Abstract
An 8-week feeding trial was conducted to evaluate the effects of dietary carbohydrate to lipid (CHO:L) ratios on growth performance, body composition, serum biochemical indexes, lipid metabolism, and gene expression of central appetite regulating factors in Chinese perch (Siniperca chuatsi) (mean initial weight: 12.86 ± 0.10 g). Five isonitrogenous and isoenergetic diets (fish meal, casein as main protein sources) were formulated to contain different graded CHO:L ratio diets ranging from 0.12, 0.86, 1.71, 3.29, and 7.19. Each diet was assigned to triplicate groups of 18 experimental fish. Our results revealed that final body weight (FBW), weight gain rate (WGR), specific growth rate (SGR), and protein efficiency ratio (PER) increased with dietary CHO:L ratio from 0.12 to 1.71 and then decreased with further increases in dietary CHO:L ratio. A two-slope broken-line regression analysis based on WGR showed that the optimal dietary CHO:L level for maximum growth performance of fish was 1.60. Crude lipid and crude protein content in the liver and glycogen concentration in the muscle and liver were significantly influenced by the dietary CHO:L ratios (P < 0.05). The lowest crude lipid content in the liver was observed in fish fed the diet with a CHO:L ratio of 1.71(P < 0.05). Dietary CHO:L ratios significantly induced the glucose concentration of serum (P < 0.05). The relative expression levels of genes involved in lipid metabolism, such as srebp1 and fas in the liver, showed a trend of first decreased and then increased with the increase of dietary CHO:L ratio levels. Appropriate CHO:L ratio in the diet can effectively reduce the accumulation of liver fat. We observed in fish fed the 1.71 CHO:L ratio diet showed higher feed intake, up‐regulated mRNA expression of neuropeptide Y (npy) and agouti gene-related protein (agrp), and down‐regulated mRNA expression of cocaine- and amphetamine-regulated transcript (cart) and pro‐opiomelanocorticoid (pomc) significantly as compared to control group. Thus, these results provide the theoretical basis for feed formulation to determine the appropriate CHO:L ratio requirement of Chinese perch.
Similar content being viewed by others
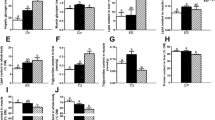
Explore related subjects
Discover the latest articles, news and stories from top researchers in related subjects.Avoid common mistakes on your manuscript.
Introduction
Over the past three decades, the growth rate of global aquaculture production is significantly higher than that of fishing, and it is the fastest growing industry in the global agricultural sector. Fish meal has become an important means to meet the demand for protein of aquatic animals and land animals. However, the demand for human consumption of fish is also increasing, resulting in high costs and fluctuations in fishmeal supply (FAO 2018; Montoya‐Camacho et al. 2019). To address this issue, in addition to constantly seeking high-quality protein sources that can replace fish meal, researchers are also improving feed formulation to save protein and improve feed protein efficiency (Turchini et al 2009).
Lipids and carbohydrates are one of the most important nutrients for organisms, and carbohydrates are a relatively inexpensive source of energy (Gao et al 2018). Previous results demonstrate that appropriate lipid and carbohydrate levels in diets can provide energy to organisms and have the effect of saving protein(Li, et al 2019a, b, c, d; Misra et al 2005; Taj et al 2020; Zhao et al 2020). Lipids provide essential fatty acids for the growth and development of fish, are the main structure of cell membranes, and facilitate the transport and absorption of fat-soluble vitamins in the body(Ding et al 2020; Xu et al 2020). Carbohydrates also have good adhesion and expandability, which can improve the stability of feed in water (Lin et al 2018). Excessive lipid will cause a series of problems such as physiological metabolic disorders, poor growth, and fatty liver (Lu et al 2014; Zhou et al 2020). Fish are also born with diabetes, especially carnivorous fish (Wilson 1994). Excessive carbohydrate level will lead to excessive metabolic burden, long-term hyperglycemia, excessive accumulation of liver glycogen, decreased immune function, and other obstacles (Kamalam et al 2016; Lin et al 2018).
In fish, glucose is closely related to lipid metabolism. Dihydroxyacetone phosphate produced in the process of glycolysis is then converted into glycerol needed for fat synthesis. Acetyl CoA generated from glucose oxidative decomposition is also a precursor for fatty acid synthesis, which can promote the generation of fat in the body (Zhang et al 2020). Dietary lipid can also be converted to glucose by gluconeogenesis (Honorato et al 2010).
Fish appetite is co-regulated by the central system and the peripheral system, through the signal transduction between various appetite regulating factors (including appetite promoting factors and appetite suppressant factors), the “appetite regulating network” of fish is formed (Volkoff et al 2004; Yokobori et al 2012). Food intake is closely related to appetite, and there is evidence in humans, other mammals, and fish that the intake of a high-fat diet can suppress appetite and reduce sensitivity to the present food (Li et al 2016; Ortinau et al 2014; Rasmussen et al 2000; Tantot et al. 2017). In bony fish, fed high carbohydrate diet or injecting glucose intraperitoneally caused rainbow trout (Oncorhynchus mykiss) (Figueiredo-Silva et al 2013), European sea bass (Dicentrarchus labrax) (Castro et al 2015), and Japanese flounder (Paralichthys olivaceus) (Liu et al 2018) decreased appetite and food intake. In recent years, researchers have made a high number of studies on the effects of dietary CHO:L ratio on growth performance, feed utilization, and antioxidant capacity of fish (Gao et al 2010; Li et al 2012; Zhou et al 2016). Nevertheless, there are few studies on the effects of different dietary CHO:L ratios on lipid metabolism and appetite of fish.
The Chinese perch (Siniperca chuatsi), which is mainly distributed in China, Russia, Korea, Vietnam, and other regions, is one of the unique freshwater aquaculture fish with great economic value in China (Liu et al 2020). It is a typical carnivorous fish with fierce feeding habits. It hunts live prey fish from its mouth-opening and could stably accept artificial compound feed after domestication (Liang et al 2010, 2001). Currently, the protein content in the commercial feed of Chinese perch is extremely high, so changing the CHO:L ratios in the diet to improve the utilization rate of protein of Chinese perch, reduce the content of fish meal, and thus reduce the feed cost is of great significance for intensive farming. In fact, there are relatively few studies on nutritional requirements of Chinese perch. This study aims to explore the effects of different dietary CHO:L ratios on growth performance, body composition, liver lipid metabolism, liver histomorphology, and appetite of Chinese perch. The results of this study may provide a basis for the development of high efficiency compound feed in the future.
Materials and methods
All animal care and experimental procedures in the present study were approved by Huazhong Agricultural University and conducted in accordance with the Guidelines for Experimental Animals (Ethical code: HZAUFI-2020–0004).
Experimental diets
The formulation and proximate composition of the experimental diets are provided in Table 1. A dietary protein level of 48% was used for this study based on the findings of previous research in our laboratory (Wang et al. 2018); five isonitrogenous and isoenergetic experimental diets were formulated to contain different levels of CHO:L ratio: 0.12 (D1), 0.86 (D2), 1.71 (D3), 3.29 (D4), and 7.19 (D5). Experimental diets were compounded with fish meal and casein as main protein sources, fish oil and soybean oil as main lipid sources, and dextrin as a carbohydrate source. All dry ingredients were thoroughly mixed in a mixer before the addition of oil and 40% water. Then, the mixture was then pelleted (4 mm diameter) by a laboratory pelleting machine (HR 2330 model, PHILIPS, Suzhou, China). The soft pellets were placed in hermetic bags were stored in a freezer at − 20 °C until used.
Experimental fish and feeding management
The experiment was conducted in an indoor aquarium system at the Chinese Perch Research Center, Huazhong Agricultural University, Wuhan, China. About 500 Chinese perch were purchased from Wuhongshan Breeding Base (Chibi, China). Before the feeding experiment, Chinese perch were trained to accept the artificial diet according to the method of Liang et al. (Liang et al 2001). After 2 weeks of acclimatization, 270 fish (mean initial weight: 12.86 ± 0.10 g) with uniform size accept artificial diet well and health and were randomly assigned to fifteen 400 l round fibreglass tanks (80 cm diameter × 80 cm height) with 18 fish per tank. Five feeds with different CHO:L ratio were weighed daily (3–5% total weight of per tank all fish) and hand-fed twice (08:00 and 19:00) to five different treatment groups till satiation. The amount of feed being consumed by fish in each tank was recorded daily. Uneaten feed was collected by siphoning after 15-min feeding and then oven-dried at 60 °C to calculate feed intake. During the feeding period, approximately 25% of the water in each tank was replenished daily at 12:00 am. The water temperature, pH, and dissolved oxygen (DO) level were recorded daily, and their values were 19–23 °C, 7.1–7.5, and > 5 mg/l, respectively. Ordinary photoperiod applied throughout the experiment.
Sample collection and analysis
After 8 weeks of feeding trial, all fish were starved for 24 h and then counted individually and weighed. Six fish were randomly selected from each replicate were anaesthetized with 3-aminobenzoic acid ethyl ester methane sulfonate (MS-222, 50 mg/l water). The blood was obtained from caudal vein of fish by syringe using a 1-ml syringe and pooled into a sterile centrifuge tube to clot overnight at 4 °C and then centrifuged at 4000 × g at 4 °C for 20 min to separate the serum and stored at – 80 °C until used for analysis. Then these fish were dissected on ice to obtain brain, muscle, liver, intestine, mesentery, and visceral adipose, quickly frozen in liquid nitrogen and cryopreserved at − 80 °C for subsequent analysis. Liver tissues from three fishes from each group were collected and immediately fixed by 4% paraformaldehyde for histological evaluation. Another 3 fish were randomly chosen from each tank for whole‐body composition.
Growth parameters
The data were analysed for weight gain rate (WGR), specific growth rates (SGR), hepatosomatic index (HSI), viscera index (VSI), mesentery fat index (MFI), survival ratio (SR), feed intake (FI), and food conversion ratio (FCR) using the following formula:
Proximate composition
Crude protein, crude lipid, ash and moisture of diets, whole body, muscle, and liver were measured according to standard Association of Official Agricultural Chemists methods (Hirwitz and Latimer 1995). Crude protein content was determined by measuring nitrogen (N × 6.25) levels using the Kjeldahl method following acid digestion with an auto Kjeldahl System (Kjelflex K-360, BUCHI Labortechnik AG, Flawil, Switzerland). Crude lipid was determined by using the Soxhlet extraction procedure. Moisture content was measured by freeze-drying samples for 48 h in a vacuum freeze dryer (Christ Beta 2–4 LD plus LT, Marin Christ Corporation, Osterode, Germany). Crude ash content was examined through incineration at 550 °C for 24 h in a muffle furnace. The crude fibre was analysed by the fritted glass crucible method using an automatic analyser (FT12, Gerhardt, Germany).
Serum biochemical analysis
Serum total protein (TP), albumin (ALB), glucose (GLU), total cholesterol (TCHO), triglycerides (TG), high-density lipoprotein (HDLC), and low-density lipoprotein (LDLC) contents, along with aspartate transaminase (AST) and alanine transaminase (ALT) activities, were determined using an automatic biochemical analyser (CHEMIX-800, Sysmex Corporation, Kobe, Japan) with commercial diagnostic reagent kits (Sysmex Wuxi Co. Ltd., Wuxi, China).
Analysis of genes expression
Total RNA was extracted by RNAiso Plus reagent (Takara, Dalian, China) manually, and the purity and quantity of RNA were determined by agarose gel electrophoresis, protein, and nucleic acid analyser. Then 1 μg of total RNA was used for reverse transcription with HiScript® II Reverse Transcriptase (Code no. R301–01/02; Vazyme, China) in a 20 μl reaction volume to synthesize cDNA. Specific primers for the candidate genes used for qPCR were designed based on previous published research paper of our laboratory (Table 2). Rpl13a gene was used as an endogenous reference to normalize the template amount. The total volume of the qRT-PCR reaction system was 20 μl, including 10 μl of SYBR Green dye (Code no. Q311–02; Vazyme, China), 0.4 μl of PCR forward/re-verse primers (10 μM), 1 μl of cDNA template, and 8.2 μl RNase-free H2O. The qRT-PCR amplification program was 95 °C for 1 min, followed by 40 cycles consisting of 95 °C for 10 s and 57 °C for 30 s and a melt curve step (from 95 °C, gradually reducing 0.5 °C/s to 57 °C, with data acquisition every 6 s). The amplification efficiencies of control and target genes were approximately equal and ranged from 96.3 to 104.9%. Gene expression levels were quantified relative to the expression of rpl13a using the optimized comparative Ct (2-ΔΔCt) value method. All amplifications were performed in triplicate for each RNA sample.
Liver histopathological examination
According the standard methods at Wuhan Google Biological Technology Co., Ltd. (Wuhan, China), liver tissues from three fishes from each group were collected and immediately fixed by using 4% neutral buffered formaldehyde for 48 h. After dehydrated and imbedded into paraffin, a tissue section was cut into 5 μm for hematoxylin and eosin (H&E) staining. For the frozen section and Oil Red O staining, liver samples were immediately frozen with liquid nitrogen and stored at − 80 °C. Serial frozen sections were cut into 8 μm for Oil Red O staining.
Statistical analysis
The normality of the data was tested by a single sample T test. And homogeneity of all data was tested before one-way analysis of variance (ANOVA). The data were expressed as mean ± SEM (standard error of the mean) and were analysed by ANOVA. A multiple comparison test (Duncan’s new multiple range test) was conducted to compare the significant differences among groups. Differences were considered significant at P ≤ 0.05. The dietary optimum CHO:L ratio of Chinese perch was estimated by the broken-line regression. Statistical analysis was conducted by using the SPSS 26.0 (SPSS, Chicago, IL, USA).
Results
Growth performance and feed utilization
As shown in Table 3, SR did not show significant difference among fish fed the different dietary treatments (P > 0.05). Fish fed D3 had the highest FBW, WGR, and SGR (P < 0.05). PER in fish fed D3 was significantly higher than that in fish fed other diets (P < 0.05). FI was significantly affected by the dietary CHO:L ratios (P < 0.05), fish fed D3 was significantly higher than D1, D2, and D4 groups. HSI and VSI in the D1 were significantly higher than that in the other groups (P < 0.05). The MFI of D4 and D5 groups were significantly lower than that of D1, D2, and D3 groups (P < 0.05). FCR exhibited an opposite trend as observed in WGR, with the lowest level in fish fed D3 group (P < 0.05).
Based on broken-line regression analysis of WGR, the dietary CHO:L ratio for optimum growth of Chinese perch was 1.60, corresponding to 15.38% of nitrogen-free extract and 8.97% of crude lipid respectively, belongs to the D3 group (Fig. 1).
Proximate composition and glycogen content in tissues
Effects of dietary CHO:L ratios on whole body, proximate composition and glycogen content of Chinese perch are listed in Table 4. The lipid content of whole body trended downward as dietary CHO:L ratios increased. There were no significant differences in moisture, crude protein, and crude lipid contents of the muscle among all treatments (P > 0.05). Dietary CHO:L ratios significantly affected the contents of crude protein and lipid in liver of Chinese perch (P < 0.05). Liver crude protein increased in D2, D3, and D5 groups compared with D1 group. Fish fed D3 had lower crude lipid content in liver than other groups and significantly lower than D1 and D4 groups (P < 0.05).
The muscle glycogen and liver glycogen had an increase trend with the increasing CHO:L ratio level. The highest muscle glycogen content was observed in D5 group and significantly higher than D1, D2, and D3 groups. There was no significant difference in liver glycogen content among D3, D4, and D5 groups (P > 0.05).
Serum biochemical indices
Dietary CHO:L ratios significantly induced the contents of GLU (Table 5). GLU in fish fed D1 and D2 were significantly lower than that in fish fed other diets (P < 0.05). TP and ALB were significantly influenced by dietary CHO:L ratios, and it was the lowest in D3 among all groups and was significantly lower than that in D1, D2, and D4 (P < 0.05). TCHO in fish fed D1 and D2 were significantly higher than other groups (P < 0.05). As dietary CHO:L ratio increased, serum TG content was significantly decreased (P < 0.05). The highest concentration of TG was found in fish fed a diet with the lowest CHO:L ratio. The activities of AST and ALT both increased with dietary CHO:L ratios, showing a trend of first decreased and then increased, and reached the lowest in D3 group (P < 0.05). As dietary CHO:L ratio increased, serum HDLC content was significantly decreased (P < 0.05). On the contrary, LDLC was not significantly influenced by dietary CHO:L ratio (P > 0.05).
Relative expression of lipid metabolism-related genes in the liver
The expressions of lipid metabolism-related genes in Chinese perch fed different dietary CHO:L ratio levels are presented in Fig. 2. The expression of srebp1 and fas in the liver showed a trend of first decreased and then increased with the increase of dietary CHO:L ratios. And the expressions of srebp1 and fas in the liver were the lowest in the D3 group, which was significantly lower than the other groups (P < 0.05). Compared with the D1 group, the expression of accα in the liver of the other groups was significantly reduced, and there was no significant difference (P > 0.05). The expression level of cpt-1 in the liver of D3 and D5 group was significantly higher than that of D1 group (P < 0.05) (Fig. 3).
The mRNA expression levels of genes involved in lipid synthesis (srebp-1, sterol regulatory element-binding proteins-1; fas, fatty acid synthase; accα, acetyl-coA carboxylase alpha). Values are presented as the means ± SEM (n = 6). Different lowercase letters above the bars indicate significant difference (P < 0.05)
Relative expression of appetite-related genes in hypothalamus
Concerning appetite regulation-related genes are presented in Fig. 4 and Fig. 5. Compared with the D1 group, the expression of npy in the D2 and D3 groups was significantly increased (P < 0.05). The expression of agrp increased with the increase in the level of dietary CHO:L ratios, showing a trend of first increased and then decreased. The expression levels of npy and agrp were the highest in the D3 group and were significantly higher than the other groups (P < 0.05). With the increase of dietary CHO:L ratios, the expression of cart decreased significantly compared with the D1 group (P < 0.05). The expression of pomc in the D2 and D3 groups were significantly lower than the other groups (P < 0.05).
The mRNA expression levels of genes involved in appetite-inhibiting genes in the hypothalamus (cart, cocaine-and amphetamine-regulated transcript; pomc, proopiomelanocortin). Values are presented as the means ± SEM (n = 6). Different lowercase letters above the bars indicate significant difference (P < 0.05)
Histology analyses of liver section and determination of hepatocyte inflammation
Figure 6 shows the Oil Red O staining and H&E staining of Chinese perch liver tissue fed with different levels of dietary CHO:L ratios diet. The Oil Red O staining confirmed that the number of red dots (lipid droplets) has exhibited no obvious difference among D2 and D4 group, but it increased sharply in D1 and D5 group. The D3 group had the least number of lipid droplets (Fig. 6A). H&E staining confirmed that except for the D3 group, the liver cells of the other treatment groups showed more different numbers of small vacuoles, and the hepatocyte nuclei were squeezed to the edge (Fig. 6B). This means that the liver cells have different degrees of pathological reactions.
Histology analyses of liver section and determination of hepatocyte inflammation. A Hepatic tissue Sect. (40 × magnification) of Oil Red O staining of Chinese perch fed with dietary different CHO:L level. B Hepatic tissue Sect. (40 × magnification) of hematoxylin and eosin staining (H&E) of Chinese perch fed with dietary different CHO:L level. Lipid droplets appear red after staining Oil Red O, and the depth of color of the red stain and the amount of the lipid droplet were positively correlated with lipid content. The nuclei of hepatocyte appear blue and the vacuole present to be hyaline after staining hematoxylin and eosin, and the numbers of nuclei were negatively correlated with hepatic steatosis. Ld, lipid droplet; Nu, nuclei; Va, vacuole
Discussion
In the present study, we provided direct evidence that dietary CHO:L ratio of 1.71 advanced the growth performance of Chinese perch. At this point, the WGR, SGR, and PER of the Chinese perch reach the maximum. When the dietary CHO:L ratio was 0.12, the WGR, SGR, and PER were all lower than those of the other groups. This indicates that a relatively low or high CHO:L ratio diet not only depressed Chinese perch growth but also caused their poor feed utilization. Protein utilization efficiency can be improved by adjusting the ratio of carbohydrates to lipids in the feed (Jobling 2012). Appropriate CHO:L ratio was helpful for fish to exert synergistic effect in the utilization of carbohydrate and lipid, improve the utilization rate of feed, and thus promote growth. Such as large yellow croaker (Larimichthys crocea) (Zhou et al 2016), juvenile hybrid grouper (Epinephelus fuscoguttatus ♀ × Epinephelus lanceolatus ♂) (Gao et al 2018), juvenile cobia (Rachycentron canadum) (Zhao et al 2020), and juvenile black seabream (Acanthopagrus schlegelii) (Sehrish et al. 2020). 0.12 CHO:L group showed poor growth, indicating that high-lipid/low-carbohydrate group was not conducive to its growth, and its VSI and HSI were higher than those in other groups, indicating that higher dietary lipid level would affect lipid deposition in viscera and the liver (Zhou et al 2020). Polyline regression analysis based on WGR revealed that the dietary CHO:L requirements of Chinese perch could be satisfied if the CHO:L ratio reached 1.60. Growth, feed efficiency, and protein utilization ratio suggest that carbohydrates are a better digestible energy source for Chinese perch compared with lipids and can save protein. Although the carbohydrate utilization capacity of carnivorous fish is weak, the carbohydrate utilization level of fish is a result of interactions with the physical state of dietary starch, molecular complexity, glucose tolerance, environment, temperature, and other nutrient elements in the feed (Brauge et al 1995; Hemre et al. 2002; Li et al 2019a, b, c, d).
In this study, dietary CHO:L ratio had no significant effect on muscle composition but had significant effect on crude lipid content of whole fish and liver. The lipid content of whole fish generally decreases and the lipid content of liver a decreasing trend first and then increasing trend in response to increasing dietary CHO:L ratio in feed. It indicates that the crude lipid content of whole body is positively correlated with the dietary lipid level, and excessive lipid will be deposited in fish body. However, fish have a poor ability to utilize carbohydrate. When the high-carbohydrate/low-lipid, fish may decompose part of the body lipid for energy supply, such as the lipid in muscle. Similar results were found in large yellow croaker (Li, et al 2019a, b, c, d) and golden pompano (Trachinotus ovatus) (Dong et al 2018). In the liver, both high lipid levels and high carbohydrate levels contribute to fat deposition. These results were similar to those previously reported in largemouth bass (Micropterus salmoides) (Zhou et al 2020) and blunt snout bream (Megalobrama amblycephala) (Wang et al 2017).
The blood index parameters could indicate the physiological and health status of fish and could change dynamically with the nutritional status of fish (Fazio 2019).In this study, TG, TCHO, and HDLC in serum were significantly decreased with the increase of CHO:L ratio in the diet, indicating that endogenous lipid transport in fish was also more active under the high lipid diet. Metabolites rich in TG and CHO in liver were transported to other abdominal organs along with blood circulation, resulting in the increase of VSI. The serum GLU content was significantly increased with the dietary CHO:L ratio increasing. Liver glycogen significantly increased in the dietary CHO:L of 0.12 to 1.71 and then leveled off. The results show that the Chinese perch could use a certain level of carbohydrate (< 15.38%) in feed and store it in liver in the form of liver glycogen. However, the Chinese perch still cannot make good use of carbohydrate. When the dietary carbohydrate level is higher than 15.38%, the serum GLU content increases continuously, leading to hyperglycemia and partial glycogen storage in the muscle. Similar results have been observed in some carnivorous fish (Ren et al 2011; Zhou et al 2016). Serum total protein content is a major indicator of physiological health of fish (Alexander et al 2011). The albumin and globulin contained in it are generally considered to play an important role in the innate immune response of fish (Wiegertjes et al 1996). ALT and AST are considered sensitive indicators of normal tissue function and are often used to determine whether the liver has been damaged (Li et al 2012; Lu et al 2020). In this study, when dietary CHO:L ratio was 1.71, TP, ALB, ALT, and AST were significantly lower than those in other groups. These results indicate that high dietary fat or carbohydrate levels will affect the health of the fish, increase the burden on the liver, and thus activate the non-specific immunity of the fish. In some previous studies, such as European sea bass (SitjÀ-Bobadilla and PÉRez-SÁNchez 1999) and blunt snout bream(Zhou et al 2013) have been reported.
Lipid deposition in the liver is a comprehensive result of lipid uptake, transport, decomposition, and synthesis in hepatocytes (Shearer et al 2012; Lu et al 2020). srebp-1 is a transcription factor that can activate target genes of its downstream fatty acid synthesis (Kuipers et al 2011). fas and accα are important enzymes in fat synthesis, which are involved in the synthesis of fatty acids (Castro et al 2016). In this study, srebp-1 and fas showed a trend of decreasing first and then increasing with the increase of dietary CHO:L ratio, indicating that fatty acid synthesis in the liver was more active in the diet of high-lipid/low-carbohydrate or high-carbohydrate/low-lipid. In addition, the increase of dietary carbohydrate level may also be the reason for the increase of liver srebp-1, which can also mediate the conversion of excess carbohydrates to fatty acids (Ferré and Foufelle 2010; Egea et al 2008); fatty acids enter the liver and are esterified into triglycerides, which are stored in lipid droplets on the one hand and enter liver cells. On the other hand, as TG-rich metabolites, they are secreted into blood for circulation (Yuan et al 2016). However, serum TCHO, TG, and LDLC levels did not increase in the high-carbohydrate/low-lipid group (D4 and D5), indicating that fatty acids may mainly exist in liver lipid droplets, which may cause harm to the health of fish. And cpt-1 is the key gene of fatty acid β oxidation. The cpt-1 in the liver of Chinese perch was significantly up-regulated when the dietary CHO:L ratio is 1.71, indicating that the β-oxidation of fatty acids is more active under this nutritional state. At this time, liver fat was significantly lower than that of the other groups, indicating that the liver of Chinese perch can effectively alleviate liver fat accumulation by reducing fatty acid synthesis and increasing fatty acid β oxidation when feeding appropriate CHO:L ratio diet.
Feed is the main cost in aquaculture, so the feed intake is considerable important to the aquaculture of economic fish, and the feed intake is controlled by the central and peripheral appetite network system (Li et al 2019a, b, c, d; Conde-Sieira and Soengas 2016). In previous studies, fish’s appetite was influenced by the nutritional content of the feed and the proportion of different ingredients added. By Basto-Silva (Basto-Silva et al 2021), studies have shown that different dietary protein to energy ratio affects the feeding intake and appetite regulation of gilthead seabream (Sparus aurata). The central system, especially the hypothalamus, can sense the nutritional state of the body, regulate food intake and metabolism through different neural circuits, and maintain the energy homeostasis of the body (Blouet and Schwartz 2009). These circuits mainly include npy/agrp, pomc/cart neurons and central and peripheral endocrine factors that respond to circulating glucose, fatty acid, or amino acid levels, respectively (Efeyan et al 2015; Conde-Sieira and Soengas 2016; Mobbs et al 2005). In previous studies based on Chinese perch in our laboratory, central nervous system npy/agrp was generally used as an appetite promoting factor, while pomc/cart was used as an appetite suppressant factor (Liu et al 2020). In this study, with the increase of dietary CHO:L ratio, the food intake of Chinese perch in D3 group was significantly higher than that in other treatment groups. The expression of npy/agrp neurons in the hypothalamus of Chinese perch was firstly increased and then decreased in response to increasing CHO:L ratio in feed. However, the expression level of inhibitory appetite factor cart in D1 group was significantly higher than that in other treatment groups, and the expression level of pomc in D2 and D3 groups was significantly downregulated. These results indicated that high lipid or high carbohydrate diet could inhibit the expression of appetite promoting genes npy and agrp to some extent and promote the expression of appetite suppressing genes cart and pomc. In previous studies, high carbohydrate diets can induce European sea bass (Castro et al 2015), rainbow trout (Otero-Rodiño et al 2016), and Siberian sturgeon (Acipenser baerii) (Gong et al 2015) reduced food intake. High lipid diets also decrease food intake of fish (Dai et al 2018). Therefore, appropriate CHO:L ratio feed can promote appetite, which has a positive effect on increasing food intake to avoid anorexia in the process of breeding.
The liver is an indicator organ to measure the nutritional and physiological status of fish, and nutritional imbalance can cause changes in the histological status of the cells(Wang et al 2014). The determination of nutrient requirements cannot be based only on the growth indicators of experimental animals, but also on their health and pathological reactions (Kumar et al 2005). Previous studies have shown that excessive dietary fat and carbohydrate content can cause metabolic burden of fish, resulting in a series of pathological reactions such as hepatic hypertrophy, hepatic steatosis, apoptosis, hepatocyte irregular arrangement, and fatty liver (Ishak et al 2016; Lu et al 2013). In our study, it was found that when fed with different carbohydrate-lipid ratio diets, liver H&E staining sections indicated that the number of hepatic cell vacuoles in D3 group was lower than that in other groups and so was the number of lipid droplets in D3 group. This indicated that the liver health status was better when the carbohydrate-to-lipid ratio was 1.71.
Conclusion
In conclusion, through broken line regression analysis, the optimal CHO:L ratio in the diet of Chinese perch is recommended to be 1.60. The CHO:L ratio in the diet affected the growth and feeding of Chinese perch, the antioxidant indexes in serum, and the accumulation of glycogen in body. In addition, the appropriate CHO:L ratio ratio in the diet up-regulated or down-regulated the expression of lipid metabolism genes in the liver and reduced the lipid deposition in the liver. Appropriate CHO:L ratio affects the transcription level of hypothalamus appetite gene, thus increasing food intake. The results of this study can provide an important reference for the optimization of compound feed for Chinese perch.
Data availability
All data are available from the corresponding author by request.
Code availability
Not applicable.
References
Alexander C, Sahu NP, Pal AK, Akhtar MS (2011) Haemato-immunological and stress responses of Labeo rohita (Hamilton) fingerlings: effect of rearing temperature and dietary gelatinized carbohydrate. J Anim Physiol an N 95:653–663. https://doi.org/10.1111/j.1439-0396.2010.01096.x
Brauge C, Corraze G, Médale FO (1995) Effects of dietary levels of carbohydrate and lipid on glucose oxidation and lipogenesis from glucose in rainbow trout, Oncorhynchus mykiss, reared in freshwater or in seawater. Comp Biochem Phys A 111:117–124. https://doi.org/10.1016/0300-9629(95)98527-N
Basto-Silva C, Enes P, Oliva-Teles A, Balbuena-Pecino S, Navarro I, Capilla E, Guerreiro I (2021) Dietary protein source and protein/carbohydrate ratio affects appetite regulation-related genes expression in gilthead seabream (Sparus aurata). Aquaculture 533:736142. https://doi.org/10.1016/j.aquaculture.2020.736142
Blouet C, Schwartz GJ (2009) Hypothalamic nutrient sensing in the control of energy homeostasis. Behav Brain Res 209:1–12. https://doi.org/10.1016/j.bbr.2009.12.024
Castro C, Corraze G, Pérez-Jiménez A, Larroquet L, Cluzeaud M, Panserat S, Oliva-Teles A (2015) Dietary carbohydrate and lipid source affect cholesterol metabolism of European sea bass (Dicentrarchus labrax) juveniles. Brit J Nutr 114:1143–1156. https://doi.org/10.1017/S0007114515002731
Castro C, Corraze G, Basto A, Larroquet L, Panserat S, Oliva-Teles A (2016) Dietary lipid and carbohydrate interactions: implications on lipid and glucose absorption, transport in gilthead sea bream (Sparus aurata) juveniles. Lipids 51:743–755. https://doi.org/10.1007/s11745-016-4140-2
Conde-Sieira M, Soengas JL (2016) Nutrient sensing systems in fish: impact on food intake regulation and energy homeostasis. Front Neurosci-Switz 10:603. https://doi.org/10.3389/fnins.2016.00603
Dai YJ, Jiang GZ, Yuan XY, Liu WB (2018) High-fat-diet-induced inflammation depresses the appetite of blunt snout bream (Megalobrama amblycephala) through the transcriptional regulation of leptin/mammalian target of rapamycin. Brit J Nutr 120:1422–1431. https://doi.org/10.1017/S000711451800288X
Ding Z, Liu Y, Han J, Liu X, Zheng Y, Li W, Li Y (2020) Dietary lipid supplementation could significantly affect the growth, fatty acid profiles, and expression of PPARα, leptin, and adiponectin genes in juvenile genetically improved farmed tilapia. Eur J Lipid Sci Tech 123(1):2000207. https://doi.org/10.1002/ejlt.202000207
Dong LF, Tong T, Zhang Q, Wang QC, Xu MZ, Yu HR, Wang J (2018) Effects of dietary carbohydrate to lipid ratio on growth, feed utilization, body composition and digestive enzyme activities of golden pompano (Trachinotus ovatus). Aquacult Nutr 24:341–347. https://doi.org/10.1111/anu.12565
Efeyan A, Comb WC, Sabatini DM (2015) Nutrient-sensing mechanisms and pathways. Nature 517:302–310. https://doi.org/10.1038/nature14190
Egea M, Metón I, Córdoba M, Fernández F, Baanante IV (2008) Role of Sp1 and SREBP-1a in the insulin-mediated regulation of glucokinase transcription in the liver of gilthead sea bream (Sparus aurata). Gen Comp Endocr 155:359–367. https://doi.org/10.1016/j.ygcen.2007.06.018
Fao (2018) The State of World Fisheries and Aquaculture 2018: Meeting the Sustainable Development Goals United Nations.
Fazio F (2019) Fish hematology analysis as an important tool of aquaculture: a review. Aquaculture 500:237–242. https://doi.org/10.1016/j.aquaculture.2018.10.030
Ferré P, Foufelle F (2010) Hepatic steatosis: a role for de novo lipogenesis and the transcription factor SREBP-1c. Diabetes Obes Metab 12:83–92. https://doi.org/10.1111/j.1463-1326.2010.01275.x
Figueiredo-Silva AC, Saravanan S, Schrama JW, Panserat S, Kaushik S, Geurden I (2013) A comparative study of the metabolic response in rainbow trout and Nile tilapia to changes in dietary macronutrient composition. Brit J Nutr 109:816–826. https://doi.org/10.1017/S000711451200205X
Gao W, Liu YJ, Tian LX, Mai KS, Liang GY, Yang HJ, Huai MY, Luo WJ (2010) Effect of dietary carbohydrate-to-lipid ratios on growth performance, body composition, nutrient utilization and hepatic enzymes activities of herbivorous grass carp (Ctenopharyngodon idella). Aquacult Nutr 16:327–333. https://doi.org/10.1111/j.1365-2095.2009.00668.x
Gao Y, Luo Y, Li X, Dong Y, Liao Y, Yao W, Jin Z, Wu X (2018) Effects of dietary carbohydrate/lipid ratios on growth, feed utilization, hematology parameters, and intestinal digestive enzyme activities of juvenile hybrid grouper (brown-marbled grouper Epinephelus fuscoguttatus♀×giant grouper E. lanceolatus♂). N Am J Aquacult 80:418–426. https://doi.org/10.1002/naaq.10057
Gong G, Xue M, Wang J, Wu X-F, Zheng Y-H, Han F, Liang X-F, Su X-O (2015) The regulation of gluconeogenesis in the Siberian sturgeon (Acipenser baerii) affected later in life by a short-term high-glucose programming during early life. Aquaculture 436:127–136. https://doi.org/10.1016/j.aquaculture.2014.10.044
Hemre GI, Mommsen TP, Krogdahl, (2002) Carbohydrates in fish nutrition: effects on growth, glucose metabolism and hepatic enzymes. Aquacult Nutr 8:175–194. https://doi.org/10.1046/j.1365-2095.2002.00200.x
Hirwitz W, Latimer G (1995) Official methods of analysis of AOAC International (16th edn). Trends Food Sci Tech 6:382–382
Honorato CA, Almeida LC, Nunes CDS, Carneiro DJ, Moraes G (2010) Effects of processing on physical characteristics of diets with distinct levels of carbohydrates and lipids: the outcomes on the growth of pacu (Piaractus mesopotamicus). Aquacult Nutr 16:91–99. https://doi.org/10.1111/j.1365-2095.2008.00644.x
Ishak SD, Kamarudin MS, Ramezani-Fard E, Saad CR, Yusof YA (2016) Effects of varying dietary carbohydrate levels on growth performance, body composition and liver histology of Malaysian mahseer fingerlings (Tor tambroides). J Environ Biol 37:755–764
Jobling M (2012) National Research Council (NRC): Nutrient requirements of fish and shrimp. 20: 601–602. https://doi.org/10.1007/s10499-011-9480-6
Kamalam BS, Medale F, Panserat S (2016) Utilisation of dietary carbohydrates in farmed fishes: new insights on influencing factors, biological limitations and future strategies. Aquaculture 467:3–27. https://doi.org/10.1016/j.aquaculture.2016.02.007
Kuipers RS, Luxwolda MF, Sango WS, Kwesigabo G, Velzing-Aarts FV, DaJ D-B, FaJ M (2011) Postpartum changes in maternal and infant erythrocyte fatty acids are likely to be driven by restoring insulin sensitivity and DHA status. Med Hypotheses 76:794–801
Kumar S, Sahu NP, Pal AK, Choudhury D, Yengkokpam S, Mukherjee SC (2005) Effect of dietary carbohydrate on haematology, respiratory burst activity and histological changes in L. rohita juveniles. Fish Shellfish Immunol 19:331–344. https://doi.org/10.1016/j.mehy.2011.02.020
Li AX, Yuan XC, Liang X-F, Liu LW, Li J, Li B, Fang JG, Li J, He S, Xue M, Wang J, Tao Y-X (2016) Adaptations of lipid metabolism and food intake in response to low and high fat diets in juvenile grass carp (Ctenopharyngodon idellus). Aquaculture 457:43–49. https://doi.org/10.1016/j.aquaculture.2016.01.014
Li H, Xu W, Jin J, Zhu X, Yang Y, Han D, Liu H, Xie S (2019a) Effects of dietary carbohydrate and lipid concentrations on growth performance, feed utilization, glucose, and lipid metabolism in two strains of gibel carp. Front Vet Sci 6:165. https://doi.org/10.3389/fvets.2019.00165
Li L, Fang J, Liang XF, Alam MS, Liu L, Yuan X (2019b) Effect of feeding stimulants on growth performance, feed intake and appetite regulation of mandarin fish, Siniperca chuatsi. Aquac Res 50:3684–3691. https://doi.org/10.1111/are.14327
Li S, Yin J, Zhang H, Liu Z, Chen N (2019c) Effects of dietary carbohydrate and lipid levels on growth performance, feed utilization, body composition and non-specific immunity of large yellow croaker (Larimichthys crocea). Aquacult Nutr 25(5):995–1005. https://doi.org/10.1111/anu.12917
Li S, Sang C, Wang A, Zhang J, Chen N (2019d) Effects of dietary carbohydrate sources on growth performance, glycogen accumulation, insulin signaling pathway and hepatic glucose metabolism in largemouth bass, Micropterus salmoides. Aquaculture 513:734391–734391. https://doi.org/10.1016/j.aquaculture.2019.734391
Li XF, Liu WB, Lu KL, Xu WN, Ying W (2012) Dietary carbohydrate/lipid ratios affect stress, oxidative status and non-specific immune responses of fingerling blunt snout bream, Megalobrama amblycephala. Fish Shellfish Immunol 33:316–323. https://doi.org/10.1016/j.fsi.2012.05.007
Liang XF, Oku H, Ogata H, Liu J, He X (2001) Weaning Chinese perch Siniperca chuatsi (Basilewsky) onto artificial diets based upon its specific sensory modality in feeding. Aquac Res 32:76–82. https://doi.org/10.1046/j.1355-557x.2001.00006.x
Liang X, Liu J, Huang B, Liang X, Liu J, Huang B (2010) The role of sense organs in the feeding behaviour of Chinese perch. J Fish Biol 52:1058–1067. https://doi.org/10.1111/j.1095-8649.1998.tb00603.x
Lin SM, Shi CM, Mu MM, Chen YJ, Luo L (2018) Effect of high dietary starch levels on growth, hepatic glucose metabolism, oxidative status and immune response of juvenile largemouth bass, Micropterus salmoides. Fish Shellfish Immunol 78:121–126. https://doi.org/10.1016/j.fsi.2018.04.046
Liu D, Guo B, Han D, Deng K, Gu Z, Yang M, Xu W, Zhang W, and Mai K (2018) Comparatively study on the insulin-regulated glucose homeostasis through brain-gut peptides in Japanese flounder Paralichthys olivaceus after intraperitoneal and oral administration of glucose. Gen Comp Endocr 9-20https://doi.org/10.1016/j.ygcen.2018.02.013
Liu L, Fang J, Liang XF, He S (2020) Nucleotide promotes feed intake and protein utilization via regulating the gene expression of feeding and nitrogen metabolism in juvenile Chinese perch (Siniperca chuatsi). Aquacult Nutr 26:1702–1712. https://doi.org/10.1111/anu.13121
Lu KL, Xu WN, Li JY, Li XF, Huang GQ, Liu WB (2013) Alterations of liver histology and blood biochemistry in blunt snout bream Megalobrama amblycephala fed high-fat diets. Fisheries Sci 79:661–671. https://doi.org/10.1007/s12562-013-0635-4
Lu K-L, Xu W-N, Wang L-N, Zhang D-D, Zhang C-N, Liu W-B (2014) Hepatic β-oxidation and regulation of carnitine palmitoyltransferase (CPT) I in blunt snout bream Megalobrama amblycephala fed a high fat diet. PLoS One 9:93135. https://doi.org/10.1371/journal.pone.0093135
Lu X, Peng D, Chen X, Wu F, Jiang M, Tian J, Liu W, Yu L, Wen H, Wei K (2020) Effects of dietary protein levels on growth, muscle composition, digestive enzymes activities, hemolymph biochemical indices and ovary development of pre-adult red swamp crayfish (Procambarus clarkii). Aquacult Rep 18:100542. https://doi.org/10.1016/j.aqrep.2020.100542
Misra S, Sahu NP, Pal AK, Xavier B, Kumar S, Mukherjee SC (2005) Pre- and post-challenge immuno-haematological changes in Labeo rohita juveniles fed gelatinised or non-gelatinised carbohydrate with n-3 PUFA. Fish Shellfish Immunol 21:346–356. https://doi.org/10.1016/j.fsi.2005.12.010
Mobbs CV, Isoda F, Makimura H, Mastaitis J, Mizuno T, Shu I-W, Yen K, Yang X-J (2005) Impaired glucose signaling as a cause of obesity and the metabolic syndrome: the glucoadipostatic hypothesis. Physiol Behav 85:3–23. https://doi.org/10.1016/j.physbeh.2005.04.005
Montoya-Camacho N, Marquez-Ríos E, Castillo-Yáñez FJ, López JLC, López-Elías JA, Ruíz-Cruz S, Jiménez-Ruíz EI, Rivas-Vega ME, Ocaño-Higuera VM (2019) Advances in the use of alternative protein sources for tilapia feeding. Rev Aquacult 11:515–526. https://doi.org/10.1111/raq.12243
Ortinau LC, Hoertel HA, Douglas SM, Leidy HJ (2014) Effects of high-protein vs. high-fat snacks on appetite control, satiety, and eating initiation in healthy women. Nutr J 13:97. https://doi.org/10.1186/1475-2891-13-97
Otero-Rodiño C, Velasco C, Álvarez-Otero R, López-Patiño MA, Míguez JM, Soengas JL (2016) In vitro evidence supports the presence of glucokinase-independent glucosensing mechanisms in hypothalamus and hindbrain of rainbow trout. J Exp Biol 219:1750–1759. https://doi.org/10.1242/jeb.137737
Rasmussen RS, Ostenfeld TH, Rønsholdt B, and Mclean E (2000) Manipulation of end‐product quality of rainbow trout with finishing diets. Aquacult Nutr 6https://doi.org/10.1046/j.1365-2095.2000.00119.x
Ren MC, Ai QH, Mai KS, Ma HM, Wang XJ (2011) Effect of dietary carbohydrate level on growth performance, body composition, apparent digestibility coefficient and digestive enzyme activities of juvenile cobia, Rachycentron canadum L. Aquac Res 42:1467–1475. https://doi.org/10.1111/j.1365-2109.2010.02739.x
Shearer GC, Savinova OV, Harris WS (2012) Fish oil – how does it reduce plasma triglycerides? Bba-Rev Cancer 1821:843–851. https://doi.org/10.1016/j.bbalip.2011.10.011
SitjÀ-Bobadilla A, PÉrez-SÁnchez J (1999) Short communication diet related changes in non-specific immune response of European sea bass (Dicentrarchus labrax L.). Fish Shellfish Immunol 9:637–640. https://doi.org/10.1006/fsim.1999.0219
Tantot F, Parkes SL, Marchand AR, Boitard C, Naneix F, Laye S, Trifilieff P, Coutureau E, Ferreira G (2017) The effect of high-fat diet consumption on appetitive instrumental behavior in rats. Appetite 108:203–211. https://doi.org/10.1016/j.appet.2016.10.001
Taj S, Irm M, Jin M, Yuan Y, Andriamialinirina HJT, Zhou Q (2020) Effects of dietary carbohydrate to lipid ratios on growth performance, muscle fatty acid composition, and intermediary metabolism in juvenile black seabream (Acanthopagrus schlegelii). Front Physiol 11:507. https://doi.org/10.3389/fphys.2020.00507
Turchini GM, Torstensen BE, Ng WK (2009) Fish oil replacement in finfish nutrition. Rev Aquacult 1:10–57. https://doi.org/10.1111/j.1753-5131.2008.01001.x
Volkoff H, Canosa LF, Unniappan S, Cerdá-Reverter JM, Bernier NJ, Kelly SP, Peter RE (2004) Neuropeptides and the control of food intake in fish. Gen Comp Endocr 142:3–19. https://doi.org/10.1016/j.ygcen.2004.11.001
Wang BK, Liu WB, Xu C, Cao XF, Zhong XQ, Shi HJ, Li XF (2017) Dietary carbohydrate levels and lipid sources modulate the growth performance, fatty acid profiles and intermediary metabolism of blunt snout bream Megalobrama amblycephala in an interactive pattern. Aquaculture 481:140–153. https://doi.org/10.1016/j.aquaculture.2017.08.034
Wang LN, Liu WB, Lu KL, Xu WN, Cai DS, Zhang CN, Qian Y (2014) Effects of dietary carbohydrate/lipid ratios on non-specific immune responses, oxidative status and liver histology of juvenile yellow catfish Pelteobagrus fulvidraco. Aquaculture 426–427:41–48. https://doi.org/10.1016/j.aquaculture.2014.01.022
Wang J, Liang XF, He S, Li J, Huang K, Zhang YP, Huang D (2018) Lipid deposition pattern and adaptive strategy in response to dietary fat in Chinese perch (Siniperca chuatsi). Nutr Metab 15(1):1–11. https://doi.org/10.1186/s12986-018-0315-6
Wiegertjes GF, Stet RM, Parmentier HK, Muiswinkel WBV (1996) Immunogenetics of disease resistance in fish: a comparative approach. Dev Comp Immunol 20:365–381. https://doi.org/10.1016/S0145-305X(96)00032-8
Wilson RP (1994) Utilization of dietary carbohydrate by fish. Aquaculture 124:67–80. https://doi.org/10.1016/0044-8486(94)90363-8
Xu H, Han T, Li X, Wang J, Zheng P, Yin F, and Wang C (2020) Effects of dietary lipid levels on survival, growth performance, and antioxidant ability of the early juvenile Scylla paramamosain. Aquaculture 528https://doi.org/10.1016/j.aquaculture.2020.735559
Yokobori E, Azuma M, Nishiguchi R, Kang KS, Kamijo M, Uchiyama M, Matsuda K (2012) Neuropeptide Y stimulates food intake in the Zebrafish, Danio rerio. J Neuroendocrinol 24:766–773. https://doi.org/10.1111/j.1365-2826.2012.02281.x
Yuan XC, Liang X-F, Liu LW, Fang JG, Li J, Li AX, Cai WJ, Xue M, Wang J, Wang QC (2016) Fat deposition pattern and mechanism in response to dietary lipid levels in grass carp, Ctenopharyngodon idellus. Fish Physiol Biochem 42:1557–1569. https://doi.org/10.1007/s10695-016-0240-4
Zhang L, Liu W-B, Brown PB, Xu C, Shi H-J, Zheng X-C, Zhang L, He C, Huang Y-Y, Li X-F (2020) Utilization of raw and gelatinized starch by blunt snout bream Megalobrama amblycephala as evidenced by the glycolipid metabolism, glucose tolerance and mitochondrial function. Aquaculture 529:735603. https://doi.org/10.1016/j.aquaculture.2020.735603
Zhao H, Cao J, Chen X, Wang G, Hu J, Chen B (2020) Effects of dietary lipid-to-carbohydrate ratio on growth and carbohydrate metabolism in juvenile cobia(Rachycentron canadum). Anim Nutr 6:80–84. https://doi.org/10.1016/j.aninu.2019.11.010
Zhou C, Liu B, Ge X, Xie J, Xu P (2013) Effect of dietary carbohydrate on the growth performance, immune response, hepatic antioxidant abilities and heat shock protein 70 expression of Wuchang bream, Megalobrama amblycephala. J Appl Ichthyol 29:1348–1356. https://doi.org/10.1111/jai.12264
Zhou PP, Wang MQ, Xie FJ, Deng D-F, Zhou QC (2016) Effects of dietary carbohydrate to lipid ratios on growth performance, digestive enzyme and hepatic carbohydrate metabolic enzyme activities of large yellow croaker (Larmichthys crocea). Aquaculture 452:45–51. https://doi.org/10.1016/j.aquaculture.2015.10.010
Zhou YL, Guo JL, Tang RJ, Ma HJ, Lin SM (2020) High dietary lipid level alters the growth, hepatic metabolism enzyme, and anti-oxidative capacity in juvenile largemouth bass Micropterus salmoides. Fish Physiol Biochem 46:125–134. https://doi.org/10.1007/s10695-019-00705-7
Funding
This work was financially supported by China Agriculture Research System (CARS-46), the National Key R & D Program of China (2019YFD0900500) and the National Natural Science Foundation of China (31972809).
Author information
Authors and Affiliations
Contributions
D.P. and X-F L.: designed the experiments and draft the manuscript. D.P., F-R C., and H–X F.: performed the experiments. J. L., S-L T., K. L., and Q-W Z.: revised the manuscript.
Corresponding author
Ethics declarations
Ethics approval
All experiments and animal-handling procedures were approved by the Ethics Committee of the Institute of Laboratory Animal Centre, Huazhong Agriculture University (Ethical code: HZAUFI-2020–0004).
Consent to participate
Not applicable.
Consent for publication
All authors review and approve the manuscript for publication.
Conflict of interest
The authors declare no competing interests.
Additional information
Publisher's note
Springer Nature remains neutral with regard to jurisdictional claims in published maps and institutional affiliations.
Rights and permissions
About this article
Cite this article
Peng, D., Liang, XF., Chai, F. et al. Effects of dietary carbohydrate to lipid ratios on growth, biochemical indicators, lipid metabolism, and appetite in Chinese perch (Siniperca chuatsi). Fish Physiol Biochem 48, 101–116 (2022). https://doi.org/10.1007/s10695-021-01043-3
Received:
Accepted:
Published:
Issue Date:
DOI: https://doi.org/10.1007/s10695-021-01043-3