Abstract
MoSe2 nanosheets spheres (MoSe2·NSs) were synthesized directly on the surface of reduced graphene oxide (rGO) nanosheets (MoSe2–rGO) using a simple one-pot hydrothermal approach, which was used for supercapacitor. The synergistic effect of the MoSe2·NSs and the highly conductive rGO network endows the MoSe2–rGO composite excellent electrochemical performance. The effect of the content of graphene in the composite were investigated in details. The optimal electrode exhibits a high specific capacitance of 814.4 F g−1 at 1 A g−1 in 2 M KOH. Moreover, the assembled supercapacitor delivers a high specific capacitance of 215.7 F g−1 at 1 A g−1 and retains 81.7% of the initial capacitance at 10 A g−1 after 5000 cycles. It suggests that it has potential as an electrode material for high-performance electrochemical supercapacitors.
Similar content being viewed by others
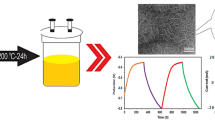
Avoid common mistakes on your manuscript.
1 Introduction
Supercapacitor, also called electrochemical supercapacitor, is a promising energy storage equipment between conventional capacitors and batteries, which exhibits high power density, good cyclic stability, and excellent reversibility [1, 2]. Supercapacitor can be divided into two basic types due to the different charge storage mechanisms: electrical double-layer capacitors (EDLCs) and pseudocapacitors. The former stores charge by charge separation at the electrode–electrolyte interface and the latter is mainly attributed to a fast Faraday reaction [3,4,5]. Pseudocapacitors have a higher specific capacitance than EDLC, but its specific capacitance tends to gradually decrease due to poor electrical conductivity. It has become a major research subject to synthesize materials which can simultaneously apply these two mechanisms [6,7,8].
Transition metal dichalcogenides (TMDC) become an ideal choice for advanced electrode materials of supercapacitor owing to their 2D sheet-like morphology, large surface area and diverse material property [9,10,11,12]. TMDC compounds with a general formula MX2 (M: Mo or W, X: S or Se), are subjected to strong covalent bonds (X–M–X), whereas the individual layers are stacked together by weak van der Waals interactions [13,14,15]. Among them, MoSe2, an semiconductor with a band gap of ~ 1.5 eV, has high theoretical capacity and low cost, which has been investigated and is considered as one of the attractive materials for supercapacitor electrode materials [16,17,18]. Similar to that of other metal oxides, its specific capacitance decline significantly with the increase of scanning rate as a result of the poor conductivity [19].
Graphene, an atomic sheet of sp2-hybrided carbon atoms, has a large specific surface area, high conductivity and ultrahigh chemical stability [20,21,22,23]. Currently, the preparation method of graphene mainly includes reduction of graphene oxide (GO) and chemical vapor deposition. Chemical reduction of GO to reduced GO (rGO) has the characteristics of large-scale preparation and low cost, which has attracted people’s attention [24]. In addition, the surface of RGO has oxygen-containing functional groups, resulting in its good hydrophilicity and facile to be modified to produce graphene matrix composites [25]. Graphene exhibits a superior stability, but the capacitance is relatively low due to the mechanism of EDLCs [26, 27]. The combination of graphene and MoSe2 can increase not only the electrical conductivy but also give full play to pseudocapacitance of the MoSe2 and double-layer capacitance characteristics of the graphene.
Some researches based on MoSe2 material for supercapacitor have been reported in recent years. Juns et al. synthesized MoSe2 nanosheets and measured it in the 1 M H2SO4, which showed a specific capacity of 30 F g−1 at 1 A g−1 [16]. Gao et al. reported the MoSe2 spheres prepared by hydrothermal sythesis, which demonstrated a specific capacitance of 243 F g−1 at 0.5 A g−1 in the 2 M KOH [17]. Karade and Sankapal reported the MoSe2/MWCNTs hybrid for supercapacitor electrode, which showed a specific capacitance of 232 F g−1 at 1.4 A g−1 [18]. Huang et al. synthesized layered MoSe2 nanosheets on Ni-foam via a hydrothermal method and it exhibited a specific capacitance of 1114.3 F g−1 at 1 A g−1 [28]. Balasingam et al. prepared MoSe2/rGO nanosheet for supercapacitor electrode and showed a specific capacitance of 211 F g−1 at a scan rate of 5 mV s−1. However, when the scan rate of 125 mV s−1, its specific capacitance drops rapidly to 100 F g−1 [29]. This paper only compared the electrochemical properties of MoSe2/rGO and pure MoSe2. The influence of graphene content on the performance of supercapacitors was not investigated in details. However, it is necessary to explore the influence of graphene content on the electrochemical performance of composites for supercapacitors.
Herein, the MoSe2 nanosheet spheres (MoSe2·NSs)–rGO composite were synthesized by a facile one-pot hydrothermal approach. The MoSe2–rGO composite reveals a synergistic effect, which results in the enhanced performance. The optimal electrochemical performance (814.4 F g−1 at 1 A g−1) is achieved in the MoSe2–rGO-25 composite. Moreover, the assembled supercapacitor based on MoSe2–rGO-25 composite also possesses a high specific capacitance of 215.7 F g−1 at 1 A g−1 and long cycle life with 81.7% capacitance retention after 5000 cycles at 10 A g−1. It can be applied as a promising supercapacitor electrode.
2 Experimental
2.1 Chemical and reagents
Graphite powders were supplied by Sigma Aldrich. Poly(tetrafluoroethylene) binder (PTFE), Nickel foam and carbon black was purchased from Macklin reagent. Se powders, Na2MoO4 and N2H4·H2O were brought from Tianjin Guangfu Fine Chemical Research Institute (China). KMnO4, KOH, H2O2 (30 wt%), HCl (36 wt%), H2SO4 (98 wt%) and ethanol were brought from Tianli Chemical Reagent Co., Ltd (China). Spring gasket, stainless steel gasket, separator and LIR 2016 coin cell case were purchased from Shenzhen Tiancheng Technology Co., Ltd (China).
2.2 Preparation of MoSe2–rGO composites
Figure 1 shows the schematic of the synthesis of MoSe2–rGO nanocomposite for supercapacitor. GO was synthesized by a modified Hummers’ method using natural graphite powders as the raw material, which is similar to the previous report [30]. Firstly, 25 mg GO was first ultrasonically dispersed in 65 mL distilled water to form a suspension. 2 mmol Na2MoO4 was added into the GO suspension. At the same time, 4 mmol Se powders were dissolved in 10 mL N2H4·H2O under the constant magnetic stirring in a separate beaker. The hydrate–Se solution was also slowly poured into the above GO suspension (Fig. 1a). Then, the mixture was transferred into a 100 mL Teflon-lined stainless steel autoclave and maintained at 200 °C for 12 h (Fig. 1b). The black precipitates were collected and washed by DI water and ethanol for three times. The final product (MoSe2–rGO-25) was obtained after freeze-drying (Fig. 1c). The GO was reduced into rGO in this process and MoSe2 NSs are grown on the surface of the rGO via the in-situ reduction of the MoO42− and Se powders by N2H4·H2O. In order to investigate the effect of graphene content on the electrochemical properties, GO with 15, 35, 50, 100 mg was also selected to combine with 2 mmol Na2MoO4 and 4 mmol Se powders, and the as-prepared sample was marked as MoSe2–rGO-15, MoSe2–rGO-35, MoSe2–rGO-50, MoSe2–rGO-100, respectively. Meanwhile, the pure MoSe2 and rGO were also prepared under the same condition.
2.3 Characterizations
The morphologies of the MoSe2, rGO and MoSe2–rGO were acquired by a scanning electron microscope (SEM) (JSM7000F, JEOL). The X-ray diffraction (XRD) was conducted on a Rigaku Rotaflex D/MAX diffractometer using the Cu Kα radiation.
2.4 Eletrochemical measurements
All electrochemical measurements were achieved in a standard three-electrode system on a VMP3 electrochemical analyzer (France) at room temperature. As-prepared active materials, carbon black and PTFE were mixed in a mass ratio of 80:10:10 to obtain a viscous slurry and coated onto the nickle foam current collector (1 × 1 cm). The prepared active material loaded on the electrode was around 5 mg per electrode. The nickel foam wrapped in slurry was dried at 80 °C. Subsequently, the dried nickel foams were pressed into a thin foil and used as the working electrode. The reference and counter electrode were composed by an Ag/AgCl (3.0 M KCl) and a platinum foil electrode, respectively. The electrolyte used was 2 M KOH. In addition, the symetric supercapacitor was prepared with two electrodes, electrolyte, spring gasket, stainless steel gasket and separator in the LIR2016 coin cell. The coin cell was crimped using hydraulic cell crimping machine (MSK-110, China). Photograph of the electrochemical tests and the assembled supercapacitor are shown in Fig. 1d, e. The internal construction schematic of the assembled supercapacitor is shown in Fig. 1f. Electrochemical impedance spectroscopy (EIS) was obtained in the frequency range from 10 mHz to 100 kHz at open circuit potential with an ac perturbation of 5 mV. Cyclic voltametry (CV) responses were recorded at various scan rates of 10 to 100 mV s−1 from − 0.2 to 0.8 V. Galvanostatic charge discharge (GCD) curves were measured from 0 to 0.5 V at current densities of 1, 2, 4, 6, 8, 10 A g−1. The discharge capacitance (C) of the asprepared electrode was calculated based on the following formula [31]:
where, \(I\) is the current (A), \(\Delta t\) denotes the discharging time (s), m is the weight of active materials (g), \(\Delta V\) is the potential change in the discharge process.
The energy density (E) and power density (P) of the assembled supercapacitor according to the following equations [32]:
where, C is the capacitance of the assembled supercapacitor, \(\Delta V\) is the potential range and \(\Delta t\) is the discharge time.
3 Results and discussion
3.1 Characterizations of the MoSe2–rGO nanocomposite
The SEM images and structures of the MoSe2, rGO and MoSe2–rGO nanocomposite are displayed in Fig. 2. It can be clearly observed that the pure MoSe2 is composed of interlaced nanosheet spheres and they seriously agglomerate together (Fig. 2a). MeSe2·NSs are densely dispersed on the surface of rGO network when the content of rGO nanosheets is smaller for MoSe2–rGO-15 (Fig. 2b). With the increase of rGO content, MeSe2·NSs are almost uniformly dispersed on the surface of rGO nanosheets, as shown in MoSe2–rGO-25 (Fig. 2c). The magnified image of MoSe2–rGO-25 reveales that MoSe2 and rGO are tightly integrated. When the rGO content continues to increase, MoSe2 NSs are sparsely interspersed on the surface of rGO nanosheets, as shown in MoSe2–rGO-100 (Fig. 2d). The rGO nanosheets exist in wrinkle shape, as shown in Fig. 2e. Figure 2f shows the XRD patterns of rGO, MoSe2 and MoSe2–rGO. The diffraction peaks of rGO at 26.3° and 41.5° represent the (002) and (001) reflections of graphene (JCPDS 75-1621), respectively. The diffraction peak of MoSe2 at 13.2°, 31.8°, 37.9°, 44.8°, 55.6° and 69.2° corresponds to the (002), (100), (103), (105), (110) and (200) plane of MoSe2, respectively (JCPDS: 77-1715) [33]. The intensity of the (002) plane peak of MoSe2 is weaker than that of the MoSe2–rGO, indicating that MoSe2·NSs are full of rGO network.
SEM images and structures of the MoSe2, rGO and MoSe2–rGO nanocomposite. a SEM image of the MoSe2. Inset: magnified image of MoSe2. b SEM image of the MoSe2–rGO-15. c SEM image of MoSe2–rGO-25. Inset: magnified image of MoSe2–rGO-25. d SEM image of the MoSe2–rGO-100. e SEM image of the rGO. f XRD patterns of the MoSe2, rGO and MoSe2–rGO nanocomposite
The electrochemical behavior of the MoSe2–rGO electrode was characterized in a typical three-electrode system. Figure 3a shows the CV curves of the electrodes with different rGO contents at a scan rate of 20 mV s−1 in 2 M KOH. The CV curve shows the presence of redox peaks, which might be occurred due to the electrochemical insertion/extraction of K+ ions in the interlayer of layered MoSe2·NSs. According to previous reports, the reaction may be accompanied by the faradaic and non-faradaic processes of the MoSe2 [18].
Electrochemical performance of the MoSe2–rGO nanocomposite. a CV curves of the different electrodes at a scan rate of 20 mV s−1. b GCD curves of the different electrodes at 1 A g−1. c Specific capacitances of the MoSe2–rGO-25 electrode at 1 A g−1. d CV curves of the MoSe2–rGO-25 at different scan rates.e GCD curves of the MoSe2–rGO-25 electrode at various current densities. f Specific capacitances of the MoSe2–rGO-25 electrode at various current densities
The area under the CV curve of MoSe2–rGO-25 is obviously larger than that of other materials. This demonstrates that the MoSe2-rGO-25 electrode has higher capacitance than that of the other electrode. Figure 3b shows the GCD curves of various electrode materials at a current density of 1 A g−1. The MoSe2–rGO-25 electrode shows the largest discharging time. Based on the GCD curves, the specific capacitance is calculated using Eq. (1). The calculated specific capacitance of various electrodes at 1 A g−1 increases intially and later decreases with increasing the initial GO content (Fig. 3c). In the MoSe2–rGO-25, it is the highest, which is consistent with the CV result. This can be attributed to the good combination of MoSe2 and rGO, which promotes the diffusion of ions and electrons in the electrolyte solution. This indicates that the graphene content has a great effect on the specific capacitance of the nanocomposite. Moreover, the peak current increases with the increase of scan rate and the CV curves almost keep the same profiles, which suggests this material has an excellent reversibility during the redox reactions at electrode/electrolyte interfaces (Fig. 3d).
Figure 3e shows GCD curves of the MoSe2–rGO-25 at various current densities. The MoSe2–rGO-25 electrode shows the largest discharging time at 1 A g−1. The discharge specific capacitance values are calculated to be 814.4, 635.8, 491.7, 451.3, 419.7 and 403.9 F g−1 at 1, 2, 4, 6, 8 and 10 A g−1, respectively (Fig. 3f). It can be seen that with increasing the curent density, the discharge specific capacitance of the MoSe2–rGO-25 electrode decreases. This may be the insufficient Faradaic redox reactions of the active material at higher discharge current densities. Moreover, the CV and GCD curves of other as-prepared electrodes are shown in Fig. S1–S6, respectively. The shape of CV and GCD curves of other MoSe2–rGO electrodes is consistent with the MoSe2–rGO-25, but the specific capacity is lower than it (Fig. S1–S5). The CV curves of rGO electrode take roughly rectangular shapes and the GCD curves of rGO electrode present a typical triangular shapes, which indicates that the rGO electrode exhibits only double-layer capacitance characteristics (Fig. S6).
EIS analysis was used to study the resistive and capacitive behaviors of the electrode materials for supercapacitor. Figure 4 shows the Nyquist plots of rGO, MoSe2 and various MoSe2–rGO electrode. The slope of the curve of MoSe2–rGO-25 in the low frequency region is between rGO and MoSe2, which is slightly lower than that of pure rGO and higher than that of other MoSe2–rGO electrodes, indicating that it has a good equivalent series resistance. The Nyquist plot is explained by the corresponding fitting equivalent circuit (inset of Fig. 4a), where Rs stands for the intrinsic ohmic resistance (comes from the intrinsic resistances of electrode material, electrolyte, current collector, leads and separator, as well as the contact resistances between them), which can be suggested by the first intercept along the real axis [34]. Rct is the interfacial charge transfer resistance (comes from the electronic and ionic resistances at the interface between the electrode and the electrolyte), which can be suggested by the diameter of the semicircle. This resistance mainly depends on the wettability between the electrolyte and electrode, the morphology and conductivity of electrode [35]. The Warburg diffusion resistance (Zw) comes from the resistance for ion diffusion from electrolyte into the electrode, corresponding to the projected length of Warburg region on the real axis [36]. Cdl is the double layer capacitance and CL is the Faradic capacitance [37]. The calculated Rct values for the rGO, MoSe2, MoSe2–rGO-15, MoSe2–rGO-25, MoSe2–rGO-35, MoSe2–rGO-50 and MoSe2–rGO-100 electrodes are 2.15, 4.06, 3.45, 2.71, 2.88, and 2.64 Ω, respectively, which indicates that the charge transfer performance can be effectively improved by combining MoSe2 and rGO. The Rs values of the rGO, MoSe2, MoSe2–rGO-15, MoSe2–rGO-25, MoSe2–rGO-35, MoSe2–rGO-50 and MoSe2–rGO-100 electrodes are 1.23, 1.31, 1.51, 1.35, 1.24, 1.34 and 1.35 Ω, respectively (Fig. 4b). It is suggested that the active material of the electrode are in good contact with the collector and the electrolyte.
Figure 5 shows the specific discharge capacitances of the different electrodes at various current densities. The specific capacity decreases with increasing the current density and the MoSe2–rGO-25 shows the largest specific capacitance. The improvement of electrochemical properties of the MoSe2–rGO-25 is mainly attributed to the following reasons: (1) MoSe2 NSs anchored on the rGO nanosheets provide more electroactive sites by EDLC as well as pseudocapacitance process; (2) rGO nanosheets effectively improve the electrical conductivity of the composite. (3) The addition of rGO effectively changes the dispersion of MoSe2 NSs in the composite. The excessive content of MoSe2 NSs or rGO hinders the diffusion of ions.
Figure 6a show GCD curves of the assembled supercapacitor based on MoSe2–rGO-25 at various current densities in 2 M KOH. The discharge specific capacitance values are calculated to be 215.7, 186.3, 182.4, 180.9, 173.3, 137.3 F g−1 at 1, 2, 4, 6, 8 and 10 A g−1 (Fig. 6b). A comparison of the specific capacity of the MoSe2–rGO-25 based supercapacitors with previous reports are summarized in Table 1 [38,39,40,41,42,43,44,45]. The cyclic stability of the assembled supercapacitor is evaluated using GCD analysis for 5000 cycles at 10 A g−1 and the specific capacitance of the assembled supercapacitor retains 81.7% (Fig. 6c). This maybe that the close bonding between the rGO and MoSe2 effectively improves the long-term stability. The Ragone plot for the assembled supercapacitor is shown in Fig. 6d. The energy and power densities of the assembled supercapacitor were estimated according to Eqs. (2, 3), respectively. Our supercapacitor device exhibits maximum energy density of 6.9 Wh kg−1 at lower power density of 257.4 W kg−1 at 1 A g−1. The energy density was 1.2 Wh kg−1 with a power density of 1127.6 W kg−1. The superior performance of the supercapacitor shows a great potential in the practical application.
Electrochemical performance of the the assembled supercapacitor. a GCD curves of the assembled supercapacitor at various current densities. b Specific capacitances of the assembled supercapacitor at various current densities. c Cycle test of the assembled supercapacitor with a GCD at a current density of 10 A g−1. d Ragone plots related to energy and power densities of the assembled supercapacitor
4 Conclusions
In summary, The MoSe2–rGO nanocomposite were obtained for supercapacitor. First, GO was prepared by Hummers’ method. Subsequently, GO was added into Na2MoO4 solution and hydrazine hydrate–Se in distilled water for the hydrothermal reaction. The GO was reduced into rGO in this process and MoSe2 NSs are grown on the surface of the rGO. The effect of graphene content on the morphologies and electrochemical properties were investigated in details. The optimal electrode (MoSe2–rGO-25) exhibites a high specific capacitance of 814.4 F g−1 at 1 A g−1. The assembled supercapacitor based on MoSe2–rGO-25 possesses a high specific capacitance of 215.7 at 1A g−1 and excellent cycle life with 81.7% capacitance retention after 5000 cycles at 10 A g−1.
References
J.-H. Choi, C. Lee, S. Cho, G. Moon, B.-S. Kim, H. Chang, H.D. Jang, High capacitance and energy density supercapacitor based on biomass-derived activated carbons with reduced graphene oxide binder. Carbon 132, 16–24 (2018). https://doi.org/10.1016/j.carbon.2018.01.105
M. Liu, Z. Wang, J. Liu, G. Wei, J. Du, Y. Li, C. An, J. Zhang, Synthesis of few-layer 1T′-MoTe2 ultrathin nanosheets for high-performance pseudocapacitors. J. Mater. Chem. A 5, 1035–1042 (2017). https://doi.org/10.1039/c6ta08206h
V.K. Mariappan, K. Krishnamoorthy, P. Pazhamalai, S. Sahoo, S.J. Kim, Electrodeposited molybdenum selenide sheets on nickel foam as a binder-free electrode for supercapacitor application. Electrochim. Acta 265, 514–522 (2018). https://doi.org/10.1016/j.electacta.2018.01.075
W. Yang, L. He, X. Tian, M. Yan, H. Yuan, X. Liao, J. Meng, Z. Hao, L. Mai, Carbon-MEMS-based alternating stacked MoS2@rGO-CNT micro-supercapacitor with high capacitance and energy density. Small (2017). https://doi.org/10.1002/smll.201700639
C. Xiang, M. Li, M. Zhi, A. Manivannan, N. Wu, Reduced graphene oxide/titanium dioxide composites for supercapacitor electrodes: shape and coupling effects. J. Mater.Chem. 22, 19161–19167 (2012). https://doi.org/10.1039/c2jm33177b
B. Zhao, T. Wang, L. Jiang, K. Zhang, M.M.F. Yuen, J.-B. Xu, X.Z. Fu, R. Sun, C.-P. Wong, NiO mesoporous nanowalls grown on RGO coated nickel foam as high performance electrodes for supercapacitors and biosensors. Electrochim. Acta 192, 205–215 (2016). https://doi.org/10.1016/j.electacta.2016.01.211
S. Yang, Y. Liu, Y. Hao, X. Yang, W.A. Goddard, X.L. Zhang, B. Cao, Oxygen-vacancy abundant ultrafine Co3O4/graphene composites for high-rate supercapacitor electrodes. Adv. Sci. 5, 1700659 (2018). https://doi.org/10.1002/advs.201700659
H. Yang, S. Kannappan, A.S. Pandian, J.-H. Jang, Y.S. Lee, W. Lu, Graphene supercapacitor with both high power and energy density. Nanotechnology 28, 445401 (2017). https://doi.org/10.1088/1361-6528/aa8948
S. Mouri, W. Zhang, D. Kozawa, Y. Miyauchi, G. Eda, K. Matsuda, Thermal dissociation of inter-layer excitons in MoS2/MoSe2 hetero-bilayers. Nanoscale 9, 6674–6679 (2017). https://doi.org/10.1039/c7nr01598d
J. Luo, P. Xu, D. Zhang, L. Wei, D. Zhou, W. Xu, J. Li, D. Yuan, Synthesis of 3D-MoO2 microsphere supported MoSe2 as an efficient electrocatalyst for hydrogen evolution reaction. Nanotechnology 28, 465404 (2017). https://doi.org/10.1088/1361-6528/aa8947
B. Zheng, Y. Chen, F. Qi, X. Wang, W. Zhang, Y. Li, X. Li, 3D-hierarchical MoSe2 nanoarchitecture as a highly efficient electrocatalyst for hydrogen evolution. 2D Mater. 4, 025092 (2017). https://doi.org/10.1088/2053-1583/aa6e65
S. Mao, Z. Wen, S. Ci, X. Guo, K.K. Ostrikov, J. Chen, Perpendicularly oriented MoSe2/graphene nanosheets as advanced electrocatalysts for hydrogen evolution. Small 11, 414–419 (2015). https://doi.org/10.1002/smll.201401598
K. Palanisamy, Y. Kim, H. Kim, J.M. Kim, W.S. Yoon, Self-assembled porous MoO2/graphene microspheres towards high performance anodes for lithium ion batteries. J. Power Sour. 275, 351–361 (2015). https://doi.org/10.1016/j.jpowsour.2014.11.001
H. Tang, K. Dou, C.C. Kaun, Q. Kuang, S. Yang, MoSe2 nanosheets and their graphene hybrids: synthesis, characterization and hydrogen evolution reaction studies. J Mater. Chem. A 2, 360–364 (2014). https://doi.org/10.1039/c3ta13584e
L. Ma, L. Xu, X. Zhou, X. Xu, L. Zhang, Synthesis of a hierarchical MoSe2/C hybrid with enhanced electrochemical performance for supercapacitors. RSC Adv. 6, 91621–91628 (2016). https://doi.org/10.1039/c6ra16157j
S.K. Balasingam, J.S. Lee, Y. Jun, Few-layered MoSe2 nanosheets as an advanced electrode material for supercapacitors. Dalton Trans. 44, 15491–15498 (2015). https://doi.org/10.1039/c5dt01985k
Y.P. Gao, K.J. Huang, H.L. Shuai, L. Liu, Synthesis of sphere-feature molybdenum selenide with enhanced electrochemical performance for supercapacitor. Mater. Lett. 209, 319–322 (2017). https://doi.org/10.1016/j.matlet.2017.08.044
S.S. Karade, B.R. Sankapal, Two dimensional cryptomelane like growth of MoSe2 over MWCNTs: symmetric all-solid-state supercapacitor. J. Electroanal. Chem. 802, 131–138 (2017). https://doi.org/10.1016/j.jelechem.2017.08.017
H. Li, L. Chen, Y. Zhang, X. Ji, S. Chen, H. Song, C. Li, H. Tang, Synthesis of MoSe2/reduced graphene oxide composites with improved tribological properties for oil-based additives. Cryst. Res. Technol. 49, 204–211 (2014). https://doi.org/10.1002/crat.201300317
J. Sha, C. Gao, S.K. Lee, Y. Li, N. Zhao, J.M. Tour, Preparation of three-dimensional graphene foams using powder metallurgy templates. ACS Nano 10, 1411–1416 (2016). https://doi.org/10.1021/acsnano.5b06857
Z. Chen, W. Ren, L. Gao, B. Liu, S. Pei, H.M. Cheng, Three-dimensional flexible and conductive interconnected graphene networks grown by chemical vapour deposition. Nat. Mater. 10, 424–428 (2011). https://doi.org/10.1038/nmat3001
K. Chen, C. Li, Z. Chen, L. Shi, S. Reddy, H. Meng, Q. Ji, Y. Zhang, Z. Liu, Bioinspired synthesis of CVD graphene flakes and graphene-supported molybdenum sulfide catalysts for hydrogen evolution reaction. Nano Res. 9, 249–259 (2016). https://doi.org/10.1007/s12274-016-1013-1
H.J. Qiu, Y. Guan, P. Luo, Y. Wang, Recent advance in fabricating monolithic 3D porous graphene and their applications in biosensing and biofuel cells. Biosens. Bioelectron. 89, 85–95 (2017). https://doi.org/10.1016/j.bios.2015.12.029
S. Mao, G. Lu, J. Chen, Three-dimensional graphene-based composites for energy applications. Nanoscale 7, 6924–6943 (2015). https://doi.org/10.1039/c4nr06609j
H. Bai, C. Li, X. Wang, G. Shi, On the gelation of graphene oxide. J. Phys. Chem. C 115, 5545–5551 (2011). https://doi.org/10.1021/jp1120299
L. Bao, T. Li, S. Chen, C. Peng, L. Li, Q. Xu, Y. Chen, E. Ou, W. Xu 3D Graphene frameworks/Co3O4 composites electrode for high-performance supercapacitor and enzymeless glucose detection. Small (2017). https://doi.org/10.1002/smll.201602077
E.G. Da Silveira Firmiano, A.C. Rabelo, C.J. Dalmaschio, A.N. Pinheiro, E.C. Pereira, W.H. Schreiner, E.R. Leite, Supercapacitor electrodes obtained by directly bonding 2D MoS2 on reduced graphene oxide. Adv. Energy Mater. 4, 1301380 (2014). https://doi.org/10.1002/aenm.201301380
K.J. Huang, J.Z. Zhang, Y. Fan, Preparation of layered MoSe2 nanosheets on Ni-foam substrate with enhanced supercapacitor performance. Mater. Lett. 152, 244–247 (2015). https://doi.org/10.1016/j.matlet.2015.03.130
S.K. Balasingam, J.S. Lee, Y. Jun, Molybdenum diselenide/reduced graphene oxide based hybrid nanosheets for supercapacitor applications. Dalton Trans. 45, 9646–9653 (2016). https://doi.org/10.1039/c6dt00449k
J. Chen, B. Yao, C. Li, G. Shi, An improved Hummers method for eco-friendly synthesis of graphene oxide. Carbon 64, 225–229 (2013). https://doi.org/10.1016/j.carbon.2013.07.055
X. Liu, J.Z. Zhang, K.-J. Huang, P. Hao, Net-like molybdenum selenide–acetylene black supported on Ni foam for high-performance supercapacitor electrodes and hydrogen evolution reaction. Chem. Eng. J. 302, 437–445 (2016). https://doi.org/10.1016/j.cej.2016.05.074
Y. Liu, T. Gao, H. Xiao, W. Guo, B. Sun, M. Pei, G. Zhou, One-pot synthesis of rice-like TiO2/graphene hydrogels as advanced electrodes for supercapacitors and the resulting aerogels as high-efficiency dye adsorbents. Electrochim. Acta 229, 239–252 (2017). https://doi.org/10.1016/j.electacta.2017.01.142
J. Yao, B. Liu, S. Ozden, J. Wu, S. Yang, M.T.F. Rodrigues, K. Kalaga, P. Dong, P. Xiao, Y. Zhang, R. Vajtai, P.M. Ajayan, 3D nanostructured molybdenum diselenide/graphene foam as anodes for long-cycle life lithium-ion batteries. Electrochim. Acta 176, 103–111 (2015). https://doi.org/10.1016/j.electacta.2015.06.138
S. Sankar, A.I. Inamdar, H. Im, S. Lee, D.Y. Kim, Template-free rapid sonochemical synthesis of spherical α-MnO2 nanoparticles for high-energy supercapacitor electrode. Ceram. Int. 44, 17514–17521 (2018). https://doi.org/10.1016/j.ceramint.2018.05.207
J.K. Jayaramulu, D.P. Dubal, B. Nagar, V. Ranc, O. Tomanec, M. Petr, K.K.R. Datta, R. Zboril, P. Gomez-Romero, R.A. Fischer, Ultrathin hierarchical porous carbon nanosheets for high-performance supercapacitors and redox electrolyte energy storage. Adv. Mater. 30, 1705789 (2018). https://doi.org/10.1002/adma.201705789
J. Zhao, Y. Jiang, H. Fan, M. Liu, O. Zhuo, X. Wang, Q. Wu, L. Yang, Y. Ma, Z. Hu, Porous 3D few-layer graphene-like carbon forultrahigh-power supercapacitors with well-defined structure-performance relationship. Adv. Mater. 29, 1604569 (2017). https://doi.org/10.1002/adma.201604569
X. Gao, H. Yue, E. Guo, L. Yao, X. Lin, B. Wang, E. Guan, D. Bychanok, In-situ polymerization growth of polyaniline nanowire arrays on graphene foam for high specific capacitance supercapacitor electrode. J Mater. Sci. Mater. Electron. 28, 17939–17947 (2017). https://doi.org/10.1007/s10854-017-7736-2
S. Ahmed, M. Rafat, Hydrothermal synthesis of PEDOT/rGO composite for supercapacitor applications. Mater. Res. Express (2017) https://doi.org/10.1088/2053-1591/aaa232
S. Ahmed, M. Rafat, Effect of lithium and sodium salt on the performance of Nb2O5/rGO nanocomposite based supercapacitor. Mater. Res. Express (2018). https://doi.org/10.1088/2053-1591/aaace2
A. Sultan, R. Mohd, M.K. Singh, S. Hashmi, A Free-standing, flexible PEDOT-PSS film and its nanocomposites with graphene nano-platelets as electrodes for quasi-solid-state supercapacitors. Nanotechnology (2018). https://doi.org/10.1088/1361-6528/aad0b8
Y. Yang, F. Huilong, R. Gedeng, X. Changsheng, J.M. Tour, Edge-oriented MoS2 nanoporous films as flexible electrodes for hydrogen evolution reactions and supercapacitor devices. Adv. Mater. 26, 8163–8168 (2014). https://doi.org/10.1002/adma.201402847
W. Lu, M. Arif, G. Duan, S. Chen, X. Liu, A high performance quasi-solid-state supercapacitor based on CuMnO2 nanoparticles. J. Power Sour. 355, 53–61 (2017). https://doi.org/10.1016/j.jpowsour.2017.04.054
P. Hui, J. Zhou, K. Sun, G. Ma, Z. Zhang, E. Feng, Z. Lei, High-performance asymmetric supercapacitor designed with a novel NiSe@MoSe2 nanosheet arrays and nitrogen-doped carbon nanosheet. Acs Sustaina Chem Eng 5, 59514–59563 (2017). https://doi.org/10.1021/acssuschemeng.7b00729
S.N. Tiruneh, B. Kang, S.H. Kwag, Y.H. Lee, M.S. Kim, D.H. Yoon, Synergistically active NiCo2S4 nanoparticles coupled with holey defect graphene hydrogel for high-performance solid-state supercapacitors. Chem. Eur. J. 24, 3263–3270 (2018). https://doi.org/10.1002/chem.201705445
K. Ghosh, C.Y. Yue, Development of 3D MoO3/graphene aerogel and sandwich-type polyaniline decorated porous MnO2-graphene hybrid film based high performance all-solid-state asymmetric supercapacitors. Electrochim. Acta 276, 47–63 (2018). https://doi.org/10.1016/j.electacta.2018.04.16
Acknowledgements
This work is supported by the fundamental research foundation for University of Heilongjiang province (Grant No. LGYC2018JQ012), the Innovative Talent Fund of Harbin city (Grant No. 2016RAQXJ185).
Author information
Authors and Affiliations
Corresponding author
Additional information
Publisher’s Note
Springer Nature remains neutral with regard to jurisdictional claims in published maps and institutional affiliations.
Electronic supplementary material
Below is the link to the electronic supplementary material.
Rights and permissions
About this article
Cite this article
Wang, Z., Yue, H.Y., Yu, Z.M. et al. One-pot hydrothermal synthesis of MoSe2 nanosheets spheres-reduced graphene oxide composites and application for high-performance supercapacitor. J Mater Sci: Mater Electron 30, 8537–8545 (2019). https://doi.org/10.1007/s10854-019-01174-7
Received:
Accepted:
Published:
Issue Date:
DOI: https://doi.org/10.1007/s10854-019-01174-7