpendicular orientation to minimize the force acting on them due to the external strain, and organize their focal adhesions and stress fibers accordingly so that the local matrix stress reaches an optimal value [36].
ALP activity and expression of OCN are used as indicators of osteoblastic activity. Extracellular matrix molecules, such as type I collagen, are considered to play important roles in osteoblast proliferation and differentiation. In our study, ALP activity was enhanced significantly at 10 % elongation in BMSCs (OVX) and BMSCs (Sham); however, it was lower in BMSCs (OVX) than in BMSCs (Sham). The mRNA expression of ALP, Col I, and OCN was up-regulated at 5 and 10 % elongation in both BMSCs (p < 0.05; Fig. 3, 4). Osteoblastic differentiation induced by 5 % elongation was observed primarily at day 3, whereas stress of larger magnitude (10 % elongation) induced higher osteoblastic gene expression at day 7. This is consistent with an early study in vivo, which showed that mechanical stimulus with a 7-day rest is more effective in improving biomechanical properties and micromorphology in ovariectomized rats compared with daily loading [37]. However, Mariko et al. [34] reported that mechanical strain stimulated osteoblastic differentiation of stromal cells at low magnitudes. Human osteoblastic cells under mechanical stretch (1 % elongation, 30 min/day, for 2 days) were shown to increase proliferation and type I collagen propeptide release, but showed reduced ALP activity and OCN release [38]. These in vitro results differ from ours obtained at low magnitudes of strain (Fig. 4). In general, the response of the cells to mechanical stretch may depend on the type and magnitude of the stress applied to them. Moreover, the differences in response to the mechanical stretch may be attributable to the differentiation stage and cell culture condition used in the experiment [39, 40]. Furthermore, our results indicate that the mRNA expression of osteoblast-related genes in BMSCs (OVX) under mechanical stress was not increased as much as that in BMSCs (Sham).
Runx2, the runt-related transcription factor 2 responsible for regulating the expression of several bone- and cartilage-related genes [41], is required for bone formation in vivo [42, 43]. It has been reported that the mRNA level of Runx2 can be up-regulated with shorter stretching times, such as 6 h, and down-regulated at higher levels of strain with longer elongation times, of 24 and 48 h [44, 45]. Our results demonstrated that expression of Runx2 in both BMSCs (OVX) and BMSCs (Sham) could increase continuously in response to stress stimulation of 10 % elongation. However, at a lower magnitude of stress (5 %) the Runx2 expression dropped at day 7. Similar to the osteoblastic marker genes, the expression of Runx2 in BMSCs (OVX) under mechanical stress was increased less than that of BMSCs (Sham).
In conclusion, mechanical stress applied to both BMSCs (OVX) and BMSCs (Sham) inhibited proliferation, but resulted in osteoblastic differentiation. A higher magnitude of stress induced osteoblastic differentiation in both BMSCs (OVX) and BMSCs (Sham) continuously; however, lower magnitude stress induced the osteoblastic differentiation significantly at the early phase. On the whole, the osteoblastic differentiation ability of BMSCs (OVX) was weaker than that of BMSCs (Sham). These results indicate that to reach the same bone mass, mechanical stress should be applied longer to BMSCs (OVX) than to BMSCs (Sham); moreover, low-magnitude stress should be applied earlier, and a higher magnitude of stress should be applied later for more osteogenesis.
References
Riggs BL, Khosla S, Melton LJ (2002) Sex steroids and the construction and conservation of the adult skeleton. Endocr Rev 23(3):279–302
Pacifici R (1998) Editorial: cytokines, estrogen, and postmenopausal osteoporosis-the second decade. Endocrinology 139(6):2659–2661
Weitzmann MN, Pacifici R (2006) Estrogen deficiency and bone loss: an inflammatory tale. J Clin Invest 116(5):1186–1194
Rodan GA, Martin TJ (2000) Therapeutic approaches to bone diseases. Science 289(5484):1508–1514
Reid IR (2002) Pharmacotherapy of osteoporosis in postmenopausal women: focus on sagety. Expert Opin Drug Saf 1(1):93–107
Yeh IT (2007) Postmenopausal hormone replacement therapy: endometrial and breast effects. Adv Anat Pathol 14(1):17–24
Suzanne LF, Roger T, Titi T et al (2011) Mitigation of bone loss with ultrasound induced dynamic mechanical signals in an OVX induced rat model of osteopenia. Bone 48(5):1095–1102
Ehrlich PJ, Lanyon LE (2002) Mechanical strain and bone cell function: a review. Osteoporos Int 13:688
Duncan RL, Turner CH (1995) Mechanotransduction and the functional response of bone to mechanical strain. Calcif Tissue Int 57:344
Howe TE, Shea B, Dawson LJ et al (2011) Exercise for preventing and treating osteoporosis in postmenopausal women. Cochrane Database Syst Rev 3:CD000333. doi:10.1002/14651858
Kreke MR, Huckle WR, Goldstein AS (2005) Fluid flow stimulates expression of osteopontin and bone sialoprotein by bone marrow stromal cells in a temporally dependent manner. Bone 36:1047–1055
Wu Y, Zhang X, Zhang P et al (2012) Intermittent traction stretch promotes the osteoblastic differentiation of bone mesenchymal stem cells by the ERK1/2-activated Cbfa1 pathway. Connect Tissue Res 53(6):451–459
Zhang P, Wu Y, Jiang Z et al (2012) Osteogenic response of mesenchymal stem cells to continuous mechanical strain is dependent on ERK1/2-Runx2 signaling. Int J Mol Med 29(6):1083–1089. doi:10.3892/ijmm.2012.934
Prockop DJ (1997) Marrow stromal cells as stem cells for nonhematopoietic tissues. Science 276:71–74
Pittenger MF, Mackay AM, Beck SC et al (1999) Multilineage potential of adult human mesenchymal stem cells. Science 284:143–147
Kalu DN (1991) The ovariectomized rat model of postmenopausal bone loss. Bone Miner 15:175–192
Zhu L, Zhao XY, Lu XG (2009) Ovariectomy-associated changes in bone mineral density and bone marrow haematopoiesis in rats. Int J Exp Pathol 90(5):512–519
Kim TH, Jung JW, Ha BG et al (2011) The effects of luteolin on osteoclast differentiation, function in vitro and ovariectomy-induced bone loss. J Nutr Biochem 22:8–15
Park SB, Lee YJ, Chung CK (2010) Bone mineral density changes after ovariectomy in rats as an osteopenic model: stepwise description of double dorso-lateral approach. J Korean Neurosurg Soc 48:309–312
Thommasini DD, Simmons HA, Pirie CM et al (1995) FDA guidelines and animal models for osteoporosis. Bone 17:125S–133S
Turner CH (2006) Bone strength: current concepts. Ann N Y Acad Sci 1068:429–446
Lespessailles E, Jaffré C, Beaupied H et al (2009) Does exercise modify the effects of zoledronic acid on bone mass, microarchitecture, biomechanics, and turnover in ovariectomized rats. Calcif Tissue Int 85(2):146–157
Rubin C, Turner AS, Mallinckrodt C et al (2002) Mechanical strain, induced noninvasively in the high frequency domain, is anabolic to cancellous bone, but not cortical bone. Bone 30:445–452
Tanaka SM, Li J, Duncan RL et al (2003) Effects of broad frequency vibration on cultured osteoblasts. J Biomech 36:73–80
Judex S, Zhong N, Squire ME et al (2005) Mechanical modulation of molecular signals which regulate anabolic and catabolic activity in bone tissue. J Cell Biochem 94:982–994
Tanaka SM, Alam I, Turner CH (2003) Stochastic resonance in osteogenic response to mechanical loading. FASEB J 17:313–314
Oxlund BS, Ortoft G, Andreassen TT et al (2003) Low intensity, high-frequency vibration appears to prevent the decrease in strength of the femur and tibia associated with ovariectomy of adult rats. Bone 32:69–77
Flieger J, Karachalios TH, Khaldi L et al (1998) Mechanical stimulation in the form of vibration prevents postmenopausal bone loss in ovariectomized rats. Calcif Tissue Int 63:510–514
Haynesworth SE, Goshima J, Goldberg VM et al (1992) Characterization of cells with osteogenic potential from human marrow. Bone 13:81–88
Bianco P, Gehron RP (2000) Marrow stromal stem cells. J Clin Invest 105:1663–1668
Cheng G, Tse J, Jain RK et al (2009) Micro-environmental mechanical stress controls tumor spheroid size and morphology by suppressing proliferation and inducing apotosis in cancer cells. PLoS ONE 4(2):e4632
Beltramo E, Berrone E, Giunti S et al (2006) Effects of mechanical stress and high glucose on pericyte proliferation, apoptosis and contractile phenotype. Exp Eye Res 83(4):989–994
Li B, Li F, Puskar KM et al (2009) Spatial patterning of cell proliferation and differentiation depends on mechanical stress magnitude. J Biomech 42(11):1622–1627
Mariko K, Hitoyata S, Zuisei K et al (2005) Effects of mechanical strain on proliferation and differentiation of bone marrow stromal cell line ST2. J Bone Miner Metab 23:219–225
Buckley MJ, Banes AJ, Levin LG et al (1988) Osteoblasts increase their rate of division and align in response to cyclic, mechanical tension in vitro. Bone Miner 4:225–236
De R, Zemel A, Safran S (2007) Dynamics of cell orientation. Nat Phys 3(9):655–659
Ma R, Zhu D, Gong H et al (2012) High-frequency and low-magnitude whole body vibration with rest days is more effective in improving skeletal micro-morphology and biomechanical properties in ovariectomised rodents. Hip Int 22(2):218–226
Kaspar D, Seidl W, Neidlinger-Wilke C et al (2000) Dynamic cell stretching increases human osteoblast proliferation and CICP synthesis but decreases osteocalcin synthesis and alkaline phosphatase activity. J Biomech 33:45–51
Weyts FA, Bosmans B, Niesing R et al (2003) Mechanical control of human osteoblast apoptosis and proliferation in relation to differentiation. Calcif Tissue Int 72:505–512
Yokota MT, Suzuki Y, Kawase T et al (1996) Distinct responses of different populations of bone cells to mechanical stress. Endocrinology 137:2028–2035
Qi MC, Zou SJ, Han LC et al (2009) Expression of bone-related genes in bone marrow MSCs after cyclic mechanical strain: implications for distraction osteogenesis. Int J Oral Sci 1:143–150
Ducy P, Zhang R, Geoffroy V et al (1997) Osf2/Cbfa1: a transcriptional activator of osteoblast differentiation. Cell 89:747–754
Otto F, Thornell AP, Crompton T et al (1997) Cbfa1, a candidate gene for cleidocranial dysplasia syndrome, is essential for osteoblast differentiation and bone development. Cell 89:765–771
Burger EH, Klein-Nulend J (1999) Mechanotransduction in bone–role of the lacuno-canalicular network. FASEB J 13(Suppl):S101–S112
Pratap J, Galindo M, Zaidi SK et al (2003) Cell growth regulatory role of Runx2 during proliferative expansion of preosteoblasts. Cancer Res 63:5357–5362
Acknowledgments
This work was supported by grants from the National Nature Science Foundation of China (Nos.30901698, 10972142), the Collaborative Foundation of Medical and Engineering Science of Shanghai JiaoTong University (No. YG2012MS40), the Key Basic Research Foundation of the Shanghai Committee of Science and Technology (No. China 12JC1405700), and the “Chen Xing” project from Shanghai Jiaotong University, and also supported by the Innovative Research Team of Shanghai Municipal Education Commission. The authors would like to thank Professor Kerong Dai, Xiaoling Zhang, and Lab of Orthopaedics Cellular and Molecular Biology for generously providing the experimental situation. They also greatly thank Professor Zonglai Jiang and Institute of Mechanobiology and Medical Engineering, Shanghai Jiao Tong University.
Author information
Authors and Affiliations
Corresponding authors
Additional information
Yuqiong Wu and Peng Zhang have contributed equally to this work.
Rights and permissions
About this article
Cite this article
Wu, Y., Zhang, P., Dai, Q. et al. Effect of mechanical stretch on the proliferation and differentiation of BMSCs from ovariectomized rats. Mol Cell Biochem 382, 273–282 (2013). https://doi.org/10.1007/s11010-013-1744-1
Received:
Accepted:
Published:
Issue Date:
DOI: https://doi.org/10.1007/s11010-013-1744-1