Abstract
Voltage-sensitive calcium channels (VSCCs) underlie cell excitability and are involved in the mechanisms that generate and maintain neuropathic and inflammatory pain. We evaluated in rats the effects of two VSCC blockers, ω-conotoxin MVIIA and Phα1β, in models of inflammatory and neuropathic pain induced with complete Freund’s adjuvant (CFA) and chronic constrictive injury (CCI), respectively. We also evaluated the effects of the toxins on capsaicin-induced Ca2+ influx in dorsal root ganglion (DRG) neurons obtained from rats exposed to both models of pain. A single intrathecal injection of Phα1β reversibly inhibits CFA and CCI-induced mechanical hyperalgesia longer than a single injection of ω-conotoxin MVIIA. Phα1β and MVIIA also inhibited capsaicin-induced Ca2+ influx in DRG neurons. The inhibitory effect of Phα1β on capsaicin-induced calcium transients in DRG neurons was greater in the CFA model of pain, while the inhibitory effect of ω-conotoxin MVIIA was greater in the CCI model. The management of chronic inflammatory and neuropathic pain is still a major challenge for clinicians. Phα1β, a reversible inhibitor of VSCCs with a preference for N-type Ca2+ channels, has potential as a novel therapeutic agent for inflammatory and neuropathic pain. Clinical studies are necessary to establish the role of Phα1β in the treatment of chronic pain.
Similar content being viewed by others
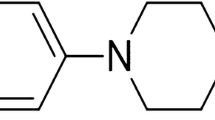
Avoid common mistakes on your manuscript.
Introduction
Pain is an unpleasant sensory and emotional experience associated with actual or potential tissue damage (definition promoted by the International Association for the Study of Pain). The treatment of pain requires an understanding of the mechanisms by which the pain signals are initially interpreted, transmitted and maintained. Pain is divided in two main categories: acute and chronic. The latter is classified in inflammatory and neuropathic pain, which have the same nerve pathways of transmission, but significant physiologic differences exist in the mechanisms through which they are biologically processed and resolved (Katherine and Galluzzi 2007). Several neurotransmitters mediate transmission of pain sensation in the brain and spinal cord such as glutamate, norepinephrine, serotonin, substance P, calcitonin gene-related peptide (CGRP), vasoactive intestinal polypeptide, somatostatin and bombesin. Glutamate, substance P, norepinephrine and serotonin are the most important neurotransmitters involved in inflammatory and neurophatic pain (Phillips and Clauw 2011).
The afferent transmission of pain signal to the central nervous system (CNS) is mediated by voltage-sensitive Ca2+ channels (VSCCs), which control the release of neurotransmitters from presynaptic terminals of dorsal root ganglion (DRG) neurons in the dorsal horn upon arrival of an action potential (Julius and Basbaum 2001). VSCCs are important for supplying the necessary Ca2+ of the increased sensory nerve excitability as well as the complex patterns of neurotransmitter release associated with the chronic pain syndrome (Gribkoff et al. 2005). In fact, intracellular Ca2+ acts as a second messenger within neurons regulating several physiological process including neurotransmitter release, excitability, enzyme activity and gene expression (Berridge et al. 2000).
Substance P and CGRP are nociceptive neurotransmitters that are released upon activation of L-, N-, and P/Q-type VSCCs in sensitive neurons (Lee et al. 2005). The N-Type (Cav2.2 channel) is found in presynaptic nerve terminals regulating the Ca2+ influx during the depolarization-induced transmitter release from both central and peripheral nerves (Miljanich and Ramachandran 1995). N-type VSCC are abundantly expressed in the superficial laminae (I and II) of the spinal cord dorsal horn, where they play an important role on the spinal processing of nociceptive afferent activity (Bowersox et al. 1996).
The role of the N-type VSCC has been investigated in the development of novel analgesics (Altier and Zamponi 2004). In fact, the expression of Cav2.2 channels is up-regulated in some chronic pain states, suggesting a role of these channels on sensory signaling of this type of pain (Cizkova et al. 2002). The spinal administration of ω-conotoxin MVIIA, a cone snail Conus magus toxin that blocks Cav2.2, reduced the hyperalgesia from nerve injury or inflammation in preclinical behavioral models of chronic pain (Malmberg and Yaksh 1995; Bowersox et al. 1996; Scott et al. 2002). Moreover, the intrathecal administration of the spider Phα1β, a reversible inhibitor of N-type VSCCs (Vieira et al. 2005), induced an analgesic effect in rodent models of acute and chronic pain with few adverse effects (Souza et al. 2008). Therefore, the current study was designed to evaluate the antinociceptive effect of Phα1β and ω-conotoxin MVIIA in a model of inflammatory and neuropathic pain induced by Complete Freund’s adjuvant (CFA) and chronic constrictive injury (CCI), respectively, in rats. The effects of the toxins on capsaicin-induced Ca2+ influx in dorsal root ganglion (DRG) neurons of rats exposed to both models of pain was also investigated.
Materials and Methods
Drugs
The toxin Phα1β was purified from the venom using HPLC AKTA purifier 10 (GE Healthcare Bio-Sciences Corp, Piscataway, New Jersey, USA) by a combination of preparative reverse phase chromatography on Vydac C4 column (300 angstroms, 250 × 22 mm, 5 microns), ion-exchange high-performance liquid chromatography on cationic and anionic columns (Synchropak S and Synchropak Q, both 250 × 4.6 mm, 300 Å, 5 microns) and reverse phase high-performance liquid chromatography on Vydac C18 (Small Pore 90 Å, 250 × 4,6 mm, 5 micron). The detection was done by a monitor UV ranging from 190 to 700 nm (Cordeiro Mdo et al. 1993). ω-conotoxin MVIIA was purchased from Latoxan (Valence, France). The stock solutions of the toxins were prepared in phosphate buffer saline (PBS) in siliconized plastic tubes, maintained at −18 °C and diluted to the desired concentration just before use. CFA (1 mg/ml of heat killed Mycobacterium tuberculosis in 85 % paraffin oil and 15 % mannide monooleate), papain, collagenase type 1A, bovine calf serum and Dulbecco’s modified Eagle medium (DMEM) were obtained from Sigma (St. Louis, MO, USA). Fluo 4-AM was purchased from Molecular Probes (Eugene, OR, USA). Nerve growth factor (NGF) 7S was obtained from Alomone Labs (Israel). Penicillin and streptomycin were purchased from Invitrogen (Carlsbad, CA, USA) the other reagents were of analytical grade.
Animals
The experiments were performed according to the current guidelines for the care of laboratory animals and for investigations of experimental pain in conscious animals (Zimmermann 1983). They were authorized through the Ethics Committee of the Universidad Federal de Minas Gerais, Protocol 179/06. Male adult Wistar rats (180–250 g) were kept housed in plastic cages (six per cage) in a home care environment during at least 1 week before the experimental protocol. Only the animals that remained in good health conditions throughout this period as assessed by appearance, behavior, and maintenance of body weight were used in the experiments. The temperature was maintained at 22 ± 1 °C, and the room illumination was maintained on a 12/12 h light/dark cycle.
Intrathecal Injection
Intrathecal injections were performed in accordance with the method previously described (Mestre et al. 1994). Briefly, 10-μl of ω-conotoxin MVIIA, Phα1β or vehicle (PBS) was administered with a 28-gauge needle through a Hamilton microsyringe. The animals were gently immobilized in order to maintain the position of the needle. Puncture of the dura mater was indicated behaviorally by a slight flick of the tail.
Inflammatory Pain Induced by CFA
CFA-induced inflammatory pain was elicited as described (Wilson et al. 2006). Briefly, rats were anesthetized with halothane, and 150 μl of CFA was subcutaneously injected into the ventral surface of the right hind paw (intraplantar injection). Forty-eight hours later we measured the basal mechanical hyperalgesia. After this time, we did the intrathecal administration of Phα1β (200 pmol/site), ω-conotoxin MVIIA (100 pmol/site) or PBS. Mechanical hyperalgesia was measured from one to 24 h after the administration of the treatments.
Neuropathic Pain Induced by Chronic Constrictive Injury (CCI)
The rats were first anesthetized by intraperitoneal injection of a mixture of ketamine (50 mg/kg) and xylazine (10 mg/kg). Neuropathic pain was induced by ligation of sciatic nerve as previously described (Bennett and Xie 1988). After surgical preparation, the common sciatic nerve was exposed at the middle of the thigh by blunt dissection through the biceps femoral. Proximal to the sciatic trifurcation, 7 mm of the nerve was freed from adhesions and four sutures (4-0 silk) were loosely tied with 1 mm space between them. In sham-operated rats, the nerve was exposed without ligation. Fifteen days after the surgical procedure, the mechanical sensitivity was measured to confirm the development of hyperalgesia.
Measurement of Mechanical Hyperalgesia Induced by CFA or CCI
The evaluation of mechanical paw withdrawal threshold was carried out using the up and down paradigm as previously described by Chaplan et al. (1994). Briefly, the rats were first acclimatized (1 h) in individual clear Plexiglas boxes (9 × 7 × 11 cm) on an elevated wire mesh platform to allow the access to the plantar surface of the hind paws. Von Frey filaments of increasing stiffness (6–100 g) were applied to surface plantar of the rat hind paw with a pressure to cause a bend on the filament. The absence of a paw lifting after 5 s resulted in the use of the next filament with increasing weight. However paw lifting indicated a positive response, and resulted the use of the next weaker filament. This paradigm continued until a total of six measurements, including the initial measurement taken before the first paw-lifting response, were collected or until four consecutive positive (assigned a score of 7.14 g) or four consecutive negative responses (assigned a score of 84.02 g) had occurred. The 50 % mechanical paw withdraw threshold (PWT) response was them calculated from the resulting scores as described by Dixon (1980).
Neuronal Culture
For CFA inflammatory pain model, culture was prepared 3 days after CFA injection and for CCI neuropathic pain model, the culture was performed 15 days after CCI surgery. During this period the animals were monitored according to their appearance, behavior, and maintenance of body weight. Before the sacrifice, the animals were tested for the quantification of the individual sensory threshold through mechanical noxious stimulation. Lumbar dorsal root ganglion (L4–L6, ipsilateral to the injury) was removed from the rats and dissociated into single isolated neurons through enzymatic digestion with 0.1 % papain for 20 min. Thereafter, a digestion process was done with 0.25 % collagenase type 1A for 30 min with light agitation. The cells were then resuspended using several passes through a fire-polished Pasteur pipette before plating onto culture dishes on Poly-l-lysine/laminin-coated coverslips. Subsequently, they were incubated in DMEM supplemented with 10 % heat-inactivated bovine calf serum, 1 % penicillin/streptomycin and 100 ng/ml of NGF. The neurons were maintained in culture for a short period before the experiment (12–24 h) at 37 °C in a water-saturated atmosphere with 5 % CO2 (Gomes et al. 2004).
Ca2+ Fluorescence Imaging
Experiments were performed at room temperature (20–25 °C) essentially as described previously (Gomes et al. 2004). Fluo-4, a Ca2+ dye was used as indicator of changes on intracellular Ca2+ concentration ([Ca2+]i). The cells were platted on cover slips were loaded with 3 μM Fluo 4-AM for 30 min in HEPES buffered salt solution (HBSS) containing (mM): 124 NaCl, 4 KCl, 2 CaCl2, 1.2 MgCl2, 10 glucose, 25 HEPES, pH 7.4. The cover slips were washed in dye-free HBSS, transferred to a superfusion chamber system (Bioptechs) and examined using microscopy. HBSS (alone or with the treatments) was continuously perfused (0.6 ml/min) by a peristaltic pump (Bioptechs) during the imaging acquisition. Imaging was performed with a Leica SP5 laser scanning confocal system running the software LAS with a 20× water immersion objective lens. Fluo 4-AM was imaged by excitation with a 488 nm argon laser line. Light emitted was collected in 510–570 nm emission bands. Cells were stimulated with brief pulses (20 s) of capsaicin (1 μM) because it has the ability to activate nociceptive fibers (Eun et al. 2001). Toxins were added 10 min before capsaicin stimulus. The quantification of the responses was normalized to the amplitude of a control stimulus with capsaicin without antagonists. Only cells that recovered to basal levels of fluorescence were analyzed. In addition, only DRG neurons with diameters <25 μm and capsaicin responsive cells (increase of 50 % on basal fluorescence) were analyzed. The regions of interest (ROI) were identified within the soma and the quantitative measurements were made by re-analysis of stored image sequences using the Leica Application Suite software. Changes in fluorescence (F) were normalized by the initial fluorescence (F 0) of each ROI and were expressed as (F/F 0) × 100 (% of baseline). The values of fluorescence were reported as mean of cells studied in at least three experiments. Significant differences in the Ca2+ increase in response to capsaicin with or without antagonists were obtained by the ratio between second and first capsaicin stimulus (Peak 2/Peak 1).
Data Analysis
The results were expressed as mean ± SEM. Data were analyzed by one or two-way analysis of variance (ANOVA) followed by Bonferroni post test. Mann–Whitney nonparametric test was used for comparison between groups in the experiments of Ca2+ fluorescence in DRGs. Maximal inhibition (Imax) obtained from each individual in vivo experiment was calculated using the formula: (%) inhibition = 100 × [(control − experiment)/control]. The level of significance was set at P < 0.05.
Results
Effect of Phα1β and ω-conotoxin MVIIA on Inflammatory Pain induced by CFA
As shown in Fig. 1a, the 50 % threshold value diminished from 20.3 ± 2.0 (baseline) to 10.7 ± 2.1 (P < 0.001) 48 h after the intraplantar injection of CFA suggesting that it produced a marked mechanical hyperalgesia. The intrathecal administration of Phα1β (200 pmol/site) reduced mechanical hyperalgesia induced by CFA from 2 to 24 h after its administration with an Imax of 100 % at 3 h. On the other hand, ω-conotoxin MVIIA (100 pmol/site) decreased the inflammatory pain induced by CFA for up 6 h after its administration with an Imax of 100 % inhibition at 1 h. The 50 % threshold value for ω-conotoxin MVIIA (100 pmol/site) in the mechanical hyperalgesia 24 h after its administration was lower than that obtained with Phα1β (200 pmol/site; P < 0.05) but was not different from the PBS (control) value (P > 0.05). Thus, the inhibition of mechanical hyperalgesia was longer with Phα1β than ω-conotoxin MVIIA in the model of inflammatory pain (Fig. 1a). The doses of Phα1β (200 pmol/site) and ω-conotoxin MVIIA (100 pmol/site) were chosen according on previous data of doses response curves obtained for the toxins on the nociceptive behavior (Souza et al. 2008). Moreover, intrathecal administration of Phα1β (200 pmol/site) or MVIIA (100 pmol/site) did not alter the normal mechanical sensitivity of the animals (Fig. 1b).
a Time course effects of intrathecal treatments with Phα1β (200 pmol/site) and ω-conotoxin MVIIA (100 pmol/site) on mechanical allodynia induced by intraplantar injection of CFA. The effects of Phα1β and ω-conotoxin MVIIA are expressed as 50 % PWT. b Time course effects of Phα1β (200 pmol/site) and ω-conotoxin MVIIA (100 pmol/site) administration on the mechanical sensitivity in rats without intraplantar injection of CFA. B represents baseline values obtained before intraplantar injection of CFA. The results are given as the mean ± standard error of 8–10 animals. *P < 0.05 and **P < 0.01 represents level of significance when compared to animals treated with PBS (two-way ANOVA followed by the test Bonferroni’s)
Effects of Phα1β and ω-conotoxin MVIIA on CCI-Induced Neuropathic Pain
Figure 2a, c show that the 50 % PWT value was reduced from 40.3 ± 5.3 (baseline) to 13.4 ± 1.63 15 days after the surgery, suggesting that CCI produced a marked mechanical allodynia (P < 0.05). The sham group did not demonstrated mechanical allodynia, and the 50 % PWT values were 53.6 ± 9.5 and 48.1 ± 11.8 before and after the sham procedure, respectively. The intrathecal administration of Phα1β (200 pmol/site) blocked the maintenance of mechanical allodynia in the CCI model (Fig. 2a) for up to 4 h after the treatment. This effect was not due to a loss of mechanical sensitivity since Phα1β did not alter normal mechanical sensitivity of the animals (Fig. 2b). Similarly, the intrathecal treatment with ω-conotoxin MVIIA (100 pmol/site) inhibited mechanical allodynia in the CCI model for up to 4 h after the treatment. As shown in Fig. 2d, the effect of ω-conotoxin MVIIA was not related to the loss of mechanical sensitivity by the toxin.
a Time course effects of the intrathecal administration of Phα1β (200 pmol/site) on CCI-induced mechanical allodynia in rats. b Time course effects of Phα1β (200 pmol/site) administration on mechanical sensitivity in rats without CCI. c Time course effects of the intrathecal administration of ω-conotoxin MVIIA (100 pmol/site) on CCI-induced mechanical allodynia in rats. d Time course effects of ω-conotoxin MVIIA (100 pmol/site) administration on mechanical sensitivity in rats without CCI. The results are given as the mean ± SEM. N = 4–14,*P < 0.05 and **P < 0.01 versus PBS. The data were analyzed using two-way ANOVA followed by the Bonferroni test. The effects of the toxins are expressed as 50 % PWT. B baseline value
Effect of Phα1β and ω-conotoxin MVIIA on Ca2+ Transients in DRGs Neurons
The intracellular Ca2+ fluorescence of small-capsaicin-responsive neurons in the control group (saline injected animals) and CFA treated animals, respectively (Fig. 3a) increased by 295 ± 27 % and 593 ± 50 %, respectively, upon treatment with capsaicin (500 nM) (Fig. 3a; P < 0.01). However, the increase of capsaicin-induced Ca2+ fluorescence in the DRG neurons of the CCI group (351 ± 26) and the sham group (353 ± 70 %) was similar (Fig. 3b; P > 0.05). Thus, capsaicin-induced increase on Ca2+ transients of DRGs neurons was higher in inflammatory than neuropathic model of pain.
a Capsaicin (Caps)-induced fluorescence in DRG neurons with or without CFA treatment. Cultured DRG neurons stained with Fluo-4 were stimulated with brief pulses (20 s) of capsaicin (500 nM) for the measurements of calcium transients. Only small (<25 μm), capsaicin-responsive neurons were considered for analysis. The points represent the mean fluorescence levels of cells pooled from at least three experiments. b Capsaicin (Caps)-induced fluorescence in DRG neurons exposed or not to CCI. The points represent the mean fluorescence levels of cells pooled from at least three experiments. c Time course of the effects of 200 nM Phα1β (red) and 200 nM MVIIA (green) on capsaicin (Caps)-induced on Ca2+ transients in the DRG neurons of rats subjected to inflammation through CFA treatment. The DRG neurons were incubated with the toxins for 10 min and subsequently stimulated with capsaicin 500 nM. The points represent the mean fluorescence levels of cells pooled from at least three experiments. d Time course of the effects of 200 nM Phα1β (red) and 200 nM MVIIA (green) on capsaicin (Caps)-induced on Ca2+ transients in the DRG neurons of rats subjected to CCI-induced neurophatic pain. The DRG neurons were incubated with the toxins for 10 min and subsequently stimulated with capsaicin 500 nM. The points represent the mean fluorescence levels of cells pooled from at least three experiments. e The quantification of capsaicin-induced peak fluorescence in the DRG neurons from saline and CFA groups in the presence of Phα1β and MVIIA. The values are normalized to the amplitude of the capsaicin stimulus without toxins (control). f The quantification of capsaicin-induced peak fluorescence in the DRG neurons from sham and CCI groups in the presence of Phα1β and MVIIA. The values are normalized to the amplitude of the capsaicin stimulus without toxins (control). *P < 0.05 compared with the respective control and # P < 0.05 compared with Phα1β administration with saline-treated animals. The data were analyzed using Mann–Whitney test (Color figure online)
Figure 3c shows that the inhibitory effect of Phα1β (200 nM) caused a higher inhibitory effect on Ca2+ transients in DRG neurons of CFA-treated rats than ω-conotoxin MVIIA (200 nM). However, the inhibitory effect of ω-conotoxin MVIIA was higher in neurons of CCl-treated rats. The quantification of the effects of the toxins is shown in Fig. 3e, f. Treatment with Phα1β (200 nM) and ω-conotoxin MVIIA (200 nM) diminished capsaicin-induced amplitude of Ca2+ transients in DRG neurons of the saline group by 43 ± 9 % and 62 ± 10 %, respectively (Fig. 3e, P < 0.05) with no statistically significant difference between the inhibitory effect of the toxins. Figure 3e shows that the inhibitory effect of Phα1β on Ca2+ transients increased to 71 ± 4 % (P < 0.05) in DRG neurons obtained from CFA group whereas the inhibitory effect of ω-conotoxin MVIIA was similar to the saline group (56 ± 15 %; P > 0.05). Thus, CFA treatment increased the inhibitory effect of Phα1β on capsaicin-induced Ca2+ transients in DRGs neurons.
Treatment with Phα1β (200 nM) and ω-conotoxin MVIIA (200 nM) reduced capsaicin-induced Ca2+ transients in DRG neurons of sham rats by 50 ± 10 % and 57 ± 7 %, respectively (Fig. 3f, P < 0.05). However, treatments with Phα1β (200 nM) and ω-conotoxin MVIIA (200 nM) inhibited capsaicin-induced Ca2+ transients in DRG neurons of CCl operated rats by 43 ± 12 % and 71 ± 9 %, respectively (Fig. 3f; P < 0.05) with no significant difference between the inhibition induced by both toxins on Ca2+ transients in the DRG neurons of sham and CCl operated rats (P > 0.05).
Altogether, these data show that the administration of Phα1β and ω-conotoxin MVIIA inhibits the capsaicin-induced Ca2+ responses in DRGs cells, and the effect of Phα1β was higher in the cells from animals subjected to CFA-induced inflammation than in those subjected CCI-induced neuropathic pain.
Discussion
The antinociception potential of VSCCs blockers has been the subject of several investigations. However, the role of Cav2.2 channels in acute and chronic pain is still not fully established (Saegusa et al. 2001; Altier and Zamponi 2004; Snutch 2005). The results of the present study demonstrate the analgesic action of intrathecal injections of Phα1β and ω-conotoxin MVIIA, a N-type VSCCs blockers, in models of inflammatory and neuropathic pain. Phα1β toxin produced a long-lasting and reversible inhibition of mechanical hyperalgesia. Moreover, both toxins alleviated hyperalgesia from a chronic neuropathic and inflammatory state without changing natural mechanical sensitivity in rats.
We have previously demonstrated that intrathecal administration of Phα1β was effective to prevent and to reverse a previously induced persistent chemical and neuropathic pain with lower side effects than treatment with ω-conotoxin MVIIA (Souza et al. 2008). The analgesic action of this toxin is associated with the inhibition of the Ca2+-evoked release of glutamate, a pro-nociceptive neurotransmitter, from rat spinal cord synaptosomes. In addition, the toxin also decreased glutamate overflow in cerebrospinal fluid (Souza et al. 2008). Interestingly, Phα1β blocked the evoked release of glutamate with potency that was approximately 3 times higher than ω-conotoxin MVIIA. Recently, we have shown that intrathecal preemptive administration of Phα1β in mice induced longer antiallodynic effect than ω-conotoxin MVIIA and morphine. Treatment with Phα1β also induced a longer mechanical antiallodynic effect than ω-conotoxin MVIIA and morphine when used after the surgical incision (Souza et al. 2011).
Therapeutic options for chronic pain are only partially effective and produce serious side effects even with the use of analgesic doses (Penn and Paice 2000). In fact, opioids have several adverse effects (constipation; nausea and vomiting; sedation; impaired judgment; and respiratory depression) that can limit their use (McQuay 1997). Although the use of these agents in neuropathic pain is nowadays more accepted than in the past, there are some differences between the different opioids regarding their efficacy against neuropathic pain (Pergolizzi et al. 2008). In addition, the level of clinical evidence for the use of opioids for neuropathic pain is weaker than for cancer pain (Pergolizzi et al. 2008). Therefore, new alternatives for the treatment of inflammatory and neuropathic persistent pain, such as Ca2+ channel blockers, have increased significantly in the recent years (Scott et al. 2002; Winquist et al. 2005; Valía-Vera et al. 2007). The CFA-induced chronic inflammatory pain in rodents has been used as an arthritic pain model (Hogan 2002; Nagakura et al. 2003).
In the present study, we showed that the administration of Phα1β and ω-conotoxin MVIIA in the lumbar subarachnoid space induced potent and long-lasting anti-hyperalgesic effects in inflammatory and neuropathic pain. The toxin Phα1β showed a longer lasting antinociceptive action during pain than that obtained with ω-conotoxin MVIIA. The former caused an anti-hyperalgesic effect that persisted over 24 h, while the effect of the latter only lasted for 6 h. ω-conotoxin MVIIA and Phα1β did not interfere with the excitability of the peripheral nerve terminals since they did not altered the normal mechanical sensitivity of control and exposed animals to inflammatory pain.
The sensory nerves implicated in the development of neurogenic inflammation and inflammatory arthritis have cell bodies located in the DRG. They have central afferent projections to the spinal cord dorsal horn and periphery projections to the joint capsule that generate inflammatory hyperalgesic responses (Staton et al. 2007). The inflammation-induced changes in the excitability and neurotransmitter release are associated with an increase in Ca2+ transients in DRG neurons consistent with previous observations (Lu and Gold 2008). The inhibitory effect of Phα1β on capsaicin-induced Ca2+ responses in DRG neurons is comparable with the inhibitory effect observed with MVIIA. However, the inhibition observed with Phα1β was higher in the DRG neurons of rats during CFA-induced inflammatory pain, suggesting that Phα1β has a special feature for inhibiting signaling in DRG neurons sensitized by the inflammatory process.
We observed that the intrathecal injections of Phα1β or ω-conotoxin MVIIA reversed nerve injury-induced mechanical hyperalgesia. However, little is known about how the CCI model of nerve injury modulates capsaicin-elicited Ca2+ responses in DRG neurons. The pathogenic mechanisms generating pain following nerve injury are complex. Painful neuropathy decreased Ca2+ flux into injured sensory neurons producing decreased excitability and a compensatory response increasing excitability leading to neuropathic pain (Hogan et al. 2000; McCallum et al. 2006). Here, we observed no significant difference in the capsaicin-induced Ca2+ increase in DRG neurons of CCI and sham-operated rats. A similar result was obtained using menthol, a TRPM8 agonist, instead of capsaicin, in the ipsilateral or contralateral DRG neurons of rats subjected to injury (Caspani et al. 2009). However, capsaicin induced a significant increase in the amplitude of Ca2+ responses in DRG neurons of rats treated with CFA that was inhibited using Phα1β or ω-conotoxin MVIIA. In fact, the intraplantar injection of CFA leads to the mechanical and thermal hyperalgesia associated with an increase of the levels of TRPV1 in DRG neurons of treated rats (Yu et al. 2008). Moreover, electrophysiological experiments showed that capsaicin induces greater inward currents in the DRG neurons of CFA-treated rats (Lu et al. 2010). KCl-induced Ca2+ responses in the DRG neurons of CFA-treated rats are also significantly up regulated (Lu et al. 2010). Although the increased levels of TRPV1 could explain the greater Ca2+ response in the DRGs of CFA-treated rats, it is noted that there is a decrease of voltage-gated Ca2+ currents with no change in the expression of α1B with a significant increase in the expression of α2δ (Lu et al. 2010).
The management of chronic inflammatory and neuropathic pain is still a major challenge to clinicians due to their unresponsiveness to the most currently used analgesic drugs. There is evidence suggesting that intrathecal administration of ω-conotoxin-GVIA is not directly implicated in most acute thermal pain sensation (e.g., the hot plate and tail flick assays in rats) but rather play a more significant role in inflammatory and chronic neuropathic conditions (Vanegas and Schaible 2000). Despite the side effects and the narrow therapeutic window, ziconotide, a synthetic form of the ω-conotoxin peptide (Wallace 2006) showed efficacy in both neuropathic and non-neuropathic pain (Schmidtko et al. 2010). In conclusion, the antinociceptive effect of Phα1β, as observed in the present study, suggests that this toxin can represent a novel therapeutic agent for the management of inflammatory and neuropathic pain.
References
Altier C, Zamponi GW (2004) Targeting Ca2+ channels to treat pain: T-type versus N-type. Trends Pharmacol Sci 25:465–470
Bennett GJ, Xie YK (1988) A peripheral mononeuropathy in rat produces disorders of pain sensation like those seen in man. Pain 33:87–107
Berridge MJ, Lipp P, Bootman MD (2000) The versatility and universality of calcium signaling. Nat Rev Mol Cell Biol 1:11–21
Bowersox SS, Gadbois T, Singh T, Pettus M, Wang YX, Luther RR (1996) Selective N-type neuronal voltage-sensitive calcium channel blocker, SNX-111, produces spinal antinociception in rat models of acute, persistent and neuropathic pain. J Pharmacol Exp Ther 279:1243–1249
Caspani O, Zurborg S, Labuz D, Heppenstall PA (2009) The contribution of TRPM8 and TRPA1 channels to cold allodynia and neurophatic pain. PLoS ONE 4:e7383
Chaplan SR, Bach FW, Pogrel JW, Chung JM, Yaksh TL (1994) Quantitative assessment of tactile allodynia in the rat paw. J Neurosci Methods 53:55–63
Cizkova D et al (2002) Localization of N-type Ca2+ channels in the rat spinal cord following chronic constrictive nerve injury. Exp Brain Res 147:456–463
Cordeiro Mdo N et al (1993) Purification and amino acid sequences of six Tx3 type neurotoxins from the venom of the Brazilian ‘armed’ spider Phoneutria nigriventer. Toxicon 31:35–42
Dixon WJ (1980) Efficient analysis of experimental observations. Annu Rev Pharmacol Toxicol 20:441–462
Eun SY, Jung SJ, Park YK, Kwak J, Kim SJ, Kim J (2001) Effects of capsaicin on Ca2+ release from the intracellular Ca2+ stores in the dorsal root ganglion cells of adult rats. Biochem Biophys Res Commun 285:1114–1120
Gomes DA et al (2004) Effect of halothane on the release of [Ca2+]i in dorsal root ganglion neurons. NeuroReport 15:1187–1190
Gribkoff VK, Winquist RJ (2005) Modulators of peripheral voltage-gated sodium channels for the treatment of neuropathic pain. Expert Opin Ther Patents 15:1751–1762
Hogan Q (2002) Animal pain models. Reg Anesth Pain Med 27:385–401
Hogan QH et al (2000) Painful neuropathy decreases membrane calcium current in mammalian primary afferent neurons. Pain 86:43–53
Julius D, Basbaum AI (2001) Molecular mechanisms of nociception. Nature 413:203–210
Katherine E, Galluzzi DO (2007) Managing neuropathic pain. J Am Osteopath Assoc 107:ES39–ES48
Lee Y, Lee CH, Oh U (2005) Painful channels in sensory neurons. Mol Cells 20:315–324
Lu SG, Gold MS (2008) Inflammation-induced increase in evoked calcium transients in subpopulations of rat dorsal root ganglion neurons. Neuroscience 153:279–288
Lu SG, Zhang XL, Luo ZD, Gold MS (2010) Persistent inflammation alters the density and distribution of voltage-activated calcium channels in subpopulations of rat cutaneous DRG neurons. Pain 151:633–643
Malmberg AB, Yaksh TL (1995) Effect of continuous intrathecal infusion of ω-conopeptides, N-type calcium-channel blockers, on behavior and antinociception in the formalin and hot-plate tests in rats. Pain 60:83–90
McCallum JB, Kwok WM, Sapunar D, Fuchs A, Hogan QH (2006) Painful peripheral nerve injury decreases calcium current in axotomized sensory neurons. Anesthesiology 105:160–168
McQuay HJ (1997) Opioid use in chronic pain. Acta Anaesthesiol Scand 41:175–183
Mestre C, Pelissier T, Fialip J, Wilcox G, Eschalier A (1994) A method to perform direct transcutaneous intrathecal injection in rats. J Pharmacol Toxicol Methods 32:197–200
Miljanich GP, Ramachandran J (1995) Antagonists of neuronal calcium channel: structure, function, and therapeutic implications. Annu Rev Pharmacol Toxicol 35:707–734
Nagakura Y et al (2003) Allodynia and hyperalgesia in adjuvant-induced arthritic rats: time course of progression and efficacy of analgesics. J Pharmacol Exp Ther 306:490–497
Penn RD, Paice JA (2000) Adverse effects associated with the intrathecal administration of ziconotide. Pain 85:291–296
Pergolizzi J et al (2008) Opioids and the management of chronic severe pain in the elderly: consensus statement of an International Expert Panel with focus on the six clinically most often used World Health Organization Step III opioids (buprenorphine, fentanyl, hydromorphone, methadone, morphine, oxycodone). Pain Pract 8:287–313
Phillips K, Clauw DJ (2011) Central pain mechanisms in chronic pain states—maybe it is all in their head. Best Pract Res Clin Rheumatol 25:141–154
Saegusa H et al (2001) Suppression of inflammatory and neuropathic pain symptoms in mice lacking the N-type Ca2+ channel. EMBO J 20:2349–2356
Schmidtko A, Lötsch J, Freynhagen R, Geisslinger G (2010) Ziconotide for treatment of severe chronic pain. Lancet 375:1569–1577
Scott DA, Wright CE, Angus JA (2002) Actions of intrathecal ω-conotoxins CVID, GVIA, MVIIA, and morphine in acute and neuropathic pain in the rat. Eur J Pharmacol 451:279–286
Snutch TP (2005) Targeting chronic and neuropathic pain: the N-type calcium channel comes of age. NeuroRx 2:662–670
Souza AH et al (2008) Analgesic effect in rodents of native and recombinant Ph alpha 1beta toxin, a high-voltage-activated calcium channel blocker isolated from armed spider venom. Pain 140:115–126
Souza AH et al (2011) Antiallodynic effect and side effects of Phα1β a neurotoxin from the spider Phoneutria nigriventer: comparison with ω-conotoxin MVIIA and morphine. Toxicon 58:626–633
Staton PC, Wilson AW, Bountra C, Chessell IP, Day NC (2007) Changes in dorsal root ganglion CGRP expression in a chronic inflammatory model of the rat knee joint: differential modulation by rofecoxib and paracetamol. Eur J Pain 11:283–289
Valía-Vera JC, Villanueva VL, Asensio-Samper JM, López-Alarcón MD, de Andrés JA (2007) Ziconotide: an innovative alternative for intense chronic neuropathic pain. Rev Neurol 45:665–669
Vanegas H, Schaible H (2000) Effects of antagonists to high-threshold calcium channels upon spinal mechanisms of pain, hyperalgesia and allodynia. Pain 85:9–18
Vieira LB et al (2005) Inhibition of high voltage-activated calcium channels by spider toxin PnT3–6. J Pharmacol Exp Ther 314:1370–1377
Wallace MS (2006) Ziconotide: a new nonopioid intrathecal analgesic for the treatment of pain. Expert Rev Neurother 6:1423–1428
Wilson AW et al (2006) An animal model of chronic inflammatory pain: pharmacological and temporal differentiation from acute models. Eur J Pain 10:537–549
Winquist RJ, Pan JQ, Gribkoff VK (2005) Use-dependent blockade of Cav2.2 voltage-gated calcium channels for neuropathic pain. Biochem Pharmacol 70:489–499
Yu L et al (2008) The role of TRPV1 in different subtypes of dorsal root ganglion neurons in rat chronic inflammatory nociception induced by complete Freund’s adjuvant. Mol Pain 4:61
Zimmermann M (1983) Ethical guidelines for investigations of experimental pain in conscious animals. Pain 16:109–110
Acknowledgments
Supported by INCT Medicina Molecular, MCT/CNPq, Capes, Pronex and Fapemig. The authors A. H. S., J. F., M. N. C., L. B. V., M. R., R. S. G., and M. V. G. declare they have deposited a patent covering the use of Phα1β for pain management. A. H. S. and C. J. C. are post doctors fellows from Capes.
Author information
Authors and Affiliations
Corresponding author
Rights and permissions
About this article
Cite this article
de Souza, A.H., Castro, C.J., Rigo, F.K. et al. An Evaluation of the Antinociceptive Effects of Phα1β, a Neurotoxin from the Spider Phoneutria nigriventer, and ω-Conotoxin MVIIA, a Cone Snail Conus magus Toxin, in Rat Model of Inflammatory and Neuropathic Pain. Cell Mol Neurobiol 33, 59–67 (2013). https://doi.org/10.1007/s10571-012-9871-x
Received:
Accepted:
Published:
Issue Date:
DOI: https://doi.org/10.1007/s10571-012-9871-x