Abstract
The activity and stability of a β-glycosidase (Thermus thermophilus) and two α-galactosidases (Thermotoga maritima and Bacillus stearothermophilus) were studied in different hydrophilic ionic liquid (IL)/water ratios. For the ILs used, the glycosidases showed the best stability and activity in 1,3-dimethylimidazolium methyl sulfate [MMIM][MeSO4] and 1,2,3-trimethylimidazolium methyl sulfate [TMIM][MeSO4]. A close correlation was observed between the thermostability of the enzymes and their stability in IL media. At high IL concentration (80%), a time-dependent irreversible denaturing effect was observed on glycosidases while, at lower concentration (<30%), a reversible inactivation affecting mainly the k cat was obtained. The results demonstrate that highly thermostable glycosidases are more suitable for biocatalytic reactions in water-miscible ILs.
Similar content being viewed by others
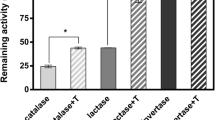
Avoid common mistakes on your manuscript.
Introduction
Due to the well-recognized biological role of oligosaccharides, there is still a great need for green and low cost methods for their preparation despite the considerable development of efficient synthetic methods (Muthana et al. 2009). In the last decade, enzyme-catalyzed reactions have proved to be very efficient for building the glycosidic bond due to their stereoselectivity (Hanson et al. 2004). For this purpose, glycoside hydrolases have been particularly investigated but their use is limited by the competition between hydrolysis and transglycosylation reactions which are both catalyzed by these enzymes. To overcome this limitation, several protein engineering strategies, based on site directed mutagenesis (Mackensie et al. 1998) or molecular directed evolution (Feng et al. 2005), have been proposed to reduce and even eliminate the hydrolytic activities of glycosidases while keeping the transglycosylation activities. Other alternatives suggested to prevent the undesirable hydrolysis reaction were reducing the water activity by using organic solvents (van Rantwijk et al. 1999) or using frozen buffer (ChiffoleauGiraud et al. 1997). However, most of the water-immiscible solvents do not dissolve carbohydrate substrates while water-miscible solvents, such as DMSO and DMF, although dissolving carbohydrates, generally inactivate glycosidases at concentrations above 20%.
Recently, ionic liquids (ILs) have received attention as a promising new class of solvent for chemo- and bio-catalytic organic synthesis (Sheldon et al. 2002). Due to their ability to dissolve polar substrates such as amino-acids or carbohydrates in a low-water environment, several enzymes, mainly esterases, lipases and proteases, have been assayed in ILs and shown to retain biocatalytic activity even at low-water activity (Van Rantwijk and Sheldon 2007). However, a limited number of studies has been devoted to the use of ILs as solvents for reactions catalyzed by glycosidases (Kaftzik et al. 2002; Kamiya et al. 2008; Lang et al. 2006), even though ILs present interesting properties such as their capacity to solubilize both carbohydrates and enzymes.
The objective of this work was to study the stability and the activity of several glycosidases at different IL/water ratios. Three retaining glycosidases, which differ in thermostability, were chosen for this study: α-galactosidase from Bacillus stearothermophilus (AgaB), α-galactosidase from Thermotoga maritima (TmGalA), and β-glycosidase from Thermus thermophilus (Ttβgly).
Materials and methods
Materials
p-Nitrophenyl α- and β-galactoside (pNPαgal and pNPβgal, respectively), 1,3-dimethylimidazolium methyl sulfate [(MMIM)(MeSO4)] and 1,2,3-trimethylimidazolium methyl sulfate [(TMIM)(MeSO4)] were purchased from Sigma-Aldrich and used without any further purification, as NMR spectra of these compounds did not present any detectable contaminant.
Glycosidase expression and purification
The α-galactosidase (TmGalA) gene cloned into a pET2b vector was kindly provided by Robert M. Kelly from the University of North Carolina (Raleigh, USA). This vector was used to transform E. coli BL-21(DE3). Expression and purification of the TmGalA enzyme were performed as previously described (Comfort et al. 2007). Expression of the Ttβgly gene was carried out in the E. coli BL-21(DE3) strain (Novagen) using the vector pET21a (Novagen). Overexpression and purification of the Ttβgly enzyme were conducted as previously described (Feng et al. 2005). The α-galactosidase AgaB was overexpressed in XL1 blue cells and purified according to the procedure described in a previous paper (Dion et al. 2001). Protein concentration and purity were determined using microcapillary electrophoresis (Agilent) with bovine serum albumin as standard.
Enzyme kinetics and activity
For determination of the kinetic parameters at different concentrations of IL, stock solutions of IL/buffer (0–70% v/v) were prepared and the pH was adjusted by adding 1 M HCl. In order to measure the initial activity of the glycosidases using the hydrolysis of pNPαgal and pNPβgal, ε405 was then determined at each pH and for each IL/H2O ratio, since increasing the IL content in a mixed IL/H2O solution strongly affected the extinction coefficient of p-nitrophenol.
Kinetic studies were performed on a microtiter plate reader (iEMS, Labsystem) at 40°C and 405 nm using pNPαgal and pNPβgal as substrates. Kinetic experiments with the α-galactosidase (TmGalA) were carried out in 25 mM citrate/phosphate buffer, 50 mM NaCl, pH 6.5. Kinetic studies with other glycosidases were performed in 50 mM phosphate buffer, pH 7. The kinetic parameters of the glycosidase were estimated by a direct fit of the data to the Michaelis–Menten equation using the Origin 7 program (OriginLab Corp.)
Stability of glycosidases in ILs
The stability of glycosidases in the presence of IL solutions was investigated by incubating the enzymes in an 80% (v/v) IL/buffer solution (pH 6.5, 25 mM citrate/phosphate buffer, 50 mM NaCl for TmGalA and pH 7, 50 mM phosphate buffer for the other glycosidases) at 40°C for different times. Then, the remaining enzymatic activity was determined at regular time intervals after dilution in buffer to give 5% (v/v) IL. Residual activities were determined at 1 mM substrate concentration from initial rates using the standard procedure described above.
Results and discussion
Selection of the ionic liquid
First, as the composition of ILs has a significant impact on enzymatic activity, the hydrolytic activities of the β-glycosidase from T. thermophilus were determined in different water-miscible ILs (Supplementary Table 1) and at different concentrations in buffer (0–33% v/v IL) at pH 7. In agreement with previous studies (Kragl et al. 2002), [MMIM][MeSO4] and [TMIM][MeSO4] ILs were less deleterious to the activity of Ttβgly, as more than 20% of its activity was retained at 33% (v/v) IL concentration (supplementary Fig. 1). Ttβgly showed approximately the same activity profile in [MMIM][MeSO4] and in [TMIM][MeSO4], but the enzyme was more soluble in [MMIM][MeSO4] at high IL concentration. Hence, this IL was selected for further experiments on other glycosidases.
Activity of TmGalA, Ttβgly and AgaB at 40°C as a function of the concentration of [MMIM][MeSO4] (% by vol). The initial activity was measured using: pNPβgal (1 mM) as substrate in 50 mM phosphate buffer, pH 7, for Ttβgly (circle), pNPαgal (1 mM) as substrate in 50 mM phosphate buffer, pH 7, for AgaB (triangle) and pNPαgal (1 mM) in 25 mM citrate/phosphate buffer, pH 6.5, for TmGalA (square)
Relation between thermostability and activity of glycosidases in ILs
The effect of ILs on a specific glycosidase has been reported (Kaftzik et al. 2002; Lang et al. 2006) but not the relationship between enzyme thermostability and its resistance to high concentrations of ILs. Hence, we studied the effect of various concentrations of [MMIM][MeSO4] (0–63% v/v IL) on the activity of glycosidases that differ in their thermostability. Figure 1 represents the residual activity of TmGalA from T. maritima, Ttβgly from T. thermophilus and AgaB from Bacillus stearothermophilus at increasing concentrations of [MMIM][MeSO4]. The temperature stability (time required to lose 50% of activity) of these enzymes is 70 min (Miller et al. 2001), 10 min (Dion et al. 1999) and 2 min at 90°C, respectively. The different behavior of these three enzymes in IL media appears to be closely correlated to their thermostability. TmGalA, which is the most thermostable enzyme, clearly exhibits a greater activity at high IL concentration.
Relation between thermostability and stability of glycosidases in ILs
We also addressed the reversibility of the inactivation at high IL concentration by measuring the residual activity of these enzymes after their incubation at high IL concentration (80% [MMIM][MeSO4]) with increasing time. After the solution was diluted in order to reach 5% (v/v) IL, the residual enzymatic activity was determined using pNPαgal as a substrate (Fig. 2). Full reversibility of the inactivation was obtained for TmGalA even after 2 h of incubation in 80% [MMIM][MeSO4]. For a longer time, a small irreversible denaturation of the enzyme was also observed after 6 h of incubation but 75% of the enzyme remained active. For Ttβgly, the stability in 80% IL was lower since almost 50% of the activity was lost after 30 min. For the least thermostable glycosidase, AgaB, 90% of the enzyme was denatured after only 1 h of incubation in 80% [MMIM][MeSO4].
Residual activity of TmGalA, Ttβgly and AgaB at 40°C as a function of the incubation time in [MMIM][MeSO4] (80% by vol). The residual activity was measured after dilution to 5% [MMIM][MeSO4] using: pNPβgal (1 mM) as substrate in 50 mM phosphate buffer, pH 7, for Ttβgly (circle), pNPαgal (1 mM) as substrate in 50 mM phosphate buffer, pH 7, for AgaB (triangle) and pNPαGal (1 mM) in 25 mM citrate/phosphate buffer, pH 6.5, for TmGalA(square)
Again, a correlation was observed between the thermostability of the enzymes and their stability in the presence of a high concentration of IL. High ion concentration can modify the water structure and hence influence the protein hydration environment. In particular, hydrophilic and water-miscible ILs might remove internally bound water from enzymes and lead to loss of enzyme activity. Thermostable enzymes are generally characterized by a greater rigidity and a more compact structure, which probably results in a stronger resistance to water abstraction by ILs.
Influence of [MMIM][MeSO4] concentrations on TmGalA kinetic parameters
As TmGalA was not irreversibly denatured by a high concentration of IL, this enzyme was chosen to study the effect of increasing IL concentrations on its kinetic parameters. Assuming that no enzyme denaturation occurred during the measurements as demonstrated in Fig. 2, the results presented in Table 1 clearly show that the k cat dramatically decreases with increasing IL concentration, while K m remains almost constant at pH 6.5. Curiously, these kinetic data are compatible with a reversible non-competitive inhibition of the galactosidase by [MMIM][MeSO4] and an apparent inhibition constant K i = 0.35 ± 0.05 M can be derived from them. Such inhibition could be due to the imidazolium cations of [MMIM][MeSO4], according to previous observations showing that substituted imidazole behaves as an inhibitor of various glycosidases (Magdolen and Vasella 2005). However, it is more likely that the decrease in k cat for hydrolytic activity simply reflects the reduced water activity (a w) at high IL concentration. This result shows that the reversible inhibition of glycosidases becomes significant at a high percentage of IL in water, which limits the utility of hydrophilic IL with these enzymes.
Conclusion
Our results indicate a close correlation between the thermostability of the glycosidases and their stability in hydrophilic ILs, suggesting that the more compact structure of the hyperthermostable enzymes prevents ILs from disrupting their protein structure. We have also shown that the decrease in glycosidase activity in ILs is caused by both an irreversible denaturing effect and a direct reversible inhibition of the enzyme activity. However, this decrease in hydrolytic efficiency is not correlated to an increase in transglycosylation activity at high donor and acceptor concentration as expected by the decrease in water activity. Taken together, these results demonstrate that it is preferable to use highly thermostable glycosidases for biocatalytic reactions in water-miscible ILs as green solvents.
References
ChiffoleauGiraud V, Spangenberg P, Rabiller C (1997) Beta-galactosidase transferase activity in ice and use of vinyl-beta-d-galactoside as donor. Tetrahedron Asymmetr 8:2017–2023
Comfort DA, Bobrov KS, Ivanen DR, Shabalin KA, Harris JM, Kulminskaya AA, Brumer H, Kelly RM (2007) Biochemical analysis of Thermotoga maritima GH36 α-galactosidase (TmGalA) confirms the mechanistic commonality of clan GH-D glycoside hydrolases. Biochemistry 46:3319–3330
Dion M, Fourage L, Hallet JN, Colas B (1999) Cloning and expression of a beta-glycosidase gene from Thermus thermophilus. Sequence and biochemical characterization of the encoded enzyme. Glycoconj J 16:27–37
Dion M, Nisole A, Spangenberg P, André C, Glottin-Fleury A, Mattes R, Tellier C, Rabiller C (2001) Modulation of the regioselectivity of a Bacillus α-galactosidase by directed evolution. Glycoconj J 18:215–223
Feng H-Y, Drone J, Hoffmann L, Tran V, Tellier C, Rabiller C, Dion M (2005) Converting a α-glycosidase into a β-transglycosidase by directed evolution. J Biol Chem 280:37088–37097
Hanson S, Best M, Bryan MC, Wong CH (2004) Chemoenzymatic synthesis of oligosaccharides and glycoproteins. Trends Biochem Sci 29:656–663
Kaftzik N, Wasserscheid P, Kragl U (2002) Use of ionic liquids to increase the yield and enzyme stability in the β-galactosidase catalyzed synthesis of N-acetyllactosamine. Org Process Res Dev 6:553–557
Kamiya N, Matsushita Y, Hanaki M, Nakashima K, Narita M, Goto M, Takahashi H (2008) Enzymatic in situ saccharification of cellulose in aqueous-ionic liquid media. Biotechnol Lett 30:1037–1040
Kragl U, Eckstein M, Kaftzik N (2002) Enzyme catalysis in ionic liquids. Curr Opin Biotechnol 13:565–571
Lang M, Kamrat T, Nidetzky B (2006) Influence of ionic liquid cosolvent on transgalactosylation reactions catalyzed by thermostable beta-glycosylhydrolase CelB from Pyrococcus furiosus. Biotechnol Bioeng 95:1093–1100
Mackensie LF, Qingping W, Warren RAJ, Withers SG (1998) Glycosynthases: mutant glycosidases for oligosaccharide synthesis. J Am Chem Soc 120:5583–5584
Magdolen P, Vasella A (2005) Monocyclic, substituted imidazoles as glycosidase inhibitors. Helv Chim Acta 88:2454–2469
Mateo C, Palomo JM, Fuentes M, Betancor L, Grazu V, Lopez-Gallego F, Pessela BCC, Hidalgo A, Fernandez-Lorente G, Fernandez-Lafuente R et al (2006) Glyoxyl agarose: a fully inert and hydrophilic support for immobilization and high stabilization of proteins. Enzyme Microb Tech 39:274–280
Miller ES Jr, Parker KN, Liebl W, Lam D, Callen W, Snead MA, Mathur EJ, Short JM, Kelly RM (2001) α-d-galactosidases from Thermotoga species (hyperthermophilic enzymes, Part A). Methods Enzymol 330:246–260
Muthana S, Cao H, Chen X (2009) Recent progress in chemical and chemoenzymatic synthesis of carbohydrates. Curr Opin Chem Biol 13:573–581
Sheldon RA, Lau RM, Sorgedrager MJ, van Rantwijk F, Seddon KR (2002) Biocatalysis in ionic liquids. Green Chem 4:147–151
Van Rantwijk F, Sheldon RA (2007) Biocatalysis in ionic liquids. Chem Rev 107:2757–2785
Van Rantwijk F, Woudenberg-van Oosterom M, Sheldon RA (1999) Glycosidase-catalysed synthesis of alkyl glycosides. J Mol Catal B Enzym 6:511–532
Acknowledgments
We are grateful to Prof. Robert M. Kelly for the kind gift of the TmGalA gene. This work was supported by a grant from ANR (ANR-07-CP2D-Glycenli) and S.F. was supported by a France/Algeria grant (C.P.F.S.).
Author information
Authors and Affiliations
Corresponding author
Electronic supplementary material
Below is the link to the electronic supplementary material.
Rights and permissions
About this article
Cite this article
Ferdjani, S., Ionita, M., Roy, B. et al. Correlation between thermostability and stability of glycosidases in ionic liquid. Biotechnol Lett 33, 1215–1219 (2011). https://doi.org/10.1007/s10529-011-0560-5
Received:
Accepted:
Published:
Issue Date:
DOI: https://doi.org/10.1007/s10529-011-0560-5