Abstract
The worldwide occurrence of cyanobacterial blooms makes it necessary to perform environmental risk assessment procedures to monitor the effects of microcytins on fish. Oxidative stress biomarkers are valuable tools in this regard. Considering that zebrafish (Danio rerio) is a common model species in fish toxicology and the zebrafish gill is potentially useful in screening waterborne pollutants, this study investigated the oxidative stress response in zebrafish gill exposed to subchronic microcystin-LR (MCLR) concentrations (2 or 20 μg/l) via measurement of toxin accumulation, protein phosphatase (PP) activity, and the antioxidant parameters (glutathione-S-transferase—GST; glutathione—GSH; superoxide dismutase—SOD; catalase—CAT; glutathione peroxide—GPx; glutathione reductase—GR), as well as levels of hydroxyl radical (OH) and lipid peroxidation (LPO). The results showed that after 30 days exposure, MCLR accumulated in zebrafish gill and MCLR exposure induced PP activity in gill. A linear inhibition of GST activity and GSH content was observed in the gills, revealing that they were involved in the first step of MCLR detoxification. The 2 μg/l MCLR treatment neglectably affected OH content and the antioxidant enzymes (SOD, CAT, GPx, and GR), however oxidative stress was induced under the 20 μg/l MCLR treatment in which an enhanced OH content and alterations of the antioxidant enzymes were observed in the treated gills, although both treatments exerted little effect on LPO level. The principal component analysis results indicated that the most sensitive biomarkers of MCLR exposure were GST and GSH in zebrafish gill. So, D. rerio could be regarded as a suitable bioindicator of MCLR exposure by measuring CAT, GR, GST, and GSH as biomarkers.
Similar content being viewed by others
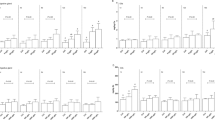
Explore related subjects
Discover the latest articles, news and stories from top researchers in related subjects.Avoid common mistakes on your manuscript.
Introduction
Over the past few decades, the occurrence of toxic cyanobacterial blooms in eutrophic rivers, lakes, reservoirs, and recreational waters has become a worldwide concern (Paerl et al. 2001). It is known that many species of cyanobacteria are able to produce cyanotoxins which are harmful or toxic to aquatic organisms, and animals including humans. Among cyanotoxins, microcystins (MCs) are considered to be one of the most toxic groups due to their potent hepatotoxicity and the fact that they are probable tumor promoters (Chorus and Bartram 1999). The group consists of more than 80 types with microcystin-LR (MCLR) as the most studied and the most toxic (Hoeger et al. 2005). MCs have caused mortality in animals and illness in both animals and humans (Carmichael and Falconer 1993), and they have been associated with fish kills (Andersen et al. 1993; Zimba et al. 2001). These threats have led the World Health Organization (WHO) to establish a provisional guideline value for MCLR in 1 μg/l of drinking water (WHO 1998).
The hepatotoxicity of MCs has extensively been investigated under both in vivo and in vitro conditions. In fish, both field and laboratory studies indicate that MCs accumulate in liver (Malbrouck et al. 2003, 2004; Xie et al. 2007), and also in other organs such as gill (Rodger et al. 1994; Zimba et al. 2001), and kidney (Williams et al. 1995; Mohamed et al. 2003). When MCs are absorbed and distributed into different organs, it might be biotransformed, which is elegantly exemplified by a previous study that the conversion of MCs in animal liver to a more polar compound is correlated with a depletion of the cellular glutathione (GSH) pool (Kondo et al. 1996). Pflugmacher et al. (1998) also show the existence of a MCLR-GSH conjugate formed enzymatically via soluble glutathione-S-transferase (GST) in various aquatic organisms ranging from plants (Ceratophyllum demersum), invertebrates (Dreissena polymorpha and Daphnia magna) up to fish eggs and fish (Danio rerio). This conjugation appears to be the first step in the detoxification of cyanobacterial toxins. Thus, the activity of GST has been utilized to point out MCs detoxification in several aquatic organisms like microalgae, macrophytes, invertebrates, and fish (Wiegand et al. 1999; Pflugmacher et al. 2001; Pietsch et al. 2001; Pflugmacher 2004; Pinho et al. 2005).
The typical toxicological action of MCs is to inhibit serine/threonine protein phosphatase (PP), followed by hyperphosphorylation of regulated proteins with two main consequences: the destruction of cytoskeleton directly causing the cytotoxic effects, and deregulation of cell division, leading to the tumor-promoting activity (Gehringer 2004). However, other mechanisms (e.g., oxidative damage) are also involved in the development of MCs toxicity (Ding et al. 2001; Li et al. 2003; Jos et al. 2005; Prieto et al. 2006). Oxidative stress is established when the pro-oxidant forces overwhelm the antioxidant defenses in cells, which comprises enzymatic and non-enzymatic mechanisms. Some of the most important antioxidant enzymes are superoxide dismutase (SOD), catalase (CAT), glutathione peroxidase (GPx), and glutathione reductase (GR); while the non-enzymatic defenses include GSH, vitamins E, C and A, etc. (Wilhelm Filho et al. 2000). These defenses can prevent the formation of reactive oxygen species (ROS) or intercept oxidative propagation reactions promoted by the ROS once formed (Bainy et al. 1996). Then, they can protect cells against DNA strand breaks, protein oxidation, and lipid peroxidation (LPO), the latter of which may cause loss of integrity of lipid membranes and generation of toxic aldehydes (Wiston and DiGiulio 1991).
Since many environmental pollutants display toxic effects related to oxidative stress, antioxidant enzyme activities (e.g., SOD, CAT, GPx and GR) are being studied as potential biomarkers in environmental risk assessment programs, together with LPO products such as aldehydes (van der Oost et al. 2003).
Although a great deal of attention has been paid to the toxic effects of MCLR on fish liver (Li et al. 2003; 2004; Malbrouck et al. 2003, 2004; Xie et al. 2007; Mezhoud et al. 2008a, b), their toxic effects on other organs (e.g., gill) have been neglected. The fish gill is a multifunctional organ performing vital functions such as respiration, osmoregulation, acid–base balance and nitrogenous waste excretion (Evans et al. 2005). Gills are always in close contact with the water, which makes them significant targets for waterborne pollutants. Paradoxically, they are highly vulnerable to toxic contaminants because firstly, their large surface area facilitates greater toxic interaction and absorption and secondly, their detoxification system is not as robust as that of liver (Mallatt 1985). Gills, therefore, are potentially useful monitor organs to reflect the health of aquatic organisms. Actually, gills have frequently been used in the assessment of impact of aquatic pollutants in freshwater as well as marine habitats (Athikesavan et al. 2006; Fernandes et al. 2007; Abrahamson et al. 2007; Jönsson et al. 2009). So in this study, zebrafish (D. rerio) were exposed to subchronic MCLR concentrations (2 or 20 μg/l MCLR, both of which were waterborne) for 30 days, and the oxidative stress response in gill was investigated via measurement of toxin accumulation, PP activity and antioxidant parameters (SOD, CAT, GPx, GR, GST, and GSH), as well as levels of OH and LPO.
Materials and methods
Zebrafish exposure experiment
Zebrafish were acclimatized in aerated fresh water tanks for 20 days prior to the experiment at a water temperature of 25°C and a 12 h light/dark cycle, and fed twice a day, 0900 and 1500 hours, with commercial dry bait. During the acclimation, fish were supplemented with artemia (about 40 mg per fish) three times a week to maintain nutritional status. Then, fish (weighing 0.7 ± 0.07 g) were randomly assigned to three experimental groups for exposure to two MCLR concentrations, 2 and 20 μg/l, and non-exposure. Each treatment included two groups with 40 fishes for each group. The experiments were carried out in glass tanks (44 × 30 × 28 cm3) with 30 l filtered fresh water and lasted for 30 days under the same conditions as described above during acclimation. Each day, one-third of the aged water was renewed with fresh water containing 2 or 20 μg/l MCLR, or none. No mortality was found in any treatment during the 30 day exposure. At the end of the experiment, all the fishes were taken from each group and their gills were dissected and pooled together. Immediately, they were frozen in liquid nitrogen and stored at −80°C for toxin content and biochemical response analysis.
MCLR analysis
MCLR content in zebrafish gills was analyzed according to the method of Deblois et al. (2008) with minor modification. Briefly, freeze-dried tissue was homogenized in 0.6 ml of 85% methanol (MeOH) using an ultrasonic disrupter (Model 450, Branson Ultrasonics, Danbury, CT, USA) at 4°C for 5 min. The homogenate was extracted at 4°C for 6 h and then centrifuged (8,000×g) at 4°C for 10 min, and the supernatant was recovered. The pellet was extracted again using the same procedure and the supernatant was pooled with that collected earlier and washed with the same volume of hexane for 4 h. The hexane layer was discarded and the MeOH layer was completely dried using Speed Vac. The dried gill extract was resolubilized in 100 μl of 50% MeOH for toxin analysis using HPLC with a Diode-Array Detection detector. The toxin separation was performed on a microsphere C18 reverse-phase column (3 μm) under isocratic conditions with a mobile phase of 10 mM ammonium acetate and acetonitrile (7.4:2.6) for 20 min. The toxin content was quantified using an MCLR standard and expressed as micrograms cellular MCLR per gram tissue.
Biochemical assays
PP activity analysis
Gill homogenate was prepared using tissue protein extraction reagent (T-PER, Pierce Biotechnology Inc., Rockford, IL, USA). 0.5 mg gill tissue was sonicated in 1 ml T-PER reagent and the supernatant was recovered by centrifugation (16,000×g) at 4°C for 20 min. PP activity was analyzed according to the method of Fontal et al. (1999). Briefly, 35 μl of gill homogenate was mixed with 5 μl of NiCl2 (40 mM), 5 μl of 5 mg/ml bovine serum albumin (Sigma) and 35 μl of phosphatase assay buffer (50 mM Tris–HCl, 0.1 mM CaCl2, pH 7.4) and incubated at 37°C for 10 min. Then, 120 μl of 100 μM 6,8-difluoro-4-methylumbelliferyl phosphate (Sigma) was added and it was incubated at 37°C for another 30 min. PP activity was analyzed using a fluorescence microplate reader at 355 nm (excitation) and 460 nm (emission).
Antioxidant response assays
For the biochemical assays, samples were homogenized for 5 min with 20 mM Tris-buffer (pH 7.6, containing 1 mM EDTA, 0.25 M sucrose, 0.15 mM NaCl and 1 mM dithiothreitol) at 4°C, using an ultrasonic disrupter (Model 450, Branson Ultrasonics, Danbury, CT, USA). The homogenate was centrifuged at 15,000×g for 20 min at 4°C, and the supernatant was used for biochemical parameter determination. Protein determination was performed using the method of Bradford (1976) with bovine serum albumin as a standard.
SOD activity was determined based on its ability to inhibit the reduction of cytochrome c by the O2 − generated in the xanthine oxidase/hypoxanthine system (McCord and Fridovich 1969). One unit of SOD was defined as the amount of sample causing 50% inhibition of cytochrome c reduction.
CAT activity was analyzed following Beutler (1975), determining the initial amount of H2O2 (50 mM) decomposition at 240 nm. The results were expressed in CAT units, where one unit is the amount of enzyme that hydrolyzes 1 μmol of H2O2/min/mg of protein, at 25°C and pH 8.0.
GPx activity was measured according to the modified method of Xia and Zhu (1987). One unit of GPx activity was described as 1 nmol of GSH consumption/min/mg protein at 37°C.
GR activity was based on methods described previously by Nagalakshimi and Prasad (2001), using sodium phosphate buffer (200 mM, pH 7.4), oxidized glutathione (1 mM) and NADPH (0.1 mM) and then monitoring the reduction in NADPH absorbance at 340 nm. One unit of GR activity was calculated as nanomoles of NADPH oxidized per minute per milligram protein, at 25°C and pH 7.4.
GST activity was determined using 1-chloro-2,4-dinitrobenzene as the substrate, as previously described by Habig et al. (1974). One unit of GST activity was the amount of enzyme that catalyzed the formation of 1 nmol of product/min at 37°C and pH 6.5.
The content of GSH was determined based on spectrofluorimetric assay, as previously described by Hissin and Hilf (1976) with some modifications. For GSH estimation, the assay tube contained 0.1 ml supernatant, 2.8 ml of 1 M phosphate buffer (pH 8.0) and 0.1 ml of 1 mg/ml ortho-phthalaldehyde. Then the mixture was kept at room temperature for 20 min followed by fluorescence measurement. Calibration was carried out in the same process using 2–10 μg concentrations of GSH (Sigma) as a standard. The GSH content was expressed as micrograms per milligram of protein.
The OH content was measured with a commercial kit (Jiancheng Bioengineering Institute, Nanjing, China), according to the manufacturer’s instructions. One unit was defined as the amount that produces 1 mmol of H2O2/mg protein at 37°C.
The LPO level was assessed using the thiobarbituric reactive species (TBARS) assay, which measures the production of LPO products that react with thiobarbituric acid (Ohkawa et al. 1979). Measurement of TBARS was carried out following the method of Barata et al. (2005). TBARS concentrations were derived from an external standard curve of 1,1,3,3-tetramethoxypropane (also referred to as malonaldehyde; MDA) and the values expressed in nanomoles of MDA equivalents per milligram of protein.
Statistical analyses
All measurements were repeated at least five independent replicates and the data were expressed as mean values ± standard deviation. Statistical analysis was carried out using one-way ANOVA and the Fisher Least Significant Difference test to evaluate whether the means were significantly different among different groups. Significant differences were indicated at p < 0.05. Prior to any analysis, data were log-transformed to meet ANOVA assumptions of normality and variance homoscedasticity.
The data were subjected to a principal component analysis (PCA—significant at a factorial weight >0.7). The PCA is generally used to reduce a set of data with a relatively high number of correlated variables (components) which keep most of the information contained in the original data. Each component consists of a number of elements (loads), which represent the correlation of the variables with the component. Statistical relationships between biochemical variables in zebrafish gill were also compared using non-parametric Spearman’s rank correlation.
Results
Effect of MCLR on toxin accumulation and PP activity in zebrafish gill
The toxin content and PP activity of zebrafish gills at the end of this exposure experiment are shown in Fig. 1. No MCLR was detected in MCLR non-exposed zebrafish gill while this toxin treatment significantly accumulated in the treated groups after 30 day exposure (Fig. 1a). In addition, the MCLR levels in the treated groups remarkably increased with an increase of toxin concentration, i.e., the toxin contents attained to 1.1 and 3.2 μg/g tissue, respectively, in the zebrafish gills exposed to 2 and 20 μg/l MCLR. Similarly, the gill’s PP activity in zebrafish was also increased in response to this toxin treatment, and the PP activities increased 1.4 and 1.3 times in zebrafish gills exposed to 2 and 20 μg/l MCLR, separately (Fig. 1b).
Effect of MCLR on various antioxidant parameters in zebrafish gill
Figure 2 shows the response of GST and GSH in zebrafish gill to MCLR treatment. The results show the presence of MCLR exerted a significantly inhibitory effect, and furthermore, which was linearly correlated with the toxin concentration (p < 0.001). Additionally, the low toxin treatment showed little influence on the gill’s OH content (Fig. 3a, p = 0.076), but the high toxin treatment (20 μg/l) significantly increased the gill’s OH level after 30 days of exposure. MCLR treatment negligibly affected the gill’s LPO level in zebrafish after 30 days of exposure (Fig. 3b, p = 0.179).
Activities of the antioxidant enzymes in zebrafish (Danio rerio) gill after 30 days of MCLR exposure (control, 2 and 20 μg/l). a superoxide dismutase (SOD), b catalase (CAT), c glutathione peroxidase (GPx), d glutathione reductase (GR); Data are means ± SD (n = 5–6). Different letters indicate a statistically significant difference among different metal treatments at p < 0.05
Our results demonstrated that the activities of SOD, CAT, GPx and GR in zebrafish gill were responsive to different MCLR treatments (Fig. 4, p < 0.05). The low toxin treatment (2 μg/l MCLR) had a negligible effect on the activities of SOD, CAT, GPx and GR in zebrafish gills, but these enzyme activities were noticeably influenced by the high toxin treatment (20 μg/l), i.e., the SOD and GR activities were kept in the stimulating condition, and the CAT and GPx activities were depressed under the 20 μg/l toxin treatment.
Multivariate analysis of biochemical responses in zebrafish gill
To explore overall relationships between the different variables, we ran a PCA from matrix data that included MCLR, PP, SOD, CAT, GPx, GR, GST, GSH, OH, and LPO (Table 1). The results showed that 78.25% of overall variance was explained by the first two principal components. The first component (PC1, 57.45% of variance) was built by MCLR, SOD, CAT, GPx, GR, GST, GSH, and OH. The PC2 (20.80% of overall variance) was formed by PP and LPO. We also made a vector plot based on the PCA result, which indicated that there was a relationship between some of the parameter variables (e.g., MCLR and OH, or GST and GSH; Fig. 5). In addition, various correlations between the variables were suggested by Spearman’s correlation test (Table 2). The results indicated that MCLR content was negatively correlated with CAT (p < 0.05) and GST activities (p < 0.01) and GSH content (p < 0.01), but showed a positive relationship with OH level (p < 0.05). Both GPx activity and GSH content showed a positive relationship with CAT activity (p < 0.05), howbeit, which was negatively associated with SOD activity (p < 0.01). GST activity was positively correlated with GPx activity (p < 0.05) and GSH level (p < 0.05), and GST activity showed a strongly negative correlation with OH content (p < 0.05). Similarly, GPx activity showed a strikingly negative relationship with GR activity (p < 0.05).
Principal component analysis of all biochemical parameters in zebrafish (Danio rerio) gill. The variables utilized are Microcystin-LR (MCLR) content, the activities of protein phosphatase (PP), superoxide dismutase (SOD), catalase (CAT), glutathione peroxidase (GPx), glutathione reductase (GR), glutathione-S-transferase (GST), glutathione (GSH) content, as well as levels of hydroxyl radical (OH) and lipid peroxidation (LPO)
Discussion
It should be noted that the majority of MC-related research has focused on acute toxicity of MCs to laboratory organisms (Ding et al. 2001; Li et al. 2007; Malbrouck et al. 2003, 2004; Mezhoud et al. 2008a, b), but few efforts have been devoted to explore the chronic effects of MCs. Actually, chronic exposure to MCs through drinking water is the main way for toxin accumulation in organisms including human beings, bearing in mind that the measured concentration of MCs is often a few micrograms per liter in the water of many large eutrophic lakes in the world (Chan et al. 2007; Song et al. 2007). So, to the extent of our knowledge, this is the first study focused on the oxidative stress response in zebrafish gill against 30 day exposure to subchronic MCLR (2 and 20 μg/l). We also notice that the worldwide occurrence of cyanobacterial blooms makes it necessary to perform environmental risk assessment procedures to monitor the effects of MCs on fish, and oxidative stress biomarkers are believe to be valuable tools in this regard (van der Oost et al. 2003).
Our study showed that MCLR significantly accumulated in the treated gill after 30 days of exposure, i.e., the toxin contents attained to 1.1 and 3.2 μg/g tissue, respectively, in the zebrafish gills exposed to 2 and 20 μg/l MCLR. Thus, MCLR could be taken up via fish gill, which supports a previous study that Xie et al. (2005) propose MCs uptake through routes other than the gastrointestinal tract in carnivorous fish (e.g., via gills). Also, this may show the gill as a route for the entry of MCs into fish body. Similarly, a previous study shows that MCs could be accumulated in fish gills under laboratory conditions and in wild fish (Cazenave et al. 2005). It should be noted that the MCLR contents in the toxin-exposed gills in our study were comparable with MCs concentrations in several fish or shellfish species in the environment (Amé et al. 2010; Cazenave et al. 2005; Chen and Xie 2005), hence enabling our study to show an environmentally related significance.
In this study, the accumulated MCLR in the treated gill induced cellular oxidative stress via the increased production of ROS, which was evidenced by the strongly positive relationship between MCLR content and OH level (Table 2). Similar results are found in several previous studies that MCs are able to induce oxidative stress in animals both in vivo (Jos et al. 2005; Prieto et al. 2006) and in vitro (Li et al. 2003; Botha et al. 2004). For instance, Li et al. (2003) find that an increase in ROS level of the treated group is almost two times higher than the control after 6 h exposure to the toxin (Li et al. 2003). In this study, a striking correlation among some of the variables (i.e., MCLR, OH, GST and GSH) suggested that zebrafish might be enforced to initiate its detoxificatory system in fighting against MCLR accumulation and its concomitant ROS attack. Also, a linear inhibition of GST activity and GSH content in the treated zebrafish gill revealed that conjugation of GSH by GST might be the first step in the detoxification of a cyanobacterial toxin, which was highlighted by a strongly negative relationship of MCLR content with GST activity and GSH level. This was consistent with a previous study that shows the existence of a MCLR-GSH conjugate formed enzymatically via soluble GST in various aquatic organisms, with this conjugation appearing to be the first step in MCs detoxification (Pflugmacher et al. 1998). Nevertheless, our study clearly showed that MCLR exposure significantly depressed GST activity in the treated gill, and it is in line with a previous study when inhibition of GST activity has been reported in microalgae, macrophytes and fish eggs exposed to cyanobacterial extracts (Pietsch et al. 2001). Similar results are also observed in diverse organs (e.g., liver, gill, intestine and brain) of Corydoras paleatus experimentally exposed to MCs (Cazenave et al. 2006). However, these last results are not consistent with other studies in which GST activity is either elevated (Wiegand et al. 1999) or unaffected by pure MCs (Li et al. 2003; Malbrouck et al. 2003). Therefore, GST biomarker response might be dependent on species and type of toxicants. In addition, a remarkably negative relationship between GST activity and OH content suggested that inhibition of GST activity might partly be due to being involved in the elimination of ROS.
Regardless of GST and GSH promptly being involved in counteracting MCLR accumulation and its concomitant ROS attack, the animals were presumed to live through oxidant stress during the 30 days of subchronic exposure to 20 μg/l MCLR, which was definitely exemplified by altered activity of some antioxidant enzymes. Our study showed a significant increase of SOD activity after MCLR exposure, possibly indicating its scavenging activity against ROS, which was in line with several previous studies (Takenaka and Otsu 1999; Li et al. 2003; Prieto et al. 2006). For example, Prieto et al. (2006) find that MCLR significantly induces SOD activity in diverse organs (i.e., liver, kidney and gill) of tilapia fish (Oreochromis sp.). However, little change in SOD activity is observed in tilapia gill, however, with a striking decrease of this enzyme in liver and kidney, when they are orally exposed to a single dose of cyanobacterial cells containing 120 μg/fish MCLR for 24 and 72 h (Prieto et al. 2007).
In this study, CAT and GPx activities in the treated gill decreased significantly compared to the control. CAT and GPx act cooperatively as scavengers of hydrogen peroxide (both enzymes) and other hydroperoxide (GPx). The reduction of CAT activity may be attributed to a change in the assembly of enzyme subunits when concentration of MCs increased, as other authors have reported for other antioxidant enzymes (Yin et al. 2005). As for GPx activity, the decrease seemed to be related with a depletion in GSH level, due to GPx exerting its function via catalyzing the conjugation of GSH with hydroperoxide. In this case, both enzymes seem vulnerable in fighting against ROS attack due to MCLR accumulation. A previous study shows that CAT activity decreases in liver (2.1-fold) and kidney (1.5-fold) of tilapia fish exposed to cyanobacterial cells, but no significant changes are observed in gills in any of the experiments performed (Prieto et al. 2007). They also report that a reduction of GPx activity is observed in kidney (1.3-fold), but no noticeable changes are distinguished in liver and gill of fish (Prieto et al. 2007). Prieto et al. (2006) report that MCLR exposure significantly elevates CAT activity in diverse organs (i.e., liver, kidney and gill) of tilapia fish, with exerting little effect on GPx activity in liver and gill and an inducible influence in kidney. However, other authors find a striking increase of CAT and GPx activities in the hepatocytes of the common carp (Cyprinus carpio L.) experimentally exposed to MCLR (Li et al. 2003). The above discrepancies can be due to the different experimental systems, exposure times, routes and doses employed in these studies.
GR activity was noticeably increased in the zebrafish gill after 30 days exposure to 20 μg/l MCLR. GR plays an important role in maintaining a basic GSH pool, via catalyzing the reduction of glutathione disulfide (GSSG) to GSH in NADPH-dependent reaction. So, in the present study, an elevated GR activity might partly be related to a depletion in GSH pool and a concomitantly enhanced production of GSSG caused by MCLR attack, which could be considered as a compensatory response of zebrafish in counteracting oxidative stress. Alternatively, an increase of GR activity in the treated zebrafish gill might be correlated with a decrease of GPx activity, and this could be suggested by a strongly negative relationship between GR and GPx. The strongly negative correlation between GR and GPx might be attributable to the compensatory feedback that an increased GR activity in the treated group was aimed to synthesize more GSH and subsequently initiate GPx activity to fight against oxidative stress. Similarly, increases in GR activity have been observed in fish exposed intraperitoneally to MCLR (Jos et al. 2005; Prieto et al. 2006).
Our study showed that LPO level in zebrafish gill appeared to be independent of the increasing MCLR concentrations, and this was quite unexpected. Taking into account alteration activity of the antioxidant system (e.g., SOD, CAT, GPx, GR, GST and GSH) and an enhanced production of OH under MCLR treatment, it is quite probable that the zebrafish suffered from the MCLR-induced oxidative stress or even oxidative damage. Several studies show that the factors involved in LPO do not seriously depend on the operations and functions in the antioxidant system, i.e., the LPO level is not always correlated with ROS content and it might also be controlled by other factors (Hussain et al. 1987; Strubelt et al. 1996; Nigam et al. 1999). This was in line with our results which showed that the LPO level in zebrafish gill showed no relationship with all the antioxidant biomarkers used in this experiment (Table 2). Additionally, no effect of MCLR on LPO level might be due to the effective and cooperative work in the antioxidant system, and this could be suggested by the striking correlation among the antioxidant system (i.e., SOD, CAT, GPx, GR, GST and GSH). However, in a previous study, induction of LPO in gill is significant in tilapia exposed subchronically to MCs under laboratory conditions (Jos et al. 2005). Similar results have been reported in diverse organs (i.e., liver, kidney, and gill) of tilapia fish under acutely intraperitoneal administration of MCs (Prieto et al. 2006). Clearly, the exact cause concerning the response of LPO to MCLR treatment in this case remains to be established in future.
An interesting finding of this study was that the PP activity was significantly increased in zebrafish gill after 30 days of exposure to MCLR, which was inconsistent with previous studies (Guzman et al. 2003; Malbrouck et al. 2003, 2004; Mezhoud et al. 2008a, b). Malbrouck et al. (2004) reveal that toxin accumulation induced a severe decrease in the PP activity in both fed and fasted juvenile goldfish, and the PP activity is almost totally inhibited in both treatments during the first hour of exposure. Guzman et al. (2003) show that a lethal dose of MCLR profoundly inhibits PP activity in the nuclear compartment, but this inhibition is not detected in sublethal doses which result in detectable changes in the phosphorylation of p53. These imply that the toxic effect of MCLR on PP activity might be dose-dependent, and that the toxic effects of MCLR at a subchronic dose might be quite different from those at an acute dose. Moreover, previous studies show that other targets for MCLR (e.g., the beta unit of ATP-synthase and aldehyde dehydrogenase 2) do exist in organisms (Mikhailov et al. 2003; Chen et al. 2006), and this might interrupt the overall toxicity of MCLR via the inhibition of PP activity. Actually, a previous study has exemplified the mismatch between PP inhibition and toxicity of different congeners of MC in crustacean, which indicates that other mechanisms, such as uptake, transport, detoxification, and the presence of other target sites may modulate the overall toxicity for an animal and can offset or even reverse the specific PP inhibitory activity (Blom and Jüttner 2005). Overall, the subchronic effect of MCLR in this study was not consistent with the acute dose effects noted in previous studies, and its subchronic toxicity might be initiated in cells via the ROS pathway (which was definitely evidenced by the oxidative stress response in zebrafish gill under MCLR treatment), instead of the PP pathway (Gehringer 2004).
In this study, the PCA divided the biochemical variables into two principal components. PC1 was built by MCLR, OH, SOD, CAT, GPx, GR, GST, and GSH, and most of them were involved in the antioxidant and detoxification response, which allowed us to correlate this component with the antioxidant and detoxification system in fish. PC2 highly correlated with LPO and PP, and therefore could be designated as an “intoxicated” condition induced by MCLR attack. Thus, the zebrafish firstly initiated the antioxidant and detoxification response to fight against MCLR accumulation and its concomitant ROS attack, but when the antioxidant and detoxification system were broken down, this toxin might cause toxicity to the fish via inducing LPO and dysregulating dephosphorylation/phosphorylation balance in the cells. Moreover, the strong correlation within the antioxidant and detoxification variables (e.g., GST, GSH, SOD, CAT, GPx, and GR; Table 2) highlighted a highly efficient cooperation in these systems during the fight against MCLR toxicity.
In conclusion, the 30 day exposure to subchronic MCLR (2 and 20 μg/l) caused a significant accumulation of this toxin in the zebrafish gill, and the accumulation linearly increased with an increasing MCLR concentration. The 2 μg/l MCLR treatment negligibly affected OH content and the antioxidant enzymes (SOD, CAT, GPx, and GR), however oxidative stress was induced under the 20 μg/l MCLR treatment in which an enhanced OH content and alteration of the antioxidant enzymes were observed in the treated gills, although both treatments exerted little effect on LPO level. Additionally, the increasing MCLR exposure unexpectedly induced a significant enhancement in the gill PP activity after 30 days of exposure to 2 and 20 μg/l MCLR. Overall, the subchronic toxicity of MCLR might initiate the ROS pathway, instead of the PP pathway which is the main mechanism for the acute toxicity of MCLR. Caution is necessary in utilizing PP inhibition assay to measure MC content in fish tissues and evaluate MC toxicity. PCA seems to be a useful tool when some markers vary in ways that may initially be difficult to interpret, and the PCA results indicated that the most sensitive biomarkers of MCLR exposure were GST and GSH in this fish. Bearing in mind that zebrafish (D. rerio) is a common model species in fish toxicology and the zebrafish gill is potentially useful in screening waterborne pollutants (Jönsson et al. 2009), this fish could be regarded as a suitable bioindicator of MCLR exposure by measuring CAT, GR, GST, and GSH as biomarkers.
References
Abrahamson, A., Andersson, C., Jösson, M. E., Fogelberg, O., Örberg, J., Brunström, B., & Brandt, I. (2007). Gill EROD in monitoring of CYP1A inducers in fish: a study in rainbow trout (Oncorhynchus mykiss) caged in Stockholm and Uppsala waters. Aquatic Toxicology, 85, 1–8.
Amé, M. V., Galanti, L. N., Menone, M. L., Gerpe, M. S., Moreno, V. J., & Wunderlin, D. A. (2010). Microcystin–LR, –RR, –YR and –LA in water samples and fishes from a shallow lake in Argentina. Harmful Algae, 9, 66–73.
Andersen, R. J., Luu, H. A., Chen, D. Z. X., Holmes, C. F. B., Kent, M. L., Le Blanc, M., Taylor, F. J. R., & Williams, D. E. (1993). Chemical and biological evidence links microcystins to salmon netpen liver disease. Toxicon, 31, 1315–1323.
Athikesavan, S., Vincent, S., Ambrose, T., & Velmurugan, B. (2006). Nickel induced histopathological changes in the different tissues of freshwater fish, Hypophthalmichthys molitrix (Valenciennes). Journal of Environmental Biology, 27, 391–395.
Bainy, A. C. D., Saito, E., Carvalho, P. S. M., & Junqueira, V. (1996). Oxidative stress in gill, erythrocytes, liver and kidney of Nile tilapia (Oreochromis niloticus) from a polluted site. Aquatic Toxicology, 34, 151–162.
Barata, C., Lekumberri, I., Vila-Escalé, M., Prat, N., & Porte, C. (2005). Trace metal concentration, antioxidant enzyme activities and susceptibility to oxidative stress in the tricoptera larvae Hydropsyche exocellata from Llobregat river basin (NE Spain). Aquatic Toxicology, 74, 3–19.
Beutler, E. (1975). The preparation of red cells for assay. In E. Beutler (Ed.), Red cell Metabolism: A Manual of Biochemical Methods (pp. 8–18). New YorK: Grune & Straton.
Blom, J. F., & Jüttner, F. (2005). High crustacean toxicity of microcystin congeners does not correlate with high protein phosphatase inhibitory activity. Toxicon, 46, 465–470.
Botha, N., Gehringer, M. M., Downing, T. G., van de Venter, M., & Shephard, E. G. (2004). The role of microcystin-LR in the induction of apoptosis and oxidative stress in Caco-2 cells. Toxicon, 43, 85–92.
Bradford, M. (1976). A rapid and sensitive assay of protein utilizing the principle of dye binding. Analyt Biochem, 772, 248–264.
Carmichael, W. W., & Falconer, I. R. (1993). Diseases related to freshwater blue green algal toxins, and control measures. In I. R. Falconer (Ed.), Algal Toxins in Seafood and Drinking Water (pp. 187–209). London: Academic.
Cazenave, J., Wunderlin, D. A., de los Ángeles Bistoni, M., Amé, M. V., Krause, E., Pflugmacher, S., & Wiegand, C. (2005). Uptake, tissue distribution and accumulation of microcystin-RR in Corydoras paleatus, Jenynsia multidentata and Odontesthes bonariensis. A field and laboratory study. Aquatic Toxicology, 75, 178–190.
Cazenave, J., Bistoni, M. A., Pesce, S. F., & Wunderlin, D. A. (2006). Differential detoxification and antioxidant response in diverse organs of Corydoras paleatus experimentally exposed to microcystin-RR. Aquatic Toxicology, 76, 1–12.
Chan, W. S., Recknagel, F., Cao, H. Q., & Park, H. D. (2007). Elucidation and short-term forecasting of microcystin concentrations in Lake Suwa (Japan) by means of artificial neural networks and evolutionary algorithms. Water Research, 41, 2247–2255.
Chen, J., & Xie, P. (2005). Seasonal dynamics of the hepatotoxic microcystins in various organs of four freshwater bivalves from the large eutrophic Lake Taihu of subtropical China and the risk to human consumption. Environmental Toxicology, 20, 572–584.
Chen, T., Cui, J., Liang, Y., Xin, X. B., Young, D. O., Chen, C., & Shen, P. P. (2006). Identification of human liver mitochondrial aldehyde dehydrogenase as a potential target for microcystin-LR. Toxicology, 220, 71–80.
Chorus, I., & Bartram, J. (1999). Toxic Cyanobacteria in Water: A Guide to Public Health Consequences, Monitoring, and Management. London and New York: E&FN Spon on behalf of WHO.
Deblois, C. P., Aranda-Rodriguez, R., Giani, A., & Bird, D. F. (2008). Microcystin accumulation in liver and muscle of tilapia in two large Brazilian hydroelectric reservoirs. Toxicon, 51, 435–448.
Ding, W. X., Shen, H. M., & Ong, C. N. (2001). Critical role of reactive oxygen species formation in microcystin-induced cytoskeleton disruption in primary cultured hepatocytes. Journal of Toxicology and Environmental Health, 64, 507–519.
Evans, D. H., Piermarini, P. M., & Choe, K. P. (2005). The multifunctional fish gill: dominant site of gas exchange, osmoregulation, acid–base regulation, and excretion of nitrogenous waste. Physiological Reviews, 85, 97–177.
Fernandes, C., Fontainhas-Fernandes, A., Monteiro, S. M., & Salgado, M. A. (2007). Histopathological gill changes in wild leaping grey mullet (Liza saliens) from the Esmoriz-Paramos coastal lagoon, Portugal. Environmental Toxicology, 22, 443–448.
Fontal, O. I., Vieytes, M. R., Baptista de Sousa, J. M., Louzao, M. C., & Botana, L. M. (1999). A fluorescent microplate assay for microcystin-LR. Analytical Biochemistry, 269, 289–296.
Gehringer, M. M. (2004). Microcystin-LR and okadaic acid-induced cellular effects: a dualistic response. FEBS Letters, 557, 1–8.
Guzman, R. E., Solter, P. F., & Runnegar, M. T. (2003). Inhibition of nuclear protein phosphatase activity in mouse hepatocytes by the cyanobacterial toxin microcystin-LR. Toxicon, 41, 773–781.
Habig, W. H., Pabst, M. J., & Jakoby, W. B. (1974). Glutathione S-transferases. The first enzymatic step in mercapturic acid formation. Journal of Biological Chemistry, 249, 7130–7139.
Hissin, P. J., & Hilf, R. (1976). A fluoremetric method for determination of oxidized and reduced glutathione in tissues. Analytical Biochemistry, 74, 214–226.
Hoeger, S. J., Hitzfeld, B. C., & Dietrich, D. R. (2005). Occurrence and elimination of cyanobacterial toxins in drinking water treatment plants. Toxicology and Applied Pharmacology, 203, 231–242.
Hussain, T., Shukla, G., & Chandra, S. V. (1987). Effects of cadmium on superoxide dismutase and lipid peroxidation in liver and kidney of growing rats: in vivo and in vitro studies. Pharm Toxicol, 60, 355–359.
Jönsson, M. E., Brunström, B., & Brandt, I. (2009). The zebrafish gill model: Induction of CYP1A, EROD and PAH adduct formation. Aquatic Toxicology, 91, 62–70.
Jos, Á., Pichardo, S., Prieto, A. I., Repetto, G., Vázquez, C. M., Moreno, I., & Cameán, A. M. (2005). Toxic cyanobacterial cells containing microcystins induce oxidative stress in exposed tilapia fish (Oreochromis sp.) under laboratory conditions. Aquatic Toxicology, 72, 261–271.
Kondo, F., Matsumoto, H., Yamada, S., Ishikawa, N., Ito, E., Nagata, S., Ueno, Y., Suzuki, M., & Harada, K. (1996). Detection and identification of metabolites of microcystins formed in vivo in mouse and rat livers. Chemical Research in Toxicology, 9, 1355–1359.
Li, X. Y., Liu, Y. D., Song, L., & Liu, J. T. (2003). Responses of antioxidant systems in the hepatocytes of common carp (Cyprinus carpio L.) to the toxicity of microcystin-LR. Toxicon, 42, 85–89.
Li, X. Y., Chung, I. K., Kim, J. I., & Lee, J. A. (2004). Subchronic oral toxicity of microcystin in common carp (Cyprinus carpio L.) exposed to microcystis under laboratory conditions. Toxicon, 44, 821–827.
Li, L., Xie, P., Li, S. X., Qiu, T., & Guo, L. G. (2007). Sequential ultrastructural and biochemical changes induced in vivo by the hepatotoxic microcystins in liver of the phytoplanktivorous silver carp Hypophthalmichthys molitrix. Comp. Biochem Physiol C: Pharmacol Toxicol, 146, 357–367.
Malbrouck, C., Trausch, G., Devos, P., & Kestemont, P. (2003). Hepatic accumulation and effects of microcystin-LR on juvenile goldfish Carassius auratus L. Comp Biochem Physiol C: Toxicol Pharmacol, 135, 39–48.
Malbrouck, C., Trausch, G., Devos, P., & Kestemont, P. (2004). Effect of microcystin-LR on protein phosphatase activity and glycogen content in isolated hepatocytes of fed and fasted juvenile goldfish Carassius auratus L. Toxicon, 44, 927–932.
Mallatt, J. (1985). Fish gill structural changes induced by toxicants and other irritants: a statistical review. Canadian Journal of Fisheries and Aquatic Sciences, 42, 630–648.
McCord, J. M., & Fridovich, I. (1969). Superoxide dismutase: an enzymatic function for erythrocuprein (hemocuprein). Journal of Biological Chemistry, 244, 6049–6055.
Mezhoud, K., Bauchet, A. L., Château-Joubert, S., Praseuth, D., Marie, A., François, J. C., Fontaine, J. J., Jaeg, J. P., Cravedi, J. P., Puiseux-Dao, S., & Edery, M. (2008). Proteomic and phosphoproteomic analysis of cellular responses in medaka fish (Oryzias latipes) following oral gavage with microcystin-LR. Toxicon, 51, 1431–1439.
Mezhoud, K., Praseuth, D., Puiseux-Dao, S., François, J. C., Bernard, C., & Edery, M. (2008). Global quantitative analysis of protein expression and phosphorylation status in the liver of the medaka fish (Oryzias latipes) exposed to microcystin-LR I. Balneation study. Aquatic Toxicology, 86, 166–175.
Mikhailov, A., Härmälä-Braskén, A. S., Hellman, J., Meriluoto, J., & Eriksson, J. E. (2003). Identification of ATP-synthase as a novel intracellular target for microcystin-LR. Chem Bio Interact, 142, 223–237.
Mohamed, Z. A., Carmichael, W. W., & Hussein, A. A. (2003). Estimation of microcystins in the freshwater fish Oreochromis niloticus in an Egyptian fish farm containing a Microcystis bloom. Environmental Toxicology, 13, 134–141.
Nagalakshimi, N., & Prasad, M. N. V. (2001). Responses of glutathione cycle enzymes and metabolism to cooper stress in Scenedesmus bijugatus. Plant Science, 160, 291–299.
Nigam, D., Shukla, G. S., & Agarwal, A. K. (1999). Glutathione depletion and oxidative damage in mitochondria following exposure to cadmium in rat liver and kidney. Toxicology Letters, 106, 151–157.
Ohkawa, H., Ohishi, N., & Yagi, K. (1979). Assay for lipid peroxides in animal tissues by thiobarbituric acid reaction. Analytical Biochemistry, 95, 351–358.
Paerl, H. W., Fulton, R. S., Moisander, P. H., & Dyble, J. (2001). Harmful freshwater algal blooms, with an emphasis on cyanobacteria. Sci World J, 1, 76–133.
Pflugmacher, S. (2004). Promotion of oxidative stress in the aquatic macrophyte Ceratophyllum demersum during biotransformation of the cyanobacterial toxin microcystin-LR. Aquatic Toxicology, 70, 169–178.
Pflugmacher, S., Wiegand, C., Oberemm, A., Beattie, K. A., Krause, E., Codd, G. A., & Steinberg, C. (1998). Identification of an enzymatically-formed glutathione conjugate of the cyanobacterial hepatotoxin microcystin-LR. The first step of detoxification. Biochem Biophys Acta, 1425, 527–533.
Pflugmacher, S., Wiegand, C., Beattie, K., Krause, E., Steinberg, C., & Codd, G. (2001). Uptake, effects, and metabolism of cyanobacterial toxins in the emergent reed plant Phragmites australis (Cav.) Trin Ex steud. Environmental Toxicology and Chemistry, 20, 846–852.
Pietsch, C., Wiegand, C., Amé, M. V., Nicklisch, A., Wunderlin, D., & Pflugmacher, S. (2001). The effects of a cyanobacterial crude extract on different aquatic organisms: evidence for cyanobacterial toxin modulating factors. Environmental Toxicology, 16, 535–542.
Pinho, G. L., Moura da Rosa, C., Maciel, F. E., Bianchini, A., Yunes, J. S., Proença, L. A., & Monserrat, J. M. (2005). Antioxidant responses and oxidative stress after microcystin exposure in the hepatopancreas of an estuarine crab species. Ecotox Environ Saf, 61, 353–360.
Prieto, A. I., Jos, Á., Pichardo, S., Moreno, I., & Cameán, A. M. (2006). Differential oxidative stress response to microcystin LR and RR in intraperitoneally exposed tilapia fish (Oreochromis sp.). Aquatic Toxicology, 77, 314–321.
Prieto, A. I., Pichardo, S., Jos, Á., Moreno, I., & Cameán, A. M. (2007). Time-dependent oxidative stress response after acute exposure to toxic cyanobacterial cells containing microcystins in tilapia fish (Oreochromis niloticus) under laboratory conditions. Aquatic Toxicology, 84, 337–345.
Rodger, H. D., Turnbull, T., Edwards, C., & Codd, G. A. (1994). Cyanobacterial (blue-green algal) bloom associated pathology in brown trout (Salmo trutta L.) in Loch Leven, Scotland. Journal of Fish Diseases, 17, 177–181.
Song, L. R., Chen, W., Peng, L., Wan, N., Gan, N. Q., & Zhang, X. M. (2007). Distribution and bioaccumulation of microcystins in water columns: A systematic investigation into the environmental fate and the risks associated with microcystins in Meiliang Bay, Lake Taihu. Water Research, 41, 2853–2864.
Strubelt, O., Kremer, J., Tilse, A., Keogh, J., Peutz, R., & Younes, M. (1996). Comparative studies on the toxicity of mercury, cadmium and copper toward the isolated perfused rat liver. Journal of Toxicology and Environmental Health, 47, 267–283.
Takenaka, S., & Otsu, R. (1999). Effects of L-cysteine and reduced glutathione on the toxicities of microcystin-LR: the effect for acute liver failure and inhibition of protein phosphatase activity. Aquatic Toxicology, 48, 65–68.
van der Oost, R., Beyer, J., & Vermeulen, N. P. E. (2003). Fish bioaccumulation and biomarkers in environmental risk assessment: a review. Environmental Toxicology and Pharmacology, 13, 57–149.
WHO. (1998). Guidelines for Drinking-Water Quality, Addendum to vol. 2. Geneva: World Health Organisation.
Wiegand, C., Pflugmacher, S., Oberemm, A., Meems, N., Beattie, K. A., Steinberg, C., & Codd, G. A. (1999). Uptake and effects of microcystin-LR on detoxification enzymes of early life stages of the zebrafish (Danio rerio). Environmental Toxicology, 14, 89–95.
Wilhelm Filho, D., Torres, M. A., Marcon, J. L., Fraga, C. G., & Boveris, A. (2000). Comparative antioxidant defense in vertebrates—emphasis on fish and mammals. Trends Comp Biochem Physiol, 7, 33–45.
Williams, D. E., Kent, M. L., Anderson, R. J., Klix, H., & Holmes, C. F. B. (1995). Tissue distribution and clearance of tritium-labeled dihydromicrocystin-LR epimers administered to Atlantic salmon via intraperitoneal injection. Toxicon, 33, 125–131.
Wiston, G. W., & DiGiulio, R. T. (1991). Prooxidant and antioxidant mechanisms in aquatic organism. Aquatic Toxicology, 19, 137–161.
Xia, Y. M., & Zhu, L. Z. (1987). Measurement method of glutathione peroxidase activity in blood and tissue. J Hyg Res, 16, 29–33.
Xie, L., Xie, P., Guo, L., Li, L., Miyabara, Y., & Park, H. D. (2005). Organ distribution and bioaccumulation of microcystins in freshwater fish at different trophic levels from the eutrophic Lake Chaohu, China. Environmental Toxicology, 20, 293–300.
Xie, L., Yokoyamab, A., Nakamuraa, K., & Park, H. D. (2007). Accumulation of microcystins in various organs of the freshwater snail Sinotaia histrica and three fishes in a temperate lake, the eutrophic Lake Suwa, Japan. Toxicon, 49, 646–652.
Yin, L., Huang, J., Huang, W., Li, D., Wang, G. H., & Liu, Y. (2005). Microcystin-RR-induced accumulation of reactive oxygen species and alteration of antioxidant systems in tobacco BY-2 cells. Toxicon, 46, 507–512.
Zimba, P. V., Khoo, L., Gaunt, P., Carmichael, W. W., & Brittain, S. (2001). Confirmation of catfish, Lctalurus punctatus (Rafinesque), mortality from microcystins toxins. Journal of Fish Diseases, 24, 41–47.
Acknowledgments
This work was supported by open research founding for Integrated Marine Monitoring and Applied Technologies in Harmful Algal Blooms, and it was granted from the Key Laboratory of East China Sea Branch, State Oceanic Administration People’s Republic of China (MATHAB200901).
Author information
Authors and Affiliations
Corresponding author
Rights and permissions
About this article
Cite this article
Chen, Y., Zeng, SF. & Cao, YF. Oxidative stress response in zebrafish (Danio rerio) gill experimentally exposed to subchronic microcystin-LR. Environ Monit Assess 184, 6775–6787 (2012). https://doi.org/10.1007/s10661-011-2457-0
Received:
Accepted:
Published:
Issue Date:
DOI: https://doi.org/10.1007/s10661-011-2457-0