Abstract
China faces significant challenges in reconciling food security goals with the objective of becoming a low-carbon economy. Agriculture accounts for approximately 11 % of China’s national greenhouse gas (GHG) emissions with cereal production representing a large proportion (about 32 %) of agricultural emissions. Minimizing emissions per unit of product is a policy objective and we estimated the GHG intensities (GHGI) of rice, wheat and maize production in China from 1985 to 2010. Results show significant variations of GHGIs among Chinese provinces and regions. Relative to wheat and maize, GHGI of rice production is much higher owing to CH4 emissions, and is more closely related to yield levels. In general, the south and central has been the most carbon intensive region in rice production while the GHGI of wheat production is highest in north and northwest provinces. The southwest has been characterized by the highest maize GHGI but the lowest rice GHGI. Compared to the baseline scenario, a 2 % annual reduction in N inputs, combined with improved water management in rice paddies, would mitigate 17 % of total GHG emissions from cereal production in 2020 while sustaining the required yield increase to ensure food security. Better management practices will entail additional gains in soil organic carbon further decreasing GHGI. To realize the full mitigation potential while maximizing agriculture development, the design of appropriate policies should accommodate local conditions.
Similar content being viewed by others
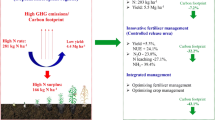
Explore related subjects
Discover the latest articles, news and stories from top researchers in related subjects.Avoid common mistakes on your manuscript.
1 Introduction
China has made substantial efforts to increase crop production to feed about 20 % of the global population with only 8 % of the world’s arable land (World Bank 2013). Looking towards 2020, the government has set a target of increasing the national grain production capacity to over 545 Mt from 497 Mt in 2010 to meet the growing demand for higher animal protein diets and to maintain the domestic food self-sufficiency rate at 95 %. This implies that average grain yield must grow by at least 0.9 % annually in the period 2011–2020. However, Chinese agriculture is grappling with related constraints in terms of limited arable land, declining water availability, increasing opportunity cost of rural labour and increasing vulnerability to climate change (Fan et al. 2011). The sector is also a significant source of anthropogenic greenhouse gases (GHG) emissions emitting approximately 820 Mt CO2 equivalent (CO2e) in 2005, or 11 % of the national total (National Coordination Committee on Climate Change (NCCC) 2012). Cropland N2O emissions produced in soils through the microbial processes of nitrification and denitrification was responsible for 25.3 % of agriculture GHG emissions in 2005 and CH4 emissions from rice cultivation contributed 20 %. Cereal production (rice, wheat and maize) accounted for about 47 % of national N fertilizer consumption (Heffer 2009) and generated around 32 % of GHG emissions from agriculture.
Agriculture is also under increasing scrutiny to mitigate climate change through both emissions reduction and carbon sequestration. The Ministry of Agriculture (MOA) has initiated programs to improve fertilizer use efficiency by 3 % and enhance irrigation water use efficiency by 6 % by 2015 from 2010. The government also plans to bring an additional 11.3 Mha of croplands under conservation tillage between 2009 and 2015 in north China (MOA 2009). The aim of integrating mitigation into agriculture translates into a reduction in GHG intensity (GHGI), expressed as the overall GHG emissions per unit of product (Chen et al. 2011; Venterea et al. 2011; Tubiello et al. 2012). Applying this indicator can encourage better practices resulting in higher crop yields and reduced N losses and GHG emissions, which is vital to pursue low carbon development in agriculture (Norse 2012).
FAO (Tubiello et al. 2014) reported that over the period 1961–2010 the world average GHGI of rice decreased by 49 % while that of wheat and maize increased by 45 %, suggesting that effective mitigation strategies are needed to achieve sustainable intensification; i.e. ensuring that efficiency improvements can lead to reduced absolute emissions. Bonesmo et al. (2012) investigated the GHGI of 95 arable farms in Norway, showing that increased gross margins in grain and oilseed production could be achieved with decreasing GHGI. The GHGIs of cereal production on experimental sites were also quantified in China indicating that economic and climate benefits can be simultaneously achieved by some improved management practices (Shang et al. 2011; Huang et al. 2013; Ma et al. 2013). But to date there is no synthetic estimate of current and historical GHGI of cereal production on a national, regional or provincial level in China. Such information is crucial for identifying efficient regional mitigation strategies and actions tailored to local agricultural production systems and management practices.
This paper estimates GHGI of rice, wheat and maize production using data for the national, regional and provincial scale for 2006. Illustrating the trends and evolution of intensity we quantity national and regional GHGI from 1985 to 2010 at 5-year intervals and analyze emission reduction and carbon sequestration potentials from cereal production. The analysis informs potential national or regional policies to foster sustainable intensification in rural China.
2 Materials and methods
2.1 Methodology
GHGI is calculated by dividing total Global Warming Potential (GWP)-weighted emissions from cereal production by crop yield (Eqn (1)). N2O emissions are accounted for quantifying GHGI of wheat and maize production while both CH4 and N2O are considered for rice paddies. Carbon sequestration is not directly included in the estimate due to large uncertainties in soil organic carbon (SOC) content and limited data availability. Despite consensus on the average SOC increment in China’s cropland, discrepancies in annual intensity change rates have been reported using various methods (Pan et al. 2010; Sun et al. 2010; Yan et al. 2011; Yu et al. 2012). Nevertheless, SOC change patterns and interactions with GHGI will be analyzed in the discussion section. The analysis focuses on emissions within the farm gate, i.e. they are not full life-cycle assessment (e.g. emissions related to energy use and fertilizer manufacture and transportation).
We followed IPCC Guidelines (IPCC 2006) to estimate direct N2O emissions from the three major N input sources - synthetic fertilizers, organic manure and crop residues. Although indirect N2O emissions via N deposition and nitrate leaching and runoff could be significant depending on the local conditions (e.g. Venterea et al. 2011; Maharjan et al. 2014), especially in cases where there is a high rate of N application, they were not taken into account in this study due to high uncertainty. Quantification of CH4 emissions from rice paddies was based on regional CH4 flux.
Where: GHGI (kgCO2e/t); ICH4 is the intensity of CH4 emissions in rice paddies and IN2O is the intensity of N2O emissions (kgCO2e/t); EmissionsN2O is the N2O emissions from rice, wheat or maize fields (kgCO2e/ha); EmissionsCH4 represents the CH4 emissions from rice paddies (kgCO2e/ha); Yield denotes the per hectare average production (t/ha); N2O-Ninput represents the total N inputs (kgN/ha); EF1 is the emission factor for N2O emissions from N inputs (kg N2O–N/kg N input); 44/28 is to convert emissions from kg N2O-N to kg N2O; FluxCH4 represents the CH4 flux from rice paddies (kgCH4/ha); GWPN2O and GWPCH4 denote the direct GWP of N2O and CH4 at the 100 yr horizon, 298 and 25; FSN, FAW, FCR represent N inputs from synthetic fertilizers, animal manure and crop residues, respectively (kgN/ha).
Detailed equations for calculating N inputs from animal manure (FAW) and crop residues (FCR) are presented in Annex A.
2.2 Data sources
Agriculture activity data were collected at the provincial level while emission factors and other parameters (e.g. IPCC default factors) were average national values. In other words, data for N2O-Ninput in Eqn (1) are province-specific and FluxCH4 were region-specific, while other factors were held identical among provinces. Regions in China refer to northeast, north, northwest, east, south and central, and southwest China, each of which includes 3–7 provinces/municipalities. Farming activity data (cropping area, production, yield and total N fertilizer consumption) were extracted from the China Rural Statistical Yearbooks (MOA 1986–2013). Per hectare N application rates for individual crops were collected from the China Agricultural Products Cost-Benefit Yearbooks (National Development and Reform Commission (NDRC) of China 2001), which are the sum of N fertilizer (pure nutrient) and 30 % N fraction in compound and mixed fertilizers. To calculate the national and provincial GHGI in 2006, we used the three-year average of 2005–2007 to represent 2006 conditions to avoid large inter-annual variations. China-specific emission factors for direct N2O emissions from croplands were obtained from Gao et al. (2011), which are 0.0105 and 0.0041 for upland and rice paddies, respectively. CH4 fluxes of rice paddies in each region were direct CH4MOD modeled results from studies by Zhang et al. (2011a), which were employed for compiling National GHG Emission Inventories.
For estimating N inputs from animal manure, livestock numbers and the fraction of grazing animals were derived from the China Livestock Yearbooks (MOA 2001–2011) while other information required was selected from relevant literature and IPCC default values corresponding to conditions in China as displayed in Table S1. Estimation of N inputs from crop residues were mainly based on values reported by Gao et al. (2011) summarized in Table S2. Detailed information for data selection is provided in Annex B.
Regional level SOC data in 2010 were derived from Yu et al. (2013) to represent 2006 levels, and historic SOC contents were derived from similar research by Yu et al. (2012).
2.3 Design of emission scenarios for future cereal production
To project total GHG emissions and investigate mitigation potential from cereal production in China to 2020, we designed four agricultural management scenarios based on historical trends and the increase in expected future productivity. Total GHG emissions shall be affected by the GHGI and grain production, or N input and CH4 flux levels, yield and cultivated area of each crop.
To focus on the impacts of GHGI change on overall emissions, cultivated area of each crop were assumed constant from 2010 to 2020. In all scenarios, 0.5, 1 and 1.5 % annual increase in yield were assigned for rice, wheat and maize respectively, based on 2005–2013 yield data released by the MOA (2006–2013). S0 is a conservative scenario that prescribes the same proportion of increase in N input relative to yield improvement. Scenario S1 assumes that no further N input is required to sustain equal productivity as in S0, while the N rate decreases by 1 % per year under S2. Scenario S3 is an optimal scenario incorporating best management practices to cut the overall N rates and improve the irrigation regimes in rice paddies while achieving the yield requirements for safeguarding national food self-sufficiency (annual N input decrease at 2 % and CH4 flux decrease at 1 %). The annual rates of change for these factors over 2010–2020 are summarized in Table 1.
3 Results and discussions
3.1 GHGI of rice production in 2006
GHGI of rice production in 2006 ranged from 730 kgCO2e/t in Ningxia Province to 1,549 kgCO2e/t in Hainan Province, with a national average of 947 kgCO2e/t (Fig. 1a). In general, CH4 made up about 90 % of the total GHG emissions and was therefore the dominant gas in determining the carbon footprint of rice cultivation. Consequently, there was no obvious relationship between GHGI levels and N application rates, the latter being the major source of N2O emissions. For example, the Jiangsu Province in east China received 51 % higher N application than national average in rice production but was moderate in GHGI (16 % lower than national average). It is, however, evident that the estimated GHGI for rice production was negatively correlated with yield levels. There was a large provincial variation in GHGI (Fig. 2a) with the most carbon intensive provinces located in the southeast coastal areas due to the highest regional CH4 flux (250 kg/ha) because of higher temperature and greater level of organic matter input (Zhang et al. 2011a). The low GHGI in the southwestern provinces (Sichuan, Chongqing, Guizhou and Yunnan) can be attributed to lower CH4 flux (200 kg/ha) relative to other places (215–250 kg/ha) because of low levels of organic matter application and rice biomass productivity. Among the six major rice producing provinces, which accounted for 55 % of the national production, Hunan and Jiangxi had higher GHGIs than the national average, while Hubei, Jiangsu, Sichuan and Heilongjiang were below the national mean.
3.2 GHGI of wheat and maize production
The national average GHGI of wheat (Fig. 1b) and maize (Fig. 1b) for 2006 production were 265 and 230 kgCO2e/t, respectively. Large spatial variability can be observed among provinces. For example, producing one ton of wheat in Ningxia emitted 3 times more N2O than in Heilongjiang, attributable to significant differences in synthetic N input and wheat and maize yields between Chinese provinces. In general, synthetic N fertilizer made up at least 70 % of total emissions and was therefore the primary emission contributor. Figure 1b and c also shows that the trends of GHGI, which are affected by place-specific yield levels, were not necessarily consistent with those of per hectare N application rates. For instance, although the N application rate for maize in Ningxia (280 kgN/ha) was 30 % higher than in Guangxi (215 kgN/ha), a much higher yield in Ningxia (6.97 t/ha) than in Guangxi (3.88 t/ha) resulted in a lower maize GHGI in Ningxia. In contrast, a high N rate and low wheat productivity made Ningxia the most carbon intensive province for wheat cultivation.
The geographic variations of GHGIs of wheat (Fig. 2b) and maize (Fig. 2c) show both similarities and differences. In general, similar levels of GHGI can be observed for wheat and maize production (except for Ningxia); e.g. Yunnan was one of the most carbon intensive areas for both wheat and maize production in 2006. More N fertilizers were added to croplands in the northwest provinces to compensate poor soil fertility, resulting in elevated regional GHGI of wheat and maize production. The levels of maize GHGI converged to the range of 200–300 kgCO2e/t, with obvious correlation with N rates and yields. Provincial discrepancies were more evident for wheat GHGI, implying that farmers were potentially more rational in determining the fertilizer amount for maize than for wheat. Among the five major wheat producing areas - Henan, Shandong, Hebei, Anhui and Jiangsu, which contributed about 73 % of the national production, GHGI levels in Hebei and Jiangsu were superior to the national average. Among the major maize producing areas, only Hebei had a higher GHGI than the national mean, while Jilin, Shandong, Henan and Heilongjiang were lower.
GHGIs at the provincial level were further aggregated to the regional scale for 2006 and compared with yields and SOC contents (Fig. S1) to indicate regional GHGI reduction strategies (Annex C).
3.3 Historical trends of regional GHGI of cereal production
Figure 3a shows that national GHGI of rice production evolved at a different way to those of wheat and maize production, and the latter has always been the least carbon intensive of the three crops. Rice GHGI saw little variation between 1985 and 2000, which can be explained by nearly the same rate of growth in the CH4 flux, yield (Fig. 3b) as well as the N rate over this period. However, when rice yield reached a periodic peak in 1998 the CH4 flux continued to climb, resulting in a sharp rise in GHGI in the first decade of the 21st century. Wheat and maize GHGIs had been steadily increasing from 1985 to 2000 since the growth rate of N application exceeded the rate of yield improvement. The GHGI began to stablize or even decrease after 2000 as the combined effects of increasing yields, abeit at a lower rate, and stabilized synthetic N rates promoted by the national “Soil testing and fertilizer recommendation program” (MOA 2005) initiated in 2005. At the national level, there was also a positive correlation between SOC improvement and cereal productivity increase (Fig. 3b) (Pan et al. 2009).
Figure 4 illustrates that nearly all regional GHGIs of rice, wheat and maize production reached a higher level in 2010 relative to 1985. For rice production (Fig. 4a), south and central and east regions have consistently been the most carbon intensive areas due to favorable climate conditions and greater level of organic matter application (Zhang et al. 2011a). In parallel, rice paddies in eastern, southern and central China are found to have experienced the greatest SOC increase (Zhang et al. 2007; Pan et al. 2010). In contrast, a lower level of crop residues, farm manure and green manure application enabled the southwest to emit least GHG in producing same amount of rice.
For wheat production (Fig. 4b), all regions except north China exhibited the same trend as the national average. Consequently, reducing N rates should be advocated in northern provinces, confirming the findings of other experimental and theoretical studies (Ju et al. 2009; Ju and Christie 2011). Maize GHGI evolution patterns (Fig. 4c) were more diverse between geographic regions, with northeast China having the lowest GHGI. The northwest has been characterized with the highest GHGI in both wheat and maize production.
3.4 Ways to improve GHGI of cereal production while safeguarding food security
Integrated soil-crop management systems and better nutrient management techniques are advocated to address the key constraints to yield improvement and alleviate environmental impacts (Fan et al. 2011; Zhang et al. 2012). Extensive overuse of synthetic N fertilizers is well documented in China (Cui et al. 2010; Chen et al. 2011), resulting in significant losses and serious environmental externalities (Guo et al. 2010). Zhang et al. (2013) suggest a possible 42 % cut in nationwide N fertilizer use applying the balance concept to equalize N input and above ground N removal. In parallel to optimal quantity, application time, right placement and appropriate product are also essential to better nutrient management. Adjusting basal/topdressing ratio of N fertilizers and popularizing fertilizer deep placement could improve crop N uptake and minimize N losses compared with conventional practices of applying largest proportion of N fertilizers on the surface before seeding (Cui et al. 2008; Zhang et al. 2011b). Replacing a proportion of ammonium-based fertilizers with nitrate-based fertilizers can also help minimize N2O emissions and ammonia losses (Zhang et al. 2013). Nitrogen use efficiency (NUE) can also be improved by applying fertilizers added with nitrification and/or urease inhibitors and slow- and controlled-released fertilizers (Akiyama et al. 2010).
Better recycling of organic manures including animal excreta, crop residues and green manure enables further improvement in NUE, SOC content and land productivity. Adopting conservation tillage is found to be conducive to accumulating SOC, improving water availability and reducing water and wind erosion, especially on land of poor productivity (Xu et al. 2007; He et al. 2010). Such practices shall be extended to wider areas supported by the MOA. Finally, biochar addition can be beneficial to soil quality and yield increase therefore offering substantial mitigation potential when it becomes economically available (Wang et al. 2014). As to CH4 emissions from rice paddies, upgrading irrigation regimes from mid-season drainage, currently being practiced in most rice cultivation regions, to intermittent irrigation or controlled irrigation, could avoid as much as 1.256 CO2e per hectare according to nationwide meta-analysis results (Wang et al. 2014).
In addition to mitigating climate change, some of these measures could actually be cost saving, simultaneously reducing input costs and/or enhancing productivity (Wreford et al. 2010; Wang et al. 2014). Further, in recent decades SOC content of cropland has increased along with improved crop yields in most regions of China (Pan et al. 2010; Yan et al. 2011; Yu et al. 2012). These findings highlight the important role of cropland in achieving emission reduction, safeguarding food security and enhancing carbon sequestration.
3.5 Implication for mitigation potential from cereal production
Figure 5 illustrates that total GHG emissions from rice, wheat and maize production have grown by 12 % from 2005 to 2010 caused by an 11 % increase in cropping area and a 5 % increase in average yield (Fig. 3b). In the S0 baseline scenario, although yields improve at the same rate of increase in N inputs, resulting in constant GHGI, total GHG emissions will still go up because of higher production levels.
However, if no more N input is needed to enhance yields, emissions will stop increasing (scenario S1) and GHGIs will decrease. In contrast, if better fertilization practices are promoted to suppress the overuse of N fertilizers, total emissions will decline (scenario S2) by 8 % compared to S0. Scenario S3 assumes substantial efforts are dedicated to minimizing the GHGI of cereal production by eradicating N over-application, adopting better water management in rice paddies and improving yield levels. In this case, IN2O of rice, wheat and maize shall decline by 2.5, 3 and 3.4 % respectively, and ICH4 by 1.5 % annually. Under this scenario, total GHG emissions are estimated to be 224MtCO2e, a 17 % decrease relative to S0 enabled by an 18 % decrease in N input, 0.5–1.5 % improvement in yields and 1 % cut in average CH4 flux. Such a mitigation scenario is feasible since the 18 % cut in N use falls under the lower range of suggested 30–60 % reduction (Ju and Christie 2011; Zhang et al. 2013) and the 546 Mt cereal production meets the target for ensuring national food security.
Apart from the emission reduction potential, SOC density is projected to continue to increase at a rate of 0.4–0.48 tC/ha/yr in paddy soils and 0.16–0.22 tC/ha/yr in upland soils in the 2010s (Yu et al. 2013). This implies that even the C inputs (including manure and crop residue) to Chinese croplands remain unchanged with no improvement in tillage practices, aggregate national SOC stocks will still increase over the period 2010–2020. If improved agricultural management practices are widely adopted, as much as 70MtCO2 could be sequestrated in the cropland soils. Carbon sequestration is therefore able to compensate 31 % of GHG emissions under scenarios S3.
4 Conclusions
A low carbon development pathway implies minimization of emissions while increasing food production and GHGI is an indicator combining both objectives. As such it is a central element of any definition of sustainable intensification (Godfray and Garnett 2014). Our results on the GHGI of rice, wheat and maize production show that the southeast was the most carbon- intensive region in rice production in terms of CH4 emissions, while GHGI of wheat and maize were both high in most north and northwest provinces due to the typical farming practices of agricultural systems in China. GHGI was low for all the three crops in the northeast area. The substantial heterogeneities of GHGI among provinces/regions and the inconsistency between trends of GHGIs and N application rates indicate considerable scope for improving carbon performance of cereal production and that actions and policies aiming to promote sustainable food production should be tailored to local conditions. Under the baseline scenarios where food production must grow to meet the demand of about 1.45 billion population, total GHG emissions will continue to increase albeit with constant GHGIs. Controlling GHG emissions from arable land thus requires additional mitigation efforts. Most abatement practices that improve crop yields will not only enable emission reductions but also improve soil fertility via carbon sequestration, therefore providing a triple win. Such findings can inform a broad range of policy, practitioner and investment discussions on GHG mitigation strategies, and can also serve as benchmark values for allocating quotas or as the baseline for generating carbon credits for any market-based mechanism.
Despite positive synergies with yield and soil fertility, abatement measures have not been widely adopted by farmers due to economic, political and social factors. Required capacity and infrastructure must be improved and agricultural extension service upgraded to lower GHGI and realize the mitigation potential and land productivity and fertility improvement potential that agricultural production offers.
References
Akiyama H, Yan XY, Yagi K (2010) Evaluation of effectiveness of enhanced-efficiency fertilizers as mitigation options for N2O and NO emissions from agricultural soils: meta-analysis. Glob Chang Biol 16:1837–1846
Bonesmo H, Skjelvåg AO, Janzen HH, Klakegg O, Tveito OE (2012) Greenhouse gas emission intensities and economic efficiency in crop production: a systems analysis of 95 farms. Agric Syst 110:142–151
Chen XP, Cui ZL, Vitousek PM et al (2011) Integrated soil-crop system management for food security. Proc Natl Acad Sci 108:6399–6404
Cui ZL, Zhang FS, Chen XP et al (2008) On-farm evaluation of an in-season nitrogen management strategy based on soil Nmin test. Field Crop Res 105:48–55
Cui ZL, Chen XP, Zhang FS (2010) Current nitrogen management status and measures to improve the intensive wheat–maize system in China. Ambio 39:376–384
Fan MS, Shen JB, Yuan LX et al (2011) Improving crop productivity and resource use efficiency to ensure food security and environmental quality in China. J Exp Bot 63(1):13–24
Gao B, Ju XT, Zhang Q, Christie P, Zhang FS (2011) New estimates of direct N2O emissions from Chinese croplands from 1980 to 2007 using localized emission factors. Biogeosci Discuss 8:6971–7006
Godfray HCJ, Garnett T (2014) Food security and sustainable intensification. Phil Trans R Soc B Biol Sci 369(1639):20120273
Guo JH, Liu XJ, Zhang Y et al (2010) Significant acidification in major Chinese. Croplands Sci 327:1008–1010
He J, Li HW, Wang QJ et al (2010) The adoption of conservation tillage in China. Ann N Y Acad Sci 1195:E96–E106
Heffer P (2009) Assessment of fertilizer use by crop at the global level. International Fertilizer Industry Association
Huang T, Gao B, Christie P, Ju XT (2013) Net global warming potential and greenhouse gas intensity in a double-cropping cereal rotation as affected by nitrogen and straw management. Biogeosciences 10:897–7911
IPCC (2006) IPCC guidelines for national greenhouse gas inventories IPCC/IGES. Hayama, Japan
Ju XT, Christie P (2011) Calculation of theoretical nitrogen rate for simple nitrogen recommendations in intensive cropping systems: a case study on the North China plain. Field Crop Res 124:450–458
Ju XT, Xing GX, Chen XP et al (2009) Reducing environmental risk by improving N management in intensive Chinese agricultural systems. Proc Natl Acad Sci 106:3041–3046
Ma YC, Kong XW, Yang B et al (2013) Net global warming potential and greenhouse gas intensity of annual rice-wheat rotations with integrated soil–crop system management. Agric Ecosyst Environ 164:209–219
Maharjan B, Venterea RT, Rosen C (2014) Fertilizer and irrigation management effects on nitrous oxide emissions and nitrate leaching[J]. Agron J 106(2):703–714
Ministry of Agriculture (MOA) (1986–2013) China rural statistical yearbook. China Agricultural Press, Beijing
MOA (2001–2011) China livestock yearbook. China Agricultural Press, Beijing
MOA (2005) Notice on the issuance of “Interim management measures of subsidy funds for fertilizer recommendation pilots
MOA (2009) Conservation Tillage Construction Plan 2009–2015
National Coordination Committee on Climate Change (NCCC) (2012) Second national communication on climate change of the PRC. China Planning Press, Beijing
National Development and Reform Commission (NDRC) of China (2001) China agricultural products cost-benefit yearbooks. China Statistics Press, Beijing
Norse D (2012) Low carbon agriculture: objectives and policy pathways. Environ Develop 1:25–39
Pan GX, Smith P, Pan W (2009) The role of soil organic matter in maintaining the productivity and yield stability of cereals in China. Agric Ecosyst Environ 129:344–348
Pan GX, Xu X, Smith P, Pan W, Lal R (2010) An increase in topsoil SOC stock of China’s croplands between 1985 and 2006 revealed by soil monitoring. Agric Ecosyst Environ 136:133–138
Shang Q, Yang X, Gao C, Wu P et al (2011) Net annual global warming potential and greenhouse gas intensity in Chinese double rice-cropping systems: a 3-year field measurement in long-term fertilizer experiments. Glob Chang Biol 17:2196–2210
Sun W, Huang Y, Zhang W, Yu Y (2010) Carbon sequestration and its potential in agricultural soils of China. Glob Biogeochem Cycles 24(3):GB3001
Tubiello FN, Salvatore M, Rossi S, Ferrara A (2012) Analysis of global emissions, carbon intensity and efficiency of food production. EAI research papers
Tubiello FN, Salvatore M, Cóndor RD et al. (2014) Agriculture, Forestry and Other Land Use Emissions by Sources and Removals by Sinks, 1990–2011 Analysis. FAO Working Paper Series ESS/14- 02
Venterea RT, Maharjan B, Dolan MS (2011) Fertilizer source and tillage effects on yield-scaled nitrous oxide emissions in a corn cropping system. J Environ Qual 40:1521–1531
Wang W, Koslowski F, Nayak DR et al (2014) Greenhouse gas mitigation in Chinese agriculture: distinguishing technical and economic potentials. Glob Environ Chang 26:53–62
World Bank (2013) World Bank Data http://data.worldbank.org/, accessed June 2013
Wreford AD, Moran D, Adger N (2010) Climate Change and Agriculture: Impacts, Adaptation and Mitigation. OECD Publishing
Xu Y, Chen W, Shen Q (2007) Soil organic carbon and nitrogen pools impacted by long-term tillage and fertilization practices. Commun Soil Sci Plant Anal 38:347–357
Yan XY, Cai ZC, Wang SW, Smith P (2011) Direct measurement of soil organic carbon content change in the croplands of China. Glob Chang Biol 17:1487–1496
Yu YQ, Huang Y, Zhang W (2012) Modeling soil organic carbon change in croplands of China, 1980–2009. Glob Planet Chang 82–83:115–128
Yu YQ, Huang Y, Zhang W (2013) Projected changes in soil organic carbon stocks of China’s croplands under different agricultural managements: 2011–2050. Agric Ecosyst Environ 178:109–120
Zhang W, Yu YQ, Sun WJ, Huang Y (2007) Simulation of soil organic carbon dynamics in Chinese rice paddies from 1980 to 2000. Pedosphere 17:1–10
Zhang W, Yu Y, Huang Y, Li T, Wang P (2011a) Modeling methane emissions from irrigated rice cultivation in China from 1960 to 2050. Glob Chang Biol 17:3511–3523
Zhang FS, Cui ZL, Fan MS et al (2011b) Integrated soil-crop system management: reducing environmental risk while increasing crop productivity and improving nutrient use efficiency in China. J Environ Qual 40:1–7
Zhang FS, Cui ZL, Chen XP et al. (2012) Integrated Nutrient Management for Food Security and Environmental Quality in China. In: Sparks, DL (ed) Advances in Agronomy 116, pp 1–40
Zhang WF, Dou ZX, He P et al (2013) New technologies reduce greenhouse gas emissions from nitrogenous fertilizer in China. Proc Natl Acad Sci 110:8375–8380
Acknowledgments
This study is funded by the Chinese Ministry of Science and Technology (2013BAD11B03), the Climate Economics Chair of Paris-Dauphine University and the Agricultural Science and Technology Innovation Program of CAAS. D.M. acknowledges support from the SmartSOIL EU FP7 project (grant number 289694).
Author information
Authors and Affiliations
Corresponding author
Electronic supplementary material
Below is the link to the electronic supplementary material.
ESM 1
(DOCX 68.3 kb)
Rights and permissions
About this article
Cite this article
Wang, W., Guo, L., Li, Y. et al. Greenhouse gas intensity of three main crops and implications for low-carbon agriculture in China. Climatic Change 128, 57–70 (2015). https://doi.org/10.1007/s10584-014-1289-7
Received:
Accepted:
Published:
Issue Date:
DOI: https://doi.org/10.1007/s10584-014-1289-7