Abstract
Application of signaling molecules has gained immense importance in improving the phytoremediative capacity of plants. This study investigated the possible role of melatonin (MEL) as a signaling molecule in ameliorating lead (Pb)-induced oxidative injury in safflower seedlings. Pot grown 10-day-old safflower seedlings were exposed to 50 μM Pb (NO3)2 alone and in combination with different MEL concentrations (0–300 μM). Exposure to Pb, resulted in a severe oxidative stress, which was indicated by reducing biomass production and enhancing the level of oxidative stress markers (e.g. MDA and H2O2). Addition of exogenous MEL considerably decreased Pb uptake and its root-to-shoot translocation while, biomass production of roots, stems and leaves increased significantly. With MEL application a marked increase in reduced glutathione (GSH) content in leaves and roots was noted as compared with Pb treatment alone. In leaves the activity of enzymes involved in glyoxalase system increased markedly by adding MEL to Pb-sressed plants. In response to increasing MEL treatments, the phytochelatin content of leaves increased substantially in comparison with Pb treatment alone. These findings confirmed that MEL can alleviate Pb toxicity by reducing Pb uptake and its root-to-shoot translocation along with modulating different antioxidant systems. The results also showed that despite the insignificant effect of melatonin on the improvement of Pb phytoremediation potential, the application of this signaling molecule can improve the survival of safflower in Pb-contaminated soils by stimulating antioxidant defense mechanisms.
Similar content being viewed by others
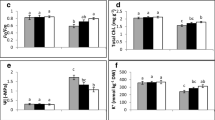
Explore related subjects
Discover the latest articles, news and stories from top researchers in related subjects.Avoid common mistakes on your manuscript.
Introduction
In recent decades, increasing heavy metals (HMs) pollution has become a serious problem. Amongst heavy metals, Pb is one of the most hazardous toxic elements to all shapes of life (Li et al. 2015). Anthropogenic release from modern industrial and agricultural activities has boosted Pb contents in the environment, leading to the elevation of human health problems. Also Pb accumulation can cause serious perturbations in plants (Zhou et al. 2017). Plants grown in soils contaminated with Pb may reveal severe toxicity symptoms caused by reactive oxygen species (ROS) generation (Rossato et al. 2012). To lessen the damage caused by ROS, plants have evolved a wide spectrum of antioxidant systems including enzymatic (e.g., superoxide dismutase (SOD), catalase (CAT), ascorbate peroxidase (APX) and glutathione reductase (GR)) and non-enzymatic metabolites (e.g., GSH and non-protein thiols (NP-SH)) (Gill and Tuteja 2010).
Besides ROS, methylglyoxal (MG) is another cytotoxic compound that accumulates in plants grown under HMs stress (Talukdar 2016). In plants, glyoxalase system, including glyoxalase I (Gly I) and glyoxalase II (Gly II), is responsible for MG detoxification. Gly I can convert GSH to S-D-lactoylglutathione (SLG) and Gly II subsequently converts SLG to D-lactate and GSH that the latter can reprocess (Hasanuzzaman and Fujita 2013; Talukdar 2016).
The application of efficient methods like phytoremediation to clean up HMs in contaminated soils can be a really advantageous approach. Phytoremediation has been demonstrated to be a cost-effective option for HM soil remediation. Plants can store HMs in tissues and sinks that can be harvested for environmentally safe disposal. Therefore, enhancing HM concentrations in the harvestable plant parts such as the leaves and stems is desirable. It has been reported that the induction of some antioxidant systems by applying some natural antioxidants in plant exposed to HMs can increase phytoremediative ability (Hasanuzzaman and Fujita 2013; Xu et al. 2015; Namdjoyan et al. 2017; Sharma et al. 2019a, 2019b). Melatonin, a potent antioxidant, has been demonstrated as playing a crucial role in plant stress tolerance (Cui et al. 2017; Sharma and Zheng 2019). Because of its antioxidant capacity, MEL can quench ROS directly. It is reported that exogenous application of MEL can ameliorate the detrimental effects of various biotic and abiotic stresses by stimulating antioxidant enzymes activity and synthesis of some none-enzymatic antioxjdants (Hasan et al. 2015). MEL also can play a significant role in the alleviation of ROS-caused oxidative damage through the stimulation of mitochondrial electron transport chain that will be followed by a reduction in free radical generation (Posmyk et al. 2008; Sarafi et al. 2017). Furthermore, it has been reported that MEL is capable of ameliorating toxic effects of HMs stress through the activation of some antioxidant systems (Hasan et al. 2015; Sarafi et al. 2017).
Because of high biomass production of crop plants and their considerable capacity to adapt to variable environments, the application of these plants for phytoremediation of contaminated soils has been widely used. Safflower (Carthamus tinctorius L.), is an annual crop plant with a vast geographical propagation. This oil seed crop is well known from ancient times for its medicinal use. Safflower flowers are used for coloring and flavoring the food and the seed oil of this plant has a very high quality. In addition to the nutritional and economic importance, safflower has been reported to possess notable properties in terms of HM tolerance and accumulation (Namdjoyan et al. 2011; Angelova et al. 2016). Previously, we found that some signaling molecules like salicylic acid and nitric oxide are able to improve safflower plant tolerance to Zn-induced toxicity (Namdjoyan et al. 2017).
Earlier studies with MEL have shown that the exogenous application of MEL could reduce the destructive effects of HMs-induced oxidative stress in some plant species (Hasan et al. 2015; Sarafi et al. 2017). The accumulation capability of safflower for some heavy metals and notable properties of this plant to tolerate the stress caused by HMs toxicity supports evaluation of exogenous melatonin treatments for metal tolerance and detoxification mechanism for its utility in phytoremediation. Though, there are several reports regarding pivotal role of antioxidant molecules in alleviation of oxidative injuries in some HM-stressed plant species (Mostofa and Fujita 2013; Namdjoyan et al. 2017), to our knowledge, the possible role of MEL in reduction of HMs-induced phytotoxicity has seldom been assessed.
In this context, this study was designed to investigate the possible effect of MEL on the improvement of Pb toxicity tolerance in Pb-stressed safflower plants. Moreover, in order to better understand Pb detoxification strategy and also to estimate phytoremediative capacity of MEL, the changes in phytochelatins (PCs) biosynthesis and glyoxalase system activity were explored in MEL-supplemented Pb-stressed plants. To our knowledge, this is the first study on the MEL participation on PC biosynthesis and MG detoxification in Pb-stressed safflower plants.
Material and methods
Plant material and growth conditions
After surface-sterilization with HgCl2 solution (0.1%, w/v), the safflower seeds were germinated on a wet filter paper at 25 °C for 48 h under sterile conditions. Next, uniform size germinated seedlings were transplanted in plastic pots (20 cm × 30 cm), 8 plants per pot, filled with perlite. Full-strength hoagland nutrient solution (Arnon and Hogland 1940) was applied. After 10 days of stabilization, Pb and MEL treatments were applied either alone or in combination. To determine the optimal dose, preliminary experiments with different concentrations of Pb(NO3)2 and MEL were carried out independently (data not presented). Pb treatments (0 and 50 μM Pb(NO3)2) were applied by root irrigation and foliar portions of seedlings were simultaneously sprayed with five levels of MEL (0, 100, 150, 200 and 300 μM) in pots containing 8 plants as follows: (T1) Control (0 μM Pb(NO3)2 + 0 μM MEL), (T2) (50 μM Pb(NO3)2 + 0 μM MEL), (T3) (0 μM Pb(NO3)2 + 100 μM MEL), (T4) (50 μM Pb(NO3)2 + 100 μM MEL), (T5) (0 μM Pb(NO3)2 + 150 μM MEL), (T6) (50 μM Pb(NO3)2 + 150 μM MEL), (T7) (0 μM Pb(NO3)2 + 200 μM MEL), (T8) (50 μM Pb(NO3)2 + 200 μM MEL), (T9) (0 μM Pb(NO3)2 + 300 μM MEL), (T10) (50 μM Pb(NO3)2 + 300 μM MEL) (Table 1). To prepare sprayed MEL solution, MEL was dissolved in 100% ethanol at a concentration of 10 mM and stored at −20 °C as a stored solution. At the time of use, stored solution was further diluted in to different concentrations. Hoagland solutions with or without Pb, were changed every 5 days followed by foliar spray of melatonin and their pH adjusted to 6.5. A completely randomized design with five replicates was carried out. Plants were grown in a controlled-environment growth room with the following conditions: 16/8 light/dark photoperiod and photon flux density 200 µmol m−2 s−1, Day/night temperature of 26/22 °C and 65 ± 5% relative humidity. The plants were harvested after 14 days of growth and subsequently separated the root, stem and leaf samples. The samples were dried at 80 °C for 48 h to estimate plant dry mater and Pb concentration.
Concentration of Pb
Powdered root, stem and leaf samples (2 g) were digested in a 5 ml of ternary mixture of HNO3:H2SO4:HClO4 (10:1:4 (v/v/v)). By using atomic absorption spectrophotometer (Perkin Elmer, Germany) the concentration of Pb in different samples was measured.
Chlorophyll contents
Fresh leaf samples (0.2 g) after homogenization in 80% acetone were filtered. Finally, total chlorophyll contents of homogenates were measured spectrophotometrically at 645 and 663 nm using method described by Lichtenthaler (1987).
Analysis of lipid peroxidation
According to method described by Heath and Packer (1968), the levels of lipid peroxidation were estimated after evaluating malondialdehyde (MDA) content in tissues. To achieve this, fresh leaf samples (0.5 g) were ground in 5 ml of 0.1% trichloroacetic acid (TCA). Next, resultant was centrifuged for 5 min at 10,000 × g. After adding 1 ml of supernatant to 4 ml of 0.5% thiobarbituric acid (TBA) in 20% TCA, the mixture was incubated at 95 °C for 30 min and then cooled quickly on ice. Finally, the absorbance of centrifuged mixture was evaluated at 532 and 600 nm (E = 155 mM−1 cm−1).
Hydrogen peroxide (H2O2) determination
Hydrogen peroxide content measurement was performed spectrophotometrically in accordance to Velikova et al. (2000). The extraction was done using leaf samples (0.2 g) in 3 ml of 0.1% (w/v) TCA in a bath. Supernatant were then centrifuged for 15 min at 12,000 × g. After mixing 0.5 ml of phosphate buffer (pH 7.0) and 1 ml of 1 M KI with 0.5 ml of supernatant, the content of H2O2 was estimated by the measurement of absorbance at 390 nm (E= 0.28 μM−1 cm−1) and stated as μmol g−1 FW (Fresh weight).
GSH and GSSG contents
The recycling method described by Anderson (1985) was used to assess GSH and GSSG content. To achieve this, 500 mg of fresh root and leaf samples were homogenized in 0.3 ml of 5% sulfosalicylic acid. After that, the supernatant was centrifuged at 10,000 × g for 10 min and then, a 0.5 ml aliquot of supernatant was added in a tube containing 0.5 ml reaction buffer [0.1 M phosphate buffer (pH 7.0), 0.5 mM EDTA and 50 μl of 3 mM 5,5′-dithio-bis (2-nitrobenzoic acid) (DTNB)]. The determination of GSH content was done by reading the absorbance at 412 nm. GSSG was calculated by subtracting the GSH from the total glutathione concentration.
Extraction and assay of phytochelatins (PCs)
Phytochelatins content was determined by the method described by Molina et al. (2008). Briefly, Fresh root and leaf samples of a known weight (0.1 g) were homogenized in 3 ml of 3% (w/v) sulfosalicylic acid for 30 min for the extraction of nonprotein thiols. Homogenized material was centrifuged at 10,000 × g for 15 min at 4 °C. Then, a 100 μl of the supernatant immediately mixed with 1 ml of Tris-HCl (0.2 mM, pH 8.2) and 75 μl DTNB (10 mM). Next, the mixture was incubated for 10 min and then its absorbance was estimated at 412 nm. An aliquot without DTNB was prepared to adjust the spectrophotometer to zero absorbance. The difference between non-protein thiols and GSH was considered in theoretical determination of PCs.
Enzyme assays
Homogenization of fresh samples (500 mg) was performed in 5 ml of ice cold potassium phosphate buffer (50 mM, pH 7.5) including 1 mM EDTA, 1% polyvinylpyrrolidone (pvp), using a prechilled mortar and pestle. In accordance to Bradford (1976), the content of protein was estimated via applying bovin serum albumin (BSA) as a standard. The estimation of SOD (EC 1.15.1.1) activity was performed by preparing 1.5 ml of reaction mixture containing 100 mM phosphate buffer (pH 7.5), 13 mM methionine, 2.25 mM nitroblu tetrazolium (NBT), 0.1 mM EDTA, 60 μM riboflavin, and enzyme extract. One unit of SOD activity was described as the volume of enzyme needed to cause 50% reduction in color at 560 nm (Beauchamp and Fridovich 1971).
A mixture containing 25 mM phosphate buffer (pH 7.0, containing 0.1 mM EDTA), 10 mM H2O2, and the enzyme was prepared to estimate CAT (EC 1.11.1.6) activity. The decline in H2O2 absorbance at 240 nm within 1 min (E= 39.4 mM−1 cm−1) was recorded (Aebi 1984).
According to Nakano and Asada (1987), the activity of APX (EC 1.11.1.11) was estimated by preparing an assay mixture consisted of 50 mM phosphate buffer (pH 7.0), 0.1 mM EDTA, 0.1 mM H2O2, 0.5 mM sodium ascorbate, and the enzyme aliquot. Subsequently, rate of ascorbate oxidation was estimated at 290 nm (E = 2.8 mM−1 cm−1).
Following the previously described method of Polle et al. (1994), a reaction mixture containing 100 mM phosphate buffer (pH 7.0), 20 mM guaiacol, 10 mM H2O2 and enzyme was prepared for the evaluation of GPX (EC 1.11.1.7) activity. The enzyme activity was calculated via an increase in absorbance at 470 nm (E= 26.6 mM−1 cm−1) through the oxidation of guaiacol.
Gly I (EC 4.4.1.5) estimation was performed according to method described by Hossain and Fujita (2010). Briefly, an assay mixture, in a final volume of 0.7 ml, was prepared via mixing 100 mM potassium phosphate buffer (pH 7.0), 15 mM magnesium sulfate, 1.7 mM GSH, 3.5 mM MG, and the enzyme aliquot. The reaction was started by adding MG and the activity was calculated at 240 nm for 1 min (E = 3.37 μM−1 cm−1).
For estimating Gly II (EC 3.1.2.6) activity, an assay mixture, in a final volume of 1 ml, including 100 mM Tris-HCl buffer (pH 7.2), 0.2 mM DTNB, 1 mM SLG and enzyme extract was prepared. After adding SLG, Gly II activity was calculated by monitoring the formation of GSH at 412 nm for 1 min (E= 13.6 μM−1 cm−1) (Principato et al. 1987).
All spectrophotometeric analyses were performed at 25 °C using a UV-vis spectrophotometer (Model UV-1601 PC, Shimadzu, Japan).
Statistical analysis
Data presented in figures and table are means ± standard error (SE) of three replicates, with the exception of growth parameters and Pb concentration, where five replications were used. ANOVA accompanied by separation of treatment means applying post hoc Tukey’s test (P< 0.05) was done to determine the significant difference between treatments.
Results
Biomass yield and Pb accumulation
Exposure to Pb caused visible toxicity symptoms, like leaf chlorosis and reduced growth, in safflower seedlings. However, these symptoms improved considerably upon MEL addition. In comparison with the controls (T1), Pb treatment resulted in a remarkable reduction in plant biomass production in different parts of safflower seedlings (Table 1). However, the lowest dry weight belonged to the root tissues, which showed a 78% decrease compared to the control samples (Table 1). As shown in Table 1, dry weight of roots and stems Pb-stressed plants increased slightly up to 100 μM MEL, while at higher concentration of MEL (150–300 μM MEL), root and stem dry weight of Pb-treated plants increased significantly when compared to control samples (T1). In leaves of Pb-stressed safflower seedlings, biomass production increased progressively at all of the MEL concentrations with respect to Pb treatment alone (Table 1).
The Pb concentration increased considerably in roots, stems and leaves of plants exposed to Pb treatment with respect to the controls (Table 1). However, as shown in Table 1, the application of MEL treatments decreased the accumulation of Pb in different parts of Pb-treated safflower with respect to Pb treatment alone.
Total chlorophyll content
The chlorophyll content of Pb-stressed plants depleted sharply compared with the controls (Fig. 1a). Though, the chlorophyll content of MEL-supplemented Pb-treated plants increased significantly in all doses of MEL compared with Pb treatment alone (Fig. 1a). Upon application of 300 μM MEL, a 56% increase in chlorophyll content of Pb-stressed plants was observed in comparison with Pb treatment alone (Fig. 1a).
MDA and H2O2 accumulation
Figure 1b, shows the MDA content of leaves, as a lipid peroxidation biomarker, in Pb-stressed plants. The content of MDA in Pb-treated plants was 2.9-fold higher when compared with the controls. Notably, upon addition of increasing MEL treatments the content of MDA in plants treated with Pb decreased significantly in comparison with Pb treatment alone (Fig. 1b). The lowest MDA content in leaves of MEL-supplemented Pb-stressed plants was noted at 300 μM MEL which was by 35% lower than in plants treated with Pb alone (Fig. 1b).
In comparison with the control, the level of H2O2 induced sharply in plants exposed to Pb treatment alone (Fig. 1c). However, with supplying MEL from 100 to 300 μM, the content of H2O2 decreased significantly in Pb-treated plants, with respect to plants treated with Pb alone (Fig. 1c).
GSH and GSSG content
When safflower plants were exposed to Pb treatment alone, the level of GSH and GSH/GSSH ratio in both roots and leaves increased significantly (Fig. 2a, c). Though, upon addition of MEL treatments, a more marked increase in the level of GSH and GSH/GSSG ratio was recorded in both roots and leaves of Pb-stressed plants in comparison with plants treated with Pb alone (Fig. 2a, c). In roots of Pb-stressed safflower seedling the highest GSH content was noted at 200 μM MEL (Fig. 2a). The maximum GSH level of leaves was recorded at 150 μM of MEL, which was by around 42% higher than in plants treated with Pb alone (Fig. 2a).
The levels of GSSG in root and leaf tissues of Pb-treated plants increased substantially as compared to the controls (Fig. 2b). In both roots and leaves of Pb-stressed safflower plants supplemented with increasing MEL treatments, the level of GSSG exhibited a marked reduction when compared with plants treated with Pb only (Fig. 2b).
Total phytochelatin content
The content of PC increased significantly in root and leaf tissues of Pb-treated plants in comparison with the control (Fig. 2c). In Pb-stressed plants, adding MEL from 100 to 300 μM caused a remarkable increase in PC levels of leaves, while in roots the content of PC increased slightly when compared with plants treated with Pb alone (Fig. 2c). However, in all MEL treatments, Pb- stressed plants tended to contain a higher PC content in leaves than in roots (Fig. 2c).
Antioxidant enzyme activity
Compared to the controls, a significant increase in SOD and APX activity was noted in response to Pb treatment alone (Fig. 3a, b). Conversely, upon addition of increasing MEL treatments the activity of SOD and APX decreased remarkably in comparison with Pb treatment alone (Fig. 3a, b).
As compared to that of controls, the activity of CAT and GPX increased slightly in Pb-stressed safflower seedlings (Fig. 3c, d). However, MEL supplementation exhibited a pattern different to that of SOD and APX (Fig. 3c, d). CAT and GPX activities increased sharply in Pb-stressed plants subjected to increasing MEL treatments, with respect to plants treated with Pb alone (Fig. 3c, d).
A substantive increase in GR activity was recorded in Pb-treated plants, when compared with the controls (Fig. 3e). The GR activity in plants exposed Pb treatment was 2.2-fold higher in comparison with the control (Fig. 3e). Adding MEL treatments to Pb-stressed safflower plants resulted in a further increase in GR activity compared with Pb treatment alone (Fig. 3e).
Activity of glyoxalase system enzymes
The activity of both enzymes involved in glyoxalase system increased substantially in Pb-stressed plants, when compared with the controls (Fig. 4a, b). In response to increasing MEL treatments, Gly I and Gly II activity further increased with respect to plants treated with Pb alone (Fig. 4a, b). At 300 μM MEL, 58 and 77% increases were noted in the activity of Gly I and Gly II, respectively, with respect to safflower plants treated with Pb alone (Fig. 4a, b).
Discussion
In this study, the exposure of safflower seedlings to Pb treatment resulted in a substantive reduction of biomass production along with the induction of toxicity symptoms like leaf chlorosis. These suggest oxidative injury induced by Pb toxicity. Our findings are in accordance with many other reports regarding destructive effects of HMs on plants growth conditions (Hasanuzzaman and Fujita 2013; Hasan et al. 2015; Namdjoyan et al. 2017). Pb-accumulation scheme in the safflower seedlings (roots > stems > leaves) exhibit an presence of a kind of protective barrier restraining Pb from permeating to stems and leaves of safflower seedlings from its roots. Roots are the first part of exposure to metal toxicity; therefore, the biomass production of roots was affected more severely by detrimental effects of Pb toxicity. Though, adding MEL to Pb-stressed plants resulted in a noticeable improvement of growth and biomass production of roots, stems and leaves. Improved growth and biomass production of root and shoot under MEL addition can be attributed to role of MEL in alleviating Pb uptake and reducing further root-to-shoot translocation of this element and its efficient binding to PCs and subsequent sequestration of Pb-PC complex to the leaf vacuoles. Correspondingly, as discussed below, PCs levels revealed significant increases in leaves in response to the MEL application. Elevated production of thioles like GSH and PCs in leaves of MEL-supplemented safflower plants may be attributed to improved biomass production of these tissues.
One of the essential characteristics of plants used in phytoremediation technique is their ability to accumulate heavy metals into harvestable plant parts such as stems and leaves. Considering that MEL application in this study led to decrease of Pb transfer from root to aerial parts of safflower seedlings, it seems that the application of this signaling molecule probably would not have a significant effect on increasing the ability of safflower in the Pb phytoremediation of soils contaminated with Pb.
Since leaves are the major photosynthetic organs of plants and therefore more sensitive to heavy metal toxicity than stems, it seems that the higher levels of Pb in stems than in leaves could be another possible mechanism to minimize the damage caused by heavy metal to the photosynthetic apparatus.
Leaf chlorosis is a prevalent symptom of chlorophyll degradation induced by stressors. Inhibited chlorophyll synthesis in Pb-stressed safflower plants can be related to destructive effects of Pb toxicity on chlorophyll biosynthesis. Furthermore, Pb-induced inhibition of chlorophyll biosynthesis may be associated with Mg and Fe absorption. Pourrut et al. (2011) previously stated that Pb treatment can reduce Mg and Fe uptake in plants. Interestingly, upon MEL application the chlorophyll content of Pb-treated safflower plants increased sharply (Fig. 1a). It has been reported that ROS generated during HM stress can cause a marked decrease in chlorophyll content (Kastori et al. 1998). Thus, we proposed that the increased chlorophyll content in our study could be due to antioxidant nature of MEL and its ability to activate antioxidant systems in safflower cells (Hasan et al. 2015; Cui et al. 2017). Weeda et al. (2014) reported that the MEL is able to downregulate chlorophyllase responsible for chlorophyll degradation. Taken together, it seems that improvement of toxicity symptoms under MEL supplementation is linked to its pivotal role as an efficient antioxidant.
In our study, the content of MDA, a lipid peroxidation biomarker, strikingly increased under Pb stress (Fig. 1b). This is consistent with previous studies that reported induced lipid peroxidation due to HM-induced ROS production (Kazemi et al. 2010; Wang et al. 2013; Hasan et al. 2015). However, the application of MEL conspicuously depleted MDA content in Pb-stressed safflower plants (Fig. 1b), highlighting its active role on the alleviation of detrimental effects of HM-induced ROS. It seems that depleted lipid peroxidation in MEL-supplemented Pb-stressed plants is due to considerable effect of MEL on the activation of some antioxidant systems (Hasan et al. 2015; Cui et al. 2017).
In the present study, the content of H2O2 increaseed remarkably in Pb-stressed plants (Fig. 1c), showing the generation of ROS under Pb excess. It is well authenticated that in parallel with HM stress the level of H2O2 rises in plants (Wang et al. 2013). However, supplying with MEL led to a marked reduction in H2O2 levels of Pb-stressed plants (Fig. 1c). This result is presumably, due to direct effect of MEL as a ROS quencher and/or its effect on the activity of antioxidant enzymes responsible for ROS scavenging (Cui et al. 2017).
In the present study, GSH content increased sharply in roots and leaves of Pb-stressed plants (Fig. 2a), indicating crucial role of GSH on Pb detoxification. The increased level of GSH in Pb-stressed safflower plants can be due to the upregulation of the activity of GR involved in ASA-GSH cycle (Hasanuzzaman and Fujita 2013). Moreover, the function of GSH as a signal molecule in HM-stressed plants has been proved (Seth et al. 2012). Further increase in GSH content of the roots and leaves of MEL-supplemented Pb-stressed plants (Fig. 2a) may be attributed to fact that MEL is able to elevate GSH biosynthesis. Cui et al. (2017) demonstrated that MEL is able to induce GSH biosynthesis under various stress conditions in associated with low H2O2 levels. Also, Wang et al. (2012) reported that exogenous MEL increased GSH content in plants by inducing expression of genes responsible for GSH biosynthesis (Hasan et al. 2015; Wang et al. 2012). In this study, GSH depletion in leaves of MEL-supplemented Pb-stressed plants at higher concentration of MEL may be attributed to its effective consumption during PC biosynthesis.
In our study, exposure of safflower seedlings to Pb treatment caused a marked increase in GSSG content of roots and leaves (Fig. 2b). The increase in GSSG content may be due to the response of GSH to oxyradicals created during heavy metal stress or because of an increase in the activity of some enzymes involved in H2O2 decomposition like glutathione S-transferase (Hasanuzzaman and Fujita 2013). Decrease in GSSG content under HM-induced toxicity has been documented in several studies (Mishra et al. 2006; Namdjoyan et al. 2012; Hasanuzzaman and Fujita 2013). Interestingly, adding MEL to Pb-stressed safflower plants resulted in reduction of GSSG content in both roots and leaves (Fig. 2b). This may be attributed to elevated GSH synthesis by MEL treatment.
In many physiological functions of the cell, the relatively higher levels of GSH/GSSG ratio is fundamental. Therefore, the evaluation of the GSH/GSSG ratio is considered as an important marker to determine the cellular redox status and a useful indicator of oxidative stress (Cui et al. 2017). A higher GSH/GSSG ratio in Pb-stressed safflower seedlings may be correlated to an overcompensation through the reinforced recycling of GSH as an antioxidant and a reductant. The increased GSH/GSSG ratio upon Pb treatment in safflower plants may also indicate the potential of this plant to tolerate Pb stress. In this experiment, adding MEL led to a further increase in GSH/GSSG ratio of Pb-stressed safflower seedlings (Fig. 2c), highlighting the possible role of MEL in ROS balance through the creation of relatively higher levels of GSH/GSSG ratio (Cui et al. 2017).
In our study, the content of PC in both root and leaf tissues increased under Pb treatment alone (Fig. 2d), indicating the active involvement of PCs in Pb detoxification possibly by the chelation of Pb and the subsequent compartmentalization of PC-Pb complex. Adding MEL caused a further increase in PCs level of leaves of Pb-stressed safflower seedlings while a slight PCs increase was recorded in roots (Fig. 2d). This suggested that MEL could effectively induce PC biosynthesis in leaves of Pb-stressed plants, resulting in higher binding of Pb with PC. In like manner, Hasan et al. (2015) reported that MEL treatment can induce PC biosynthesis in Cd-stressed tomato plants via the induction of genes responsible for PC and GSH biosynthesis. Binding of Pb to cell wall or GSH may be responsible for lower PC content of roots of Pb-stressed safflower plants.
Exposure to Pb caused a sharp increment in SOD and APX activity, showing their active role in Pb detoxification. Increased activity of SOD and APX under Pb toxicity may be associated with elevated levels of ROS or upregulated expression of genes accountable for SOD and APX biosynthesis (Mishra et al. 2006). By adding MEL, SOD and APX activity decreased in Pb-stressed seedlings (Fig. 3a, b). Hasan et al. (2015) suggested that MEL as a signal molecule plays an active role in ROS detoxification through activation of various antioxidant defense systems. According to decreased H2O2 levels in MEL-supplemented Pb-stressed safflower plants, it seems that MEL can directly act as a ROS quencher. This can justify depleted levels of SOD and APX activity in our study.
Exposure of safflower seedlings to Pb treatment exhibited a slight increase in CAT and GPX activity (Fig. 3c, d). Though, by applying MEL treatments the activity of both CAT and GPX increased sharply (Fig. 3c, d). We proposed that MEL is able to scavenge Pb-induced ROS directly because of its antioxidative nature and indirectly via activation of enzymes like CAT and GPX. Our results are consistent with those in MEL-supplemented Cd-stressed plants (Hasan et al. 2015). Cui et al. (2017) reported that MEL is capable of inducing genes encoding GPX.
In this study, a sharp increase in GR activity was observed in Pb-stressed plants (Fig. 3e), indicating its crucial role to recycle GSSG to GSH and maintain a high ratio of GSH/GSSG (Mishra et al. 2011; Hasanuzzaman and Fujita 2013). We suggested that Pb treatment increased the activity of GR, thus resulting in higher accumulation of GSH. This strategy can result in reduction of oxidative damage induced by Pb toxicity. However, under all of MEL doses, GR activity further increased (Fig. 3e). Hasan et al. (2015) reported that increased activity of GR, as an effective ROS-scavenging mechanism, is necessary to maintain the GSH/GSSG ratio in MEL-supplemented Cd-stressed tomato plants. Furthermore, according to the important role of GR to recycle the GSSG to GSH and subsequent production of PCs, the increase of GR activity also may be attributed to the more synthesis of PC.
In the current study, Gly I and Gly II activity increased significantly in Pb-treated plants (Fig. 4a, b). Upregulation of these enzymes under HM stress has been demonstrated in some plant species (Hasanuzzaman and Fujita 2013; Mostofa and Fujita 2013; Namdjoyan et al. 2017). An increase in glyoxalase system activity under Pb treatment in our study reveals the active role of this antioxidant system in Pb-induced MG detoxification probably through greater accumulation of GSH. Notably, applying MEL effectively further stimulated Gly I and Gly II activities in Pb-stressed safflower plants (Fig. 4a, b), indicating active role of MEL in Pb detoxification via provoking glyoxalase system activity. Noctor et al. (2012) stated that glyoxalase system is responsible for maintaining redox homeostasis through GSH regeneration. We proposed that MEL may effectively alleviate Pb-induced MG through the increase in GSH biosynthesis. Moreover, according to the positive correlation between MEL and glyoxalase system activity, it seems that MEL is able to reinforce GSH regeneration through the glyoxalase system.
Conclusion
The present study shows that excessive Pb results in oxidative injury to plants as indicated by the elevated levels of oxidative stress markers and reduced biomass production. It seems that MEL application can considerably decrease the deleterious effects of Pb stress through the induction of thiols synthesis accompanied by the activation of some antioxidant enzymes. Apparently, MEL can attenuate destructive effects of Pb toxicity on photosynthetic machinery and biomass production via reduction in Pb uptake and its translocation from roots to above ground parts of safflower seedlings. As the leaves of MEL-supplemented Pb-stressed plants accumulated high levels of thiols like PCs, It seems that decreased root-to-shoot Pb translocation and efficient chelation of this element by PCs and also the subsequent compartmentalization and sequestration of the PC-Pb complex under MEL supplementation might be a useful strategy to alleviate Pb toxicity. Moreover, an increase in glyoxalase system activity may be further potent strategy against Pb toxicity. However, the decrease in Pb accumulation especially in the aerial and harvestable parts of safflower plants, suggests that the MEL has little effect of increasing the phytoremediation potential of Pb. In further experiments, one can envisage conducting field experiments with other plant species to clarify MEL application as a cost-effective approach to progress the survivability of plants in soils contaminated with Pb.
References
Aebi H (1984) Catalase in vitro. Method Enzymol 105:121–126
Anderson ME (1985) Determination of glutathione and glutathione disulfide in biological samples. Methods Enzymol 113:548–554
Angelova VR, Perifanova-Nemska MN, Uzunova GP, Kolentsova EN (2016) Accumulation of heavy metals in safflower(Carthamus tinctorius L.). Int J Biol, Biomolecular, Agric, Food Biotechnological Eng 10(7):410–415
Arnon DI, Hogland DR (1940) Crop production in artificial solutions and in soils with special references to factors influencing yields and absorption of inorganic nutrients. Soil Sci 50:463–484
Beauchamp C, Fridovich I (1971) Superoxide dismutase: improved assays and an assay applicable to acrylamide gels. Anal Biochem 44:276–287
Bradford MM (1976) A rapid sensitive method for the quantification of microgram quantities of protein utilizing the principle of protein-dye binding. Anal Biochem 72:248–254
Cui G, Zhao X, Liu S, Sun F, Zhang C, Xi Y (2017) Beneficial effects of melatonin in overcoming drought stress in wheat seedlings. Plant Physio Biochem 118:138–149
Gill SS, Tuteja N (2010) Reactive oxygen species and antioxidant machinery in abiotic stress tolerance in crop plants. Plant Physiol Biochem 48:909–930
Hasan MK, Ahammed GJ, Yin L, Shi K, Xia X, Zhou Y, Yu J, Zhou J (2015) Melatonin mitigates cadmium phytotoxicity through modulation of phytochelatins biosynthesis, vacuolar sequestration, and antioxidant potential in Solanum lycopersicum L. Front Plant Sci 6:601
Hasanuzzaman M, Fujita M (2013) Exogenous sodium nitroprusside alleviates arsenic-induced oxidative stress in wheat (Triticum aestivum L.) seedlings by enhancing antioxidant defense and glyoxalase system. Ecotoxicology 22:584–596
Heath RL, Packer L (1968) Photoperoxidation in isolated chloroplasts. I. Kinetics and stoichiometry of fatty acid peroxidation. Arch Biochem Biophys 125:189–198
Hossain MA, Fujita M (2010) Evidence for a role of exogenous glycinebetaine and proline in antioxidant defense and methylglyoxal detoxification systems in mung bean seedlings under salt stress. Physiol Mol Biol Plants 16:19–29
Kastori R, Plesničar M, Sakač Z, Panković D, Arsenijević-Maksimović I (1998) Effect of excess lead on sunflower growth and photosynthesis. J Plant Nutr 21(1):75–85
Kazemi N, Khavari-Nejad RA, Fahimi H, Saadatmand S, Nejad-Sattari T (2010) Effect of exogenous salicylic acid and nitric oxide on lipid peroxidation and antioxidant enzyme activities in leaves of Brassica napus L. under nickel stress. Sci Hortic 126:402–407
Li S, Zhang G, Gao W, Zhao X, Deng C, Lu L (2015) Plant growth, development and change in GSH level in safflower (Carthamus tinctorius L.) exposed to copper and lead. Arch Biol Sci 67(2):385–396
Lichtenthaler HK (1987) Chlorophylls and carotenoids: pigments of photosynthetic biomembranes. Method Enzymol 148:350–382
Mishra S, Jha AB, Dubey RS (2011) Arsenite treatment induces oxidative stress, upregulates antioxidant system, and causes phytochelatin synthesis in rice seedlings. Protoplasma 248:565–577
Mishra S, Srivastava S, Tripathi RD, Govindarajan R, Kuriakose SV, Prasad MNV (2006) Phytochelatin synthesis and response of antioxidants during cadmium stress in Bacopa monnieri L. Plant Physiol Biochem 44(1):25–37
Molina AS, Nievas C, Chaca MVP, Garibotto F, Gonzalez U, Marsa SM, Luna C, Gimenez MS, Zirulnik F (2008) Cadmium-induced oxidative damage and antioxidative defense mechanisms in Vigna mungo L. Plant Growth Regul 56:285–295
Mostofa MG, Fujita M (2013) Salicylic acid alleviates copper toxicity in rice (Oryza sativa L.) seedlings by up-regulating antioxidative and glyoxalase systems. Ecotoxicology 22(6):959–973
Nakano Y, Asada K (1987) Purification of ascorbate peroxidase in spinach chloroplasts; its inactivation in ascorbate-depleted medium and reactivation by monodehydroascorbate radical. Plant Cell Physiol 28(1):131–140
Namdjoyan S, Kermanian H, Soorki AA, Tabatabaei SM, Elyasi N (2017) Interactive effects of salicylic acid and nitric oxide in alleviating zinc toxicity of safflower (Carthamus tinctorius L.). Ecotoxicology 26:752–761
Namdjoyan S, Namdjoyan S, Kermanian H (2012) Induction of phytochelatin and responses of antioxidants under cadmium stress in safflower (Carthamus tinctorius L.) seedlings. Turk J Bot 36(5):495–502
Namdjoyan SH, Khavari-Nejad RA, Bernard F, Nejad-Sattari T, Shaker H (2011) Antioxidant defense mechanisms in response to cadmium treatments in two safflower cultivars. Russ J Plant Physiol 58:403–413
Noctor G, Mhamdi A, Chaouch S, Han YI, Neukermans J, Marquez-Garcia B, Queval G, Foyer CH (2012) Glutathione in plants: an integrated overview. Plant Cell Environ 35(2):454–484
Polle A, Otter T, Seifert F (1994) Apoplastic peroxidases and lignification in needles of Norway spruce (Picea abies L.). Plant Physiol 106(1):53–60
Posmyk MM, Kuran H, Marciniak K, Janas KM (2008) Presowing seed treatment with melatonin protects red cabbage seedlings against toxic copper ion concentrations. J Pineal Res 45:24–31
Pourrut B, Shahid M, Dumat C, Winterton P, Pinelli E (2011) Lead uptake, toxicity, and detoxification in plants. Rev Environ Contam Toxicol 213:113–136
Principato GB, Rosi G, Talesa V, Giovannini E, Uotila L (1987) Purification and characterization of two forms of glyoxalase II from the liver and brain of Wistar rats. Biochem Biophys Acta 911:349–355
Rossato LV, Nicoloso FT, Farias JG, Cargnelluti D, Tabaldi LA, Antes FG, Dressler VL, Morsch VM, Schetinger MRC (2012) Effects of lead on the growth, lead accumulation and physiological responses of Pluchea sagittalis. Ecotoxicology 21(1):111–123
Sarafi E, Tsouvaltzis P, Chatzissavvidis C, Siomos A, Therios I (2017) Melatonin and resveratrol reverse the toxic effect of high boron (B) and modulate biochemical parameters in pepper plants (Capsicum annuum L.). Plant Physiol Biochem 112:173–182
Seth CS, Remans T, Keunen E, Jozefczak M, Gielen H, Opdenakker K, Weyens N, Vangronsveld J, Cuypers A (2012) Phytoextraction of toxic metals: a central role for glutathione. Plant Cell Environ 35(2):334–346
Sharma A, Shahzad B, Kumar V, Kohli SK, Sidhu GPS, Bali AS, Kapoor D, Bhardwaj R, Zheng B (2019a) Phytohormones regulate accumulation of osmolytes under abiotic stress. Biomolecules 9(7):285
Sharma A, Shahzad B, Rehman A, Bhardwaj R, Landi B, Zheng B (2019b) Response of phenylpropnoid pathway and the role of polyphenols in plants under abiotic stress. Biomolecules 24(13):2452
Sharma A, Zheng B (2019) Melotonin mediated regulation of drought stress: physiological and molecular aspects. Plants 8(7):190
Talukdar D (2016) Exogenous thiourea modulates antioxidant defense and glyoxalase systems in lentil genotypes under arsenic stress. J Plant Stress Physiol 2:9–21
Velikova V, Yordanov I, Edreva A (2000) Oxidative stress and some antioxidant systems in acid rain-treated bean plants: protective role of exogenous polyamines. Plant Sci 151(1):59–66
Wang P, Yin L, Liang D, Li C, Ma F, Yue Z (2012) Delayed senescence of apple leaves by exogenous melatonin treatment: toward regulating the ascorbate-glutathione cycle. J Pineal Res 53(1):11–20
Wang Q, Liang X, Dong Y, Xu L, Zhang X, Kong J, Liu S (2013) Effects of exogenous salicylic acid and nitric oxide on physiological characteristics of perennial ryegrass under cadmium stress. Plant Growth Regul 32:721–731
Weeda S, Zhang N, Zhao X, Ndip G, Guo Y, Buck GA, Fu C, Ren S (2014) Arabidopsis transcriptome analysis reveals key roles of melatonin in plant defense systems. PloS ONE 9(3):e93462
Xu LL, Fan ZY, Dong YJ, Kong J, Bai XY (2015) Effects of exogenous salicylic acid and nitric oxide on physiological characteristics of two peanut cultivars under cadmium stress. Biol Plant 59:171–182
Zhou J, Jiang Z, Ma J, Yang L, Wei Y (2017) The effects of lead stress on photosynthetic function and chloroplast ultrastructure of Robinia pseudoacacia seedlings. Environ Sci Pollut R 24(11):10718–10726
Acknowledgements
This work was supported by the funding of Research Council of the Shahr-e-Qods Branch of Islamic Azad University. Special acknowledgements are given to the editors and reviewers. Also we specially thank Dr. Sajjad Sedaghat, for invaluable facilities and support.
Author information
Authors and Affiliations
Corresponding author
Ethics declarations
Conflict of interest
The authors declare that they have no conflict of interest.
Ethical approval
This article does not contain any studies with animal.
Informed consent
Informed consent was obtained from all individual participants included in the study.
Additional information
Publisher’s note Springer Nature remains neutral with regard to jurisdictional claims in published maps and institutional affiliations.
Rights and permissions
About this article
Cite this article
Namdjoyan, S., Soorki, A.A., Elyasi, N. et al. Melatonin alleviates lead-induced oxidative damage in safflower (Carthamus tinctorius L.) seedlings. Ecotoxicology 29, 108–118 (2020). https://doi.org/10.1007/s10646-019-02136-9
Accepted:
Published:
Issue Date:
DOI: https://doi.org/10.1007/s10646-019-02136-9