Abstract
Previous mechano-transduction studies have investigated the endothelial cell (EC) morphological response to mechanical stimuli; generally consisting of a wall shear stress (WSS) and a cyclic tensile hoop strain (THS). More recent studies have investigated the EC biochemical response (intercellular adhesion molecule, ICAM-1, and vascular cellular adhesion molecule, VCAM-1, expression) to idealized mechanical stimuli. However, current literature is lacking in the area of EC biochemical response to combinations of physiological WSS and THS mechanical stimuli. The objective of this study is to investigate the EC response to physiological WSS and THS stimuli and to compare this response to that of ECs exposed to idealized steady WSS and cyclic THS of the same magnitudes. This study also investigated the EC response to a nicotine chemical stimulus combined with a suspected athero-prone physiological mechanical stimulus. A bioreactor was designed to apply a range of combinations of physiological WSS and THS waveforms. The bioreactor was calibrated and validated using computational fluid dynamics and video extensometry techniques. The bioreactor was used to investigated the biochemical response exhibited by human umbilical vein endothelial cells (HUVECs) exposed to physiological athero-protective (first bioreactor test case, pulsatile WSS combined with pulsatile THS) and athero-prone (second bioreactor test case, oscillating WSS combined with pulsatile THS) mechanical environments. The final testing environment (third bioreactor test case) combined a nicotine chemical stimulus with the mechanical stimuli of the second bioreactor test case. In first and second bioreactor test cases, the addition of a pulsatile THS to the WSS resulted in opposite trends of ICAM-1 down-regulation and up-regulation, respectively. This outcome suggests that the effect of the additional pulsatile THS depends on the state of the applied WSS waveform. Similarly, in first and second bioreactor test cases, the addition of a pulsatile THS to the WSS resulted in a VCAM-1 up-regulation. However, it has been previously shown that the addition of a cyclic THS to a high- or low-steady WSS resulted in a VCAM-1 down-regulation, indicating that the EC response to idealized mechanical stimuli (steady WSS and cyclic THS) is not comparable to physiological mechanical stimuli (unsteady WSS and pulsatile THS), even though in both situations the average magnitude of WSS and THS applied were similar. In third bioreactor test case, a nicotine chemical stimulus induced a substantial VCAM-1 up-regulation and a moderate ICAM-1 up-regulation. The addition of the mechanical stimuli of the second bioreactors test case resulted in a greater VCAM-1 up-regulation than what was expected, considering the observations of the previous second bioreactor test case alone. This study found that the EC biochemical response to physiological mechanical stimuli is not comparable to the previously observed EC response to idealized mechanical stimuli, even though in both environments the mechanical stimuli were of a similar magnitude. Also, the level of VCAM-1 expressed by the nicotine stimulated ECs showed an elevated level of sensitivity to the athero-prone mechanical stimuli.
Similar content being viewed by others
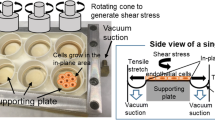
Avoid common mistakes on your manuscript.
Introduction
The human vascular system is continuously exposed to hemodynamic forces, namely wall shear stress (WSS), tensile hoop strain (THS), and pressure. The magnitudes of these forces can vary greatly throughout the arterial system, this can be caused by changes in arterial wall mechanical properties, anatomical location, and arterial geometry. Pathological studies show that arterial disease is frequently found in localized regions that are exposed to very specific hemodynamic WSS.23,28,50
In the majority of previous in vitro mechano-transduction studies, isolated mechanical forces have examined the endothelial cell (EC) response to either WSS6,7,13,21,38 or THS.11,18,20,27,31,34,35,39,40,42,44,46–48 Additional studies have investigated the morphological and cytoskeletal response of ECs to combinations of pulsatile WSS and THS.30,52 These studies have indicated that cell reorientation and alignment and F-actin filament reorganization are a result of a WSS stimulus, and that this cellular response is greatly enhanced with the addition of a THS. The latter study52 alluded to the hypothesis that the addition of the THS might enhance the sensitivity of the ECs toward a WSS stimulus, i.e., a situation where the combination of the WSS and THS resulted in having a synergetic effect on the ECs morphological response. This experimental result tends to imply that a simultaneous mechanical force environment will result in a better understanding of the in vivo function of ECs.
One known EC response to mechanical stimulation is the up- or down-regulation of adhesion molecules. The adhesion molecules ICAM-1 and VCAM-1 when expressed in abnormal quantities have been shown to be associated with the initiation and progression of atherosclerosis.3,10,16,17,29,32,33,36,43 It has been demonstrated that a steady WSS can down regulate the level of EC VCAM-1 expression,3 this down-regulation was shown to be time and shear stress dependent and reversible. Similarly Nagel et al. 33 investigated the response of HUVECs to a range of steady WSS, and showed that ECs expressed a time-dependent, but force-independent ICAM-1 up-regulation in response to the WSS. Additionally, other studies have focused on EC ICAM-1 and VCAM-1 expression in response to a cyclic THS. Cheng et al. 15 and Yun et al. 49 exposed ECs to a cyclic THS for up to 24 h and observed a significant time-dependent ICAM-1 up-regulation. Ali et al. 2 found that a 25% cyclic THS, applied over 6 h, resulted in a 5.5-fold increase in the level of EC ICAM-1 expression.
The effect of combined chemical and mechanical stimulation has received limited coverage in the literature. For example, the EC response to a nicotine chemical stimulus has been investigated previously.1,45 However, the effect on adhesion molecule expression of a combined nicotine and mechanical stimulus has not been observed in the literature to date. Zhang et al. 1 showed that a nicotine chemical stimulus (10−5 M and 10−7 M) resulted in significant increased mRNA levels of endothelial nitric oxide synthase and VCAM-1 after 24 h. Albaugh et al.1 also found that a nicotine stimulus (10−8 M) up-regulated EC VCAM-1 expression after 3 h exposure. Additionally, this study also showed that an ICAM-1 up-regulation occurred, but to a lesser degree. Neither of these studies assessed a simultaneous mechanical and chemical environment, which would more accurately reflect the in vivo situation.
In this study, we propose to investigate the response of ECs to a multiaxial physiological mechanical force environment, in combination with a chemical stimulus. The study used a custom bioreactor which was validated for the application of simultaneous physiological mechanical loading patterns. The specific force patterns applied to the cells relate to physiological conditions found at the carotid sinus, an athero-prone condition similar to the conditions found at the outer wall of the carotid bulb, and an athero-protective condition relating to the force environment at the distal region at the carotid bifurcation. The test environment was designed to test the hypothesis that atherosclerosis initiation (i.e., inappropriate expression of adhesion molecules) could be identified when different simultaneous WSS and THS patterns are applied to ECs. The study also provides information on the compounding effect that two significant risk factors have on the function of ECs, via an atherosclerosis prone mechanical stimulus and a nicotine chemical stimulus.
Experimental Methods and Waveform Validation
Bioreactor Design
The bioreactor was designed to simultaneously apply a range of physiological WSS and THS mechanical stimuli to a variety of tissues and cell types. The bioreactor achieves this goal by combining two previous bioreactor techniques: a cone and plate rheometer6 and multiple flexible silicone substrates upon which the cells are seeded.45 The cone and plate rheometer generates the desired cellular WSS, while the strain applied to the cellular substrates replicates the THS applied to the arterial wall, similar to the situation if an arterial wall was cut longitudinally and rolled out flat and stretched laterally. The WSS and the substrate strain are applied in perpendicular directions, as would normally be the case in the in vivo arterial system. A cone diameter and cone angle of 120 mm and 1.0°, respectively, were used. The tip of the cone was machined flat (20 mm diameter) to prevent rubbing between the cone and plate surface. The cone to plate separation at the center point was 175 μm. Eight square windows were cut from the plate at a mean radial position of 35 mm. Eight corresponding flexible cellular substrates were placed beneath the plate at each of the window locations, as shown in Fig. 1. For each experiment 300 mL of cell media (EGM-2 Cell Media) was used. Both the WSS and THS are separately controlled by two stepper motors, which are individually programed using LabView (version 6.1i). The design, construction, and validation of this system for simple wave forms were previously presented by Breen et al.8
The bioreactor’s rheometer plate (shown as transparent with the cone removed for demonstrational purposes). Eight square windows were cut from the plate and beneath each of the windows is a corresponding flexible cellular substrate. The white arrows indicate the direction of applied WSS, and the black arrows indicate the direction of applied THS. Two further cellular substrate controls were not exposed to a WSS or a THS
Mechanical Stimulation
Physiological WSS Applied
The bioreactor was previously calibrated to generate a range of idealized steady WSS levels.9 However, the human vasculature is exposed to a range of pulsatile and sometimes oscillatory WSS levels. In this study, we implemented two contrasting physiological WSS waveforms, these were determined in a study by Dai et al. 19 using MRI and ultrasound techniques. A pulsatile WSS waveform and an oscillating WSS waveform were chosen to represent the WSS applied to the distal region of the internal carotid artery (ICA) and the internal carotid sinus, respectively, as shown in Fig. 2. These waveforms are considered to be athero-protective and athero-prone.4,14,19,26,28 The two contrasting unsteady WSS waveforms were applied to substrates 1–8, while no WSS was applied to substrates 9 and 10 (non-mechanical stimulated controls), as shown in Table 1.
Pulsatile and oscillating WSS waveforms found in regions of the distal ICA and carotid sinus, respectively. The authors would like to acknowledge Dai et al.19 for the WSS data
In this study, the oscillating shear index (OSI) was calculated to quantify the oscillatory nature of the athero-prone WSS waveform. The definition of the OSI is given in a study by He et al.,24 where a maximum value of OSI (0.5) is indicative of a completely oscillatory flow without any net flow. The calculated OSI for the athero-prone WSS waveform shown in Fig. 2 is 0.49, which is a highly oscillatory waveform.
The Reynolds number for the flow in the cone and plate was calculated for the maximum desired cone angular velocity (250 rpm) at a cellular radial position of 35 mm as 0.2863, with a cone angle of 1° (0.01745 radians), the cell medium dynamic viscosity of 0.003 N s/m2 (3.0 cP) and a density of 1057 kg/m3. The calculated Reynolds number is <1 and is indicative of laminar flow.12
Physiological THS Applied
In a study by Zhao et al.,53 the continuous carotid pulse pressure was measured for five healthy human subjects. These pressure waveforms were noninvasively measured using a high-fidelity external pressure transducer, which was applied to the skin over the common carotid artery. The average of five pulsatile pressure waveforms was used to determine the basic shape of the pulsatile THS waveforms used for this study (see Fig. 3). Three waveforms of varying peak THS magnitudes (12.0%, 8.0%, and 4.0%, shown in Fig. 3c) were applied to different substrates (specifically substrates 1–3 and 5–7), as detailed in Table 1. To simplify labeling of these three THS waveforms, they will be referred to as 0–12%, 0–8%, and 0–4% for the remainder of this paper. It is important to note that no THS was applied to substrates 4, 8, and no WSS or THS was applied to the control samples (substrates 9 and 10), as shown in Table 1.
(a) The pulsatile pressure waveform for the human carotid artery measured in five healthy subjects, (b) the averaged pulsatile pressure waveform, and (c) the three different magnitudes of pulsatile THS waveforms used in the bioreactor tests, where as follows: (i) 0–12% THS, (ii) 0–8% THS, and (iii) 0–4% THS. The authors would like to acknowledge Zhao et al.53 for the pressure waveform data. Image (a) was reproduced with permission from Elsevier
Computational Fluid Dynamic Calibration and Validation for Unsteady WSS
In both the athero-protective and athero-prone WSS waveforms, the Womersley number was calculated to be greater than 1, which indicates that the inertial forces would have a significant influence on the flow.41 Considering the small nonuniformity in the plate geometry and the high Womersley number calculated for the WSS waveforms, it was considered that the best approach to validate the pulsatile and oscillating WSS waveforms would be to use a computational fluid dynamics programme (ANSYS, CFX, www.ansys.com). This validation approach also considered the shallow indents on the plate surface. These shallow indents did affect the flow at the localized edge regions of the shallow indent, but his effect was minimal and had an insignificant effect on the over WSS applied to the cellular samples (see Breen et al. 9 for further details).
The bioreactor was calibrated for a range of steady flow laminar WSS conditions, where a linear relationship between the desired WSS level applied to the cellular substrates and the required cone angular velocity (CAV) to produce this WSS was expressed in terms of a “master curve.” However, in the experimental study reported here, involving unsteady flow, varying WSS waveforms were desired, each requiring a corresponding CAV profile. The linear relationship determined in Breen et al.9 for steady flow (and the resulting master curve) were insufficient to directly determine the appropriate CAV profiles due to the unsteady nature of the flow and the inertia and momentum effects associated with mimicking physiological (mechanical) stimuli. In order to account for momentum/inertia effects, the CFD preprocessor flow state within Ansys-CFX was set to “transient flow.” For transient flow, the CFD model determines the applied cellular WSS for a sequence of time steps (TSs) throughout the wave period. A TS size convergence study was performed and from this study it was determined that 50 and 85 TSs per wave period, for the pulsatile and oscillating WSS waveforms types were necessary to generate sufficiently accurate solutions.
To determine the required input CAV profiles that would generate the two desired WSS waveforms, an initial CAV profile was determined using the steady-state master curve. Then, an iterative process was employed where the proportionality relationship between the WSS and the CAV values was independently adjusted for each TS until the calculated WSS waveform converged to the desired output WSS waveform. The results of this study are shown in Fig. 4 for the pulsatile (athero-protective) WSS waveform and in Fig. 5 for the oscillating (athero-prone) WSS waveform; in both cases, two iterations were sufficient to generate a converged solution.
Finally, the model was run for both cases for a number of wave periods to ensure that there were no significant long-term transient effects, thereby validating the calibration of the required input CAV profiles for repeated cycles of WSS variation.
Experimental Preparation and Setup
Ten cellular substrates were cut from silicone (Goodfellow Cambridge Limited) sheets (100 × 100 × 0.1 mm3); each substrate was cut into 65 × 20 mm2 strips. The substrates were cleaned with 70% ethanol and distilled water in an ultrasonic bath (Bransonic®) for 15 min. The substrates were allowed to air dry in a sterile laminar flow hood. All ten substrates were coated with fibronectin (FN, 6 mg/cm2) to improve the cellular attachment. Human umbilical vein endothelial cells (HUVECs, Cambrex Corp.) were used for the experiments. The cells were cultured using an endothelial growth media (EGM-2, Cambrex Corp) in a humidified incubator (37 °C and 5% CO2). The EGM-2 contained 500 mL of Endothelial Cell Basal Medium-2 and the following growth supplements: hydrocortisone, hFGF-B, VEGF, R3-IGF-1, ascorbic acid, heparin, FBS, hEGF, and GA-1000. The cells were serially passaged using a solution of 0.5% trypsin–0.2% EDTA (Sigma-Aldrich Co.) at a subcultivation ratio of 1:2. The cell media were changed every 2 days during cell culture. The cells used in the experiments were cultured to between passages 6 and 9.
The bioreactor components were washed (detergent: Virkon®, DuPont), cleaned (ethanol), and autoclaved (121 °C for 45 min). The electrical stepper motors were detached from the bioreactor prior to the autoclaving process. The cellular substrates are pretensioned before the cells are seeded to minimize any chance of substrate flexure during testing. A 500 μL aliquot of HUVECs suspended in EGM-2 (150,000 cells/cm2) was placed on each of the ten fibronectin-coated cellular substrates. The cells were seeded on all ten cellular substrates at a concentration of 150,000 cells/cm2 and incubated at 37 °C in a humidified atmosphere of 5% CO2/95% air. After the cells formed a confluent monolayer, the culture medium was changed and the bioreactor was filled with 300 mL of the 2.586% dextran/EGM-2 solution (dynamic viscosity of 3cP). At this stage, the ECs were ready to be subjected to a mechanical and/or chemical stimulation. Two control cellular substrates (9 and 10) were incubated in a separate compartment (with no application of mechanical forces) of the bioreactor. This separate compartment was supplied with the same cell media as the rest of the other test cellular substrates. The control substrates (9 and 10) were not exposed to any mechanical stimulus. The testing environment (the levels of oxygen, carbon dioxide, and humidity) was set and controlled by the incubator within which the bioreactor was placed for the duration of the experiments. The pH of the cell media was measured before the cells were placed in the bioreactor and after the cells were in the bioreactor for 12 h. Less than a 3% change in the pH level was observed over the duration of a 12 h bioreactor test (results not shown).
After each bioreactor test, a section of cellular substrates samples were fixed with paraformaldehyde and the cells nuclei were stained using propidium iodide. These cells were captured and examined using fluorescent microscopy. These images were used to qualitatively assess the HUVECs viability after each bioreactor test, ensuring that a healthy confluent monolayer cells was maintained. See Fig. 6 for a typical image of ECs exposed to a pulsatile WSS and pulsatile THS (0–12%) for 12 h.
After each bioreactor test, the remaining sample cell populations were fixed, stained, and examined for ICAM-1 and VCAM-1 expression using immunostaining and flow cytometry. The ECs were stained using FITC conjugated mouse anti-human ICAM-1 primary antibody (Bender MedSystems, clone number: RR1/1) and FITC conjugated mouse anti-human VCAM-1 primary antibody (Abcam plc, clone number: M/K-2). A population of cells from each substrate was stained with a FITC-conjugated IgG2A second antibody to account for any nonspecific antibody binding (antibody negative control).
The stained cellular samples were analysed with a fluorescence-activated cell sorter (FACS Calibur System, BD Biosciences). The specific mean fluorescence intensity (MFI) was obtained for each cell population after correction for nonspecific binding. The flow cytometry data are acquired in dot plot format, where the appropriate populations of dots are selected and quantified using a dot plot analysis program (Win MDI). The selected data are then presented in histogram distribution plots to quantify the level of MFI of ICAM-1 and VCAM-1 expressed. In this current study, the MFI of ICAM-1 and VCAM-1 expressed was presented as bar diagrams for ease of analysis. The level of ICAM-1 and VCAM-1 expressed was normalized against the basal level of ICAM-1 and VCAM-1 expression in the control samples, where the cells were exposed to no mechanical stimuli (see Breen et al.8 for further details on data analysis protocol).
Results
The individual tests performed using the bioreactor can be described in terms of the three different bioreactor test cases. The first bioreactor test case: a pulsatile WSS combined with three levels of pulsatile THS waveforms (0–12%, 0–8%, and 0–4%), a pulsatile WSS alone and two nonmechanically stimulated controls. The second bioreactor test case: an oscillating WSS combined with three levels of pulsatile THS waveforms (0–12%, 0–8%, and 0–4%), an oscillating WSS alone and two nonmechanically stimulated controls. The third bioreactor test case: the same mechanical stimuli as in second bioreactor test case but with a nicotine chemical stimulus. The third bioreactor test case also had two additional substrates (substrate numbers 11 and 12) that were not mechanically or chemically stimulated. The testing period for all three bioreactor test cases was 12 h.
The levels of biochemical response exhibited by the HUVECs were analysed using statistical analysis software (GraphPad InStat®, version 3.05). This programme was used to perform a one-way analysis of variance (ANOVA), which compares the degree of ICAM-1 and VCAM-1 exhibited by the stimulated and unstimulated HUVECs. When a statistical significance was detected, a post-test analysis was conducted using Tukey test. p-values less than 0.05 were considered statistically significant.
Twelve Hours Pulsatile WSS and THS Bioreactor Test
The first bioreactor test case was repeated three times, providing a sample number (n) of 6 for each unique combination of stimuli, as two cellular samples were exposed to the same mechanical stimulus in each test. The results in terms of ICAM-1 and VCAM-1 expression for the first bioreactor test case are shown in Fig. 7.
ICAM-1 and VCAM-1 expression of HUVECs exposed to a pulsatile WSS and a range of pulsatile THS for 12 h. The mean fluorescence intensify (MFI) was normalized against the static control samples. The applied pulsatile WSS (Puls. WSS) and THS waveforms are shown in Figs. 2 and 3c. The data are represented as mean ± SD. Six samples were measured for each combination of stimulus; *p < 0.05, **p < 0.01, and ***p < 0.001
The pulsatile WSS alone resulted in a significant ICAM-1 up-regulation (35% increase relative to the control, p < 0.05) and VCAM-1 down-regulation (34% decrease relative to the control, p < 0.01), a very similar cellular response to what was previously found in a study where HUVECs were exposed to a high steady WSS alone.8 However, the addition of a pulsatile THS to the pulsatile WSS reduced the initial ICAM-1 up-regulation and VCAM-1 down-regulation, respectively. The greatest reduction of the initial ICAM-1 up-regulation (17% reduction relative to the pulsatile WSS alone response) occurred when the largest pulsatile THS (0–12%) was combined with the pulsatile WSS. This specific combination of mechanical stimuli also resulted in the greatest reduction of the initial VCAM-1 down-regulation, where a 41% reduction in the initial VCAM-1 down-regulation (in response to the pulsatile WSS alone) was observed (see Fig. 7).
Twelve Hours Oscillating WSS and THS Bioreactor Test
The second bioreactor test case was repeated three times, again generating a sample number of six for each unique combination of stimuli. The results in terms of ICAM-1 and VCAM-1 expression for the second bioreactor test case are shown in Fig. 8.
ICAM-1 and VCAM-1 expression of HUVECs exposed to an oscillating WSS and a range of pulsatile THS for 12 h. The MFI was normalized against the static control samples. The applied oscillating WSS (Osc. WSS) and THS waveforms are shown in Figs. 2 and 3c. The data are represented as mean ± SD. Six samples were measured for each combination of stimulus; *p < 0.05, **p < 0.01, and ***p < 0.001
After 12 h of oscillating WSS alone, a significant VCAM-1 up-regulation (23% increase relative to the control, p < 0.01) and an ICAM-1 up-regulation (18% increase relative to the control) was observed (see Fig. 8). This significant VCAM-1 up-regulation correlates with the findings of previous studies,4,14,19,26,28 which suggests that an oscillating WSS up-regulates specific adhesion molecules that are associated with excessive monocyte adhesion and trans-endothelium migration.
The addition of a range of pulsatile THS caused a further trend of up-regulation of both ICAM-1 and VCAM-1. In regards ICAM-1 expression, a combination of the oscillating WSS and the medium pulsatile THS (0–8%) produced the maximum ICAM-1 up-regulation (29% up-regulation relative to the control). Similarly for VCAM-1, a combination of the oscillating WSS and the largest pulsatile THS (0–12%) resulted in the maximum VCAM-1 up-regulation (51% up-regulation relative to the control, p < 0.001), as shown in Fig. 8.
In overall terms, the addition of the pulsatile THS to the pulsatile or oscillating WSS resulted in a general trend of VCAM-1 up-regulation in both the first and second bioreactor test cases (see Figs. 7 and 8). However, the addition of the pulsatile THS to the pulsatile WSS in the first bioreactor test case resulted in a trend of ICAM-1 down-regulation (see Fig. 7), whereas the same addition of the pulsatile THS in second bioreactor test case triggered a trend of ICAM-1 up-regulation (see Fig. 8). On the other hand, a drop in the ICAM-1 up-regulation was recorded when the ECs were exposed to a combination of an oscillating WSS and the largest pulsatile THS (0–12%), as shown in Fig. 8. Note that a similar ICAM-1 down-regulation was observed in a previous study by Breen et al.,8 where HUVECs were exposed to a low-steady WSS (0.4 Pa) combined with a high (0–12%) saw tooth THS waveform.
Twelve Hours of the Athero-Prone Bioreactor Test With a Nicotine Stimulus
A study by Benowitz et al. 5 showed that nicotine in the blood stream increases heart rate and blood pressure, resulting in an in vivo environment consisting of elevated pressures, which are applied at a faster rate. This in vivo environment could likely result in elevated levels of arterial THS magnitudes and rates. This in vivo environment could lead to endothelium damage, resulting in an endothelial dysfunction and a smooth muscle cell (SMC) trans-endothelium proliferation, potentially causing a stenosis, similar to what has been observed following angioplasty and stenting procedures.25 The third bioreactor test case investigated the HUVECs response to one such potentially stenotic or athero-prone chemical and mechanical environment.
The third bioreactor test case investigated the biochemical response exhibited by HUVECs exposed to the same mechanical stimuli as the second bioreactor test case with the addition of a nicotine chemical stimulus (10−5 M). The concentration of nicotine used for these tests is equivalent to the concentration of nicotine that is present in the blood stream of a regular smoker.51 The nicotine was mixed with the common pool of cell medium and this pool of medium was used as the nicotine delivery vehicle for the nicotine stimulated cellular samples (cellular samples 1–10) for the 12 h testing period. However, two further control samples (cellular samples 11–12) were used during this bioreactor test, where these cellular samples were incubated in a separate Petri dish, which was filled with the same cell medium as the other cellular samples but without the nicotine additive. None of the four control samples (samples 9, 10, 11, and 12) were exposed to any mechanical stimuli. This particular bioreactor test was preformed three times, yielding a sample number of six substrates for each unique level of mechanical and/or chemical stimuli. The resulting levels of ICAM-1 and VCAM-1 expressed are shown in Fig. 9.
ICAM-1 and VCAM-1 expression of HUVECs exposed to an oscillating (Osc.) WSS and a range of pulsatile (Pul.) THS with a nicotine (10−5 M) chemical stimulus for 12 h. The data are represented as mean ± SD. Six samples were measured for each combination of stimulus; *p < 0.05 and **p < 0.01 vs. static control. The control and A–E labels were used to identify the specific mechanical and chemical stimuli applied to the various test samples
The 12 h nicotine chemical stimulus (alone) resulted in a significant up-regulation of both ICAM-1 (27% increase, p < 0.05) and VCAM-1 (108% increase, p < 0.01), which agrees with the observations of previous studies by Zhang et al. 51 and Albaugh et al.1 The combination of an oscillating WSS with a nicotine stimulus resulted in a further trend of VCAM-1 (141% increase relative to the nonmechanical and nonchemical stimulated control, p < 0.01) up-regulation. However, the addition of the oscillating WSS, to the nicotine chemical stimulated samples, did not have any significant effect on the level of ICAM-1 expressed (see Fig. 9).
The addition of a pulsatile THS, combined with the oscillating WSS and nicotine stimulus, resulted in a trend of ICAM-1 and VCAM-1 up-regulation. The greatest ICAM-1 (42% up-regulation relative to the no mechanical and no chemical stimulus control, p < 0.01) and VCAM-1 (165% up-regulation relative to the no mechanical and no chemical stimulus control, p < 0.01) up-regulation was observed when the ECs were exposed to a medium pulsatile THS (0–8%) and a high pulsatile THS of (0–12%), respectively. This pattern of ICAM-1 and VCAM-1 response corresponds to what was previously found in the second bioreactor test case, where no nicotine stimulus was included.
Overall, both ICAM-1 and VCAM-1 up-regulation followed the same trends observed in the second bioreactor test case (see Fig. 8), including a decrease in the level of ICAM-1 expression when the ECs were exposed to the largest pulsatile THS (0–12%), as shown in Fig. 9.
Discussion and Conclusions
A novel bioreactor was designed, calibrated, and validated to apply pulsatile or oscillating WSS with/without pulsatile THS waveforms, which are typical of the mechanical environment found at the carotid artery in the in vivo situation. These WSS and THS waveforms were calibrated and validated using computational fluid dynamics and video extensometry techniques. The novelty of this bioreactor is demonstrated in the way that the cellular samples are exposed to simultaneous pulsatile or oscillating WSS combined with a range of pulsatile THS waveforms.
For each bioreactor test, all the cellular substrates were submerged in a common pool of cell media. This situation has the potential to cause a cross migration of biochemical signals which could influence the protein synthesis of neighboring cellular samples. However, Morigi et al.32 have shown that ECs grown in static conditions, with either mechanically preconditioned cell media or new fresh cell media, expressed equivalent levels of ICAM-1 and VCAM-1 expression. This study showed that the secreted biochemical signals in the mechanically preconditioned cell media had a negligible effect on the level of ICAM-1 or VCAM-1 expression. Therefore, based on this, the authors considered it reasonable that any cellular protein synthesis observed after a test with the current bioreactor could be assumed to be exclusively a biochemical response to the mechanical stimulus, and the cellular response could be assumed to be uninfluenced by any biochemical signals that may be secreted into the common pool of cell media.
The HUVECs biochemical response to an isolated cyclic THS was not investigated in this study, but previous studies have investigated the ICAM-1 and VCAM-1 response to a cyclic THS mechanical stimulus.2,15 These studies observed that a cyclic THS resulted in an ICAM-1 and VCAM-1 up-regulation. In this current study, the addition of the pulsatile THS to the pulsatile or oscillating WSS resulted in a trend of VCAM-1 up-regulation for all three bioreactor test cases and a trend of ICAM-1 up-regulation for the second and third bioreactor test cases, excluding the ICAM-1 down-regulation observed when the ECs were exposed to the highest THS (0–12%). However, in the first bioreactor test case the addition of the pulsatile THS to the pulsatile WSS resulted in a trend of ICAM-1 down-regulation (see Fig. 7), which is unexpected considering that it has been shown in previous studies15,49 that an isolated cyclic THS results in an ICAM-1 up-regulation. Furthermore, in the second bioreactor test case an ICAM-1 up-regulation was observed when the ECs were exposed to the same pulsatile THS combined with an oscillating WSS. This unanticipated ICAM-I down-regulation in response to the additional pulsatile THS in the first bioreactor test case would suggest that the pulsatile WSS may have an controlling influence on the ECs response to a pulsatile THS, in terms of ICAM-1 expression.
In this study, the bioreactor was used to apply two different mechanical environments. In first bioreactor test case, an pulsatile WSS mechanical stimulus resulted in a significant ICAM-1 up-regulation (35% increase, p < 0.05) and the addition of a range of pulsatile THS, combined with the pulsatile WSS, resulted in a trend of ICAM-1 down-regulation, as shown in Fig. 7. However, the same addition of a range of pulsatile THS combined with an oscillating WSS in the second bioreactor test case, resulted in a trend of ICAM-1 up-regulation (Fig. 8). This observation would suggest that the addition of a pulsatile THS to two contrasting WSS waveforms may not result in a similar ICAM-1 response. Specifically for the oscillating and pulsatile WSS waveforms used in this study, the addition of the pulsatile THS resulted in opposing patterns of ICAM-1 response. This outcome would suggest that the shape of the WSS waveform applied to HUVECs may have a dominating influence on the ICAM-1 response to the additional pulsatile THS.
In the first and second bioreactor test cases, the addition of a pulsatile THS to both the oscillating and pulsatile WSS waveforms resulted in a VCAM-1 up-regulation, as shown in Figs. 7 and 8. However, it was previously observed that the addition of a cyclic THS to a high (2.5 Pa) or a low (0.4 Pa) steady WSS resulted in a VCAM-1 down-regulation.8 This observation would indicate, at least for VCAM-1 synthesis, that the effect of an idealized mechanical environment (a steady WSS combined with a cyclic THS) is not comparable to a more physiological mechanical environment (an pulsatile or oscillating WSS combined with a pulsatile THS), even though in both situations the average magnitude of WSS and THS applied were similar. The authors would argue that this observation demonstrates the necessity to apply realistic unsteady (pulsatile or oscillating) WSS and pulsatile THS mechanical stimuli when investigating the EC biochemical response to an in vivo physiological environment.
VCAM-1 is known to promote monocyte-endothelium adhesion, as does ICAM-1, but VCAM-1 also promotes monocyte trans-endothelium migration.22 Therefore, it could be claimed that a combination of mechanical stimuli that generates the greatest level of VCAM-1 up-regulation is likely to be the most athero-prone mechanical environment. For the mechanical stimuli considered in this study, the greatest level of VCAM-1 up-regulation occurred when the cells were exposed to an oscillating WSS combined with the highest level of THS (0–12%). This outcome suggests that an arterial region that is exposed to this combination of mechanical stimuli would be most susceptible to atherosclerosis formation. In a finite element analysis study conducted by Zhao et al.,53 it was determined that a similar combination of low WSS and high mechanical stress occur in the sinus region of the ICA, an arterial location that is strongly linked with the focal formation of atherosclerosis.50
When analysing the second bioreactor test case results as a whole, both VCAM-1 and ICAM-1 tended to be up-regulated (see Fig. 8). The greatest level of VCAM-1 up-regulation (51% up-regulation relative to the control, p < 0.001) occurred when the cells were exposed to an oscillating WSS combined with the highest level of pulsatile THS (0–12%). This outcome suggests that an arterial region that is exposed to this combination of mechanical stimuli could be most highly susceptible to atherosclerosis formation. In a finite element analysis study conducted by Zhao et al.,53 it was determined that a similar combination of low WSS and high mechanical stress occur in the sinus region of the ICA, an arterial location that is strongly linked with the focal formation of atherosclerosis.50
In contrast, it could be argued that a combination of mechanical stimuli that generates the greatest level of VCAM-1 down-regulation is likely to be the most athero-protective mechanical environment. In this study, the lowest level of pulsatile THS combined with the pulsatile WSS resulted with the greatest VCAM-1 down-regulation (34% decrease relative to the control, p < 0.01), as shown in Fig. 7. This finding is consistent with the low level of atherogenic lesions found in straight arterial regions, e.g., the distal region of the ICA, which is considered less susceptible to atherosclerosis.19
In this study, the addition of a pulsatile THS to the unsteady WSS waveforms resulted in a trend of VCAM-1 up-regulation. However, previous mechano-transduction studies have shown that the addition of a cyclic THS to a steady WSS resulted in a trend of VCAM-1 down-regulation (see Breen et al. 8). This contradictory result implies that to achieve a better understanding of the in vivo cellular response of ECs to mechanical environments a more realistic mechanical stimulus environment must be used in in vitro experiments. Our observations demonstrate the importance of using physiological mechanical stimuli in future mechano-transduction experiments in order to determine accurate physiological cellular responses.
A 12 h nicotine chemical stimulus, applied in the third bioreactor test case, resulted in an ICAM-1 up-regulation (27%, p < 0.05) and a substantial VCAM-1 up-regulation (108%, p < 0.01), as shown in Fig. 9a. These results agree with the findings of Zhang et al. 1 and Albaugh et al. 1 Such a large up-regulation of both adhesion molecules would clearly indicate that this level of nicotine in the blood stream would be conducive to systemic atherosclerosis.
The addition of an oscillating WSS to the nicotine chemical stimulus caused a further VCAM-1 up-regulation (32% increase, see Fig. 9b), which was greater than expected, considering that an oscillating WSS alone resulted in a 23% VCAM-1 up-regulation (see Fig. 8). A similar situation was also found when the ECs were exposed to a range of pulsatile THS levels (see Figs. 9c–9e). This outcome suggests that the nicotine stimulated ECs were somewhat sensitized to the athero-prone mechanical stimuli and that the addition of the athero-prone mechanical stimuli would have a greater detrimental effect on the ECs than if the ECs were not exposed to a nicotine stimulus at all. This would suggest that the vasculature of a smoker is more likely to develop atherosclerosis for two reasons: firstly, due to the nicotine chemical stimulus that substantially up-regulated the level of VCAM-1 and secondly, due to the heightened sensitivity of the ECs VCAM-1 expression in response to an athero-prone mechanical environment.
The nicotine alone stimulus did result in an ICAM-1 up-regulation, but the addition of an oscillating WSS had a negligible effect on the level of ICAM-1 expression (see Fig. 9b). In fact, an ICAM-1 up-regulation on the order of 18% was expected, considering that an oscillating WSS alone, in the second bioreactor test case, resulted in such an up-regulation, as shown in Fig. 8. This suggests that the nicotine stimulus had an overwhelming influence on the ICAM-1 expression and desensitized the ECs to an oscillating WSS. The application of a range of pulsatile THS did cause a moderate ICAM-1 up-regulation (see Figs. 9c and 9d). However, a moderate decrease in the quantity of ICAM-1 expression occurred when the largest pulsatile THS (0–12%) was applied, this decrease was previously observed in the second bioreactor test case, as shown in Fig. 8. However, in the second bioreactor test case the magnitude of ICAM-1 down-regulation was much greater, which further supports the idea that the nicotine stimulus has a desensitising effect on the level of ICAM-1 expressed by the ECs exposed to mechanical stimuli.
This study investigated the EC biochemical response to mechanical and chemical stimuli using two molecular markers, namely ICAM-1 and VCAM-1. One limitation of the study was that other adhesion molecules were neglected, such as P-selectin, E-selectin, and PECAM-1, which could also have been used to quantify the EC biochemical response to the physiological mechanical stimuli.
In all three bioreactor test cases, the WSS and THS waveforms were applied in sequence with each other, i.e., there was no time lag or shear phase angle (SPA) between the applied WSS waveform and the THS waveform (SPA = 0°). However, it is interesting to note for the in vivo system that this is not always necessarily true and the SPA can vary throughout the human vasculature system.37 Even though a SPA of zero was used for the bioreactor test cases reported here, significant interactions between the WSS and the THS have been demonstrated. However, it has to be acknowledged that to assess the significance of the interactions between the WSS and the THS for other SPAs, further experiments would have to be conducted. A future study investigating the EC biochemical response to mechanical stimuli applied with various SPAs could provide very interesting results, considering that a study conducted Qui et al. 37 showed that ECs secrete different levels of endothelin-1 and nitric oxide when exposed to various SPA levels.
In summary, a bioreactor capable of applying unsteady combinations of pulsatile or oscillating WSS and pulsatile THS waveforms was calibrated, validated, and used to experimentally investigate the biochemical response of ECs to suspected athero-prone and athero-protective mechanical stimuli. This bioreactor is capable of applying a wide range of WSS and THS waveforms, in a controlled environment. However, one limitation of this bioreactor is that it does not allow for independent control of the pressure applied to the cellular samples. Nonetheless, the capability of this bioreactor to simultaneously apply pulsatile or oscillating WSS waveforms combined with pulsatile THS waveforms to multiple cellular samples gives this bioreactor an advantage over other bioreactors that can only apply isolated mechanical stimuli.
References
Albaugh, G., et al. Nicotine induces mononuclear leukocyte adhesion and expression of adhesion molecules, VCAM and ICAM, in endothelial cells in vitro. Ann. Vasc. Surg. 18(3):302–307, 2004.
Ali, M. H., et al. Mitochondrial requirement for endothelial responses to cyclic strain: implications for mechanotransduction. Am. J. Physiol. Lung Cell Mol. Physiol. 287(3):L486–L496, 2004.
Ando, J., et al. Shear stress inhibits adhesion of cultured mouse endothelial cells to lymphocytes by downregulating VCAM-1 expression. Am. J. Physiol. 267(3 Pt 1):C679–C687, 1994.
Barakat, A., and D. Lieu. Differential responsiveness of vascular endothelial cells to different types of fluid mechanical shear stress. Cell Biochem. Biophys. 38(3):323–343, 2003.
Benowitz, N. L., et al. Interindividual variability in the metabolism and cardiovascular effects of nicotine in man. J. Pharmacol. Exp. Ther. 221(2):368–372, 1982.
Blackman, B. R., K. A. Barbee, and L. E. Thibault. In vitro cell shearing device to investigate the dynamic response of cells in a controlled hydrodynamic environment. Ann. Biomed. Eng. 28:363–372, 2000.
Blackman, B. R., G. Garcia-Cardena, and M. A. Gimbrone, Jr. A new in vitro model to evaluate differential responses of endothelial cells to simulated arterial shear stress waveforms. J. Biomech. Eng. 124(4):397–407, 2002.
Breen, L., P. E. McHugh, B. P. Murphy, et al. Multi-axial mechanical stimulation of HUVECs demonstrates that combined loading is not equivalent to the superposition of individual wall shear stress and tensile hoop stress components. J. Biomech. Eng. 131(8):081001, 2009.
Breen, L., et al. Development of a novel bioreactor to apply shear stress and tensile strain simultaneously to cell monolayers. Rev. Sci. Instrum. 77:104301, 2006.
Brooks, A. R., P. I. Lelkes, and G. M. Rubanyi. Gene expression profiling of human aortic endothelial cells exposed to disturbed flow and steady laminar flow. Physiol. Genom. 9(1):27–41, 2002.
Buck, R. C. Reorientation response of cells to repeated stretch and recoil of the substratum. Exp. Cell Res. 127(2):470–474, 1980.
Buschmann, M. H., et al. Analysis of flow in a cone-and-plate apparatus with respect to spatial and temporal effects on endothelial cells. Biotechnol. Bioeng. 89:493–502, 2002.
Bussolari, S. R., C. F. Dewey, Jr., and M. A. Gimbrone, Jr. Apparatus for subjecting living cells to fluid shear stress. Rev. Sci. Instrum. 53(12):1851–1854, 1982.
Chappell, D. C., et al. Oscillatory shear stress stimulates adhesion molecule expression in cultured human endothelium. Circ. Res. 82(5):532–539, 1998.
Cheng, J. J., et al. Cyclic strain enhances adhesion of monocytes to endothelial cells by increasing intercellular adhesion molecule-1 expression. Hypertension 28(3):386–391, 1996.
Chiu, J. J., et al. Shear stress inhibits adhesion molecule expression in vascular endothelial cells induced by coculture with smooth muscle cells. Blood 101(7):2667–2674, 2003.
Chiu, J. J., et al. Shear stress increases ICAM-1 and decreases VCAM-1 and E-selectin expressions induced by tumor necrosis factor-[alpha] in endothelial cells. Arterioscler. Thromb. Vasc. Biol. 24(1):73–79, 2004.
Clark, C. B., T. J. Burkholder, and J. A. Frangos. Uniaxial strain system to investigate strain rate regulation in vitro. Rev. Sci. Instrum. 72:2415–2422, 2001.
Dai, G., et al. Distinct endothelial phenotypes evoked by arterial waveforms derived from atherosclerosis-susceptible and -resistant regions of human vasculature. Proc. Natl. Acad. Sci. USA 101(41):14871–14876, 2004.
Dartsch, P. C., and E. Betz. Response of cultured endothelial cells to mechanical stimulation. Basic Res. Cardiol. 84(3):268–281, 1989.
Dewey, Jr., C. F., et al. The dynamic response of vascular endothelial cells to fluid shear stress. J. Biomech. Eng. 103(3):177–185, 1981.
Frijns, C. J., and L. J. Kappelle. Inflammatory cell adhesion molecules in ischemic cerebrovascular disease. Stroke 33(8):2115–2122, 2002.
Gnasso, A., et al. In vivo association between low wall shear stress and plaque in subjects with asymmetrical carotid atherosclerosis. Stroke 28(5):993–998, 1997.
He, X., and D. N. Ku. Pulsatile flow in the human left coronary artery bifurcation: average conditions. J. Biomech. Eng. 118(1):74–82, 1996.
Hoffmann, R., et al. Patterns and Mechanisms of In-Stent Restenosis: A Serial Intravascular Ultrasound Study. Circulation 94(6):1247–1254, 1996.
Hsiai, T. K., et al. Monocyte recruitment to endothelial cells in response to oscillatory shear stress. Faseb. J. 17(12):1648–1657, 2003.
Kanda, K., and T. Matsuda. Behavior of arterial wall cells cultured on periodically stretched substrates. Cell Transpl. 2(6):475–484, 1993.
Malek, A. M., S. L. Alper, and S. Izumo. Hemodynamic shear stress and its role in atherosclerosis. JAMA 282(21):2035–2042, 1999.
Mohan, S., et al. Regulation of low shear flow-induced HAEC VCAM-1 expression and monocyte adhesion. Am. J. Physiol. 276(5 Pt 1):C1100–C1107, 1999.
Moore, Jr., J. E., et al. A device for subjecting vascular endothelial cells to both fluid shear stress and circumferential cyclic stretch. Ann. Biomed. Eng. 22(4):416–422, 1994.
Moretti, M., et al. Endothelial cell alignment on cyclically-stretched silicone surfaces. J. Mater. Sci. Mater. Med. 15(10):1159–1164, 2004.
Morigi, M., et al. Fluid shear stress modulates surface expression of adhesion molecules by endothelial cells. Blood 85(7):1696–1703, 1995.
Nagel, T., et al. Shear stress selectively upregulates intercellular adhesion molecule-1 expression in cultured human vascular endothelial cells. J. Clin. Invest. 94(2):885–891, 1994.
Naruse, K., T. Yamada, and M. Sokabe. Involvement of SA channels in orienting response of cultured endothelial cells to cyclic stretch. Am. J. Physiol. Heart Circ. 274:H1532–H1538, 1998.
Neidlinger-Wilke, C., et al. Cell alignment is induced by cyclic changes in cell length: studies of cells grown in cyclically stretched substrates. J. Orthop. Res. 19(2):286–293, 2001.
Ohtsuka, A., et al. The effect of flow on the expression of vascular adhesion molecule-1 by cultured mouse endothelial cells. Biochem. Biophys. Res. Commun. 193(1):303–310, 1993.
Qiu, Y., and J. M. Tarbell. Interaction between wall shear stress and circumferential strain affects endothelial cell biochemical production. J. Vasc. Res. 37(3):147–157, 2000.
Sato, M., N. Ohshima, and R. M. Nerem. Viscoelastic properties of cultured porcine aortic endothelial cells exposed to shear stress. J. Biomech. 29(4):461–467, 1996.
Shirinsky, V. P., et al. Mechano-chemical control of human endothelium orientation and size. J. Cell Biol. 109(1):331–339, 1989.
Sipkema, P., et al. Effect of cyclic axial stretch of rat arteries on endothelial cytoskeletal morphology and vascular reactivity. J. Biomech. 36(5):653–659, 2003.
Smith, B. W., et al. Velocity profile method for time varying resistance in minimal cardiovascular system models. Phys. Med. Biol. 48(20):3375–3387, 2003.
Takemasa, T., et al. Oblique alignment of stress fibers in cells reduces the mechanical stress in cyclically deforming fields. Eur. J. Cell Biol. 77(2):91–99, 1998.
Tsao, P. S., et al. Fluid flow inhibits endothelial adhesiveness. Nitric oxide and transcriptional regulation of VCAM-1. Circulation 94(7):1682–1689, 1996.
Wang, J. H., P. Goldschmidt-Clermont, and F. C. Yin. Contractility affects stress fiber remodeling and reorientation of endothelial cells subjected to cyclic mechanical stretching. Ann. Biomed. Eng. 28(10):1165–1171, 2000.
Wang, D. L., et al. Cyclical strain increases endothelin-1 secretion and gene expression in human endothelial cells. Biochem. Biophys. Res. Commun. 195(2):1050–1056, 1993.
Wang, H., et al. Cell orientation response to cyclically deformed substrates: experimental validation of a cell model. J. Biomech. 28(12):1543–1552, 1995.
Wang, J. H., et al. Specificity of endothelial cell reorientation in response to cyclic mechanical stretching. J. Biomech. 34(12):1563–1572, 2001.
Wang, J. H., et al. Fibroblast responses to cyclic mechanical stretching depend on cell orientation to the stretching direction. J. Biomech. 37(4):573–576, 2004.
Yun, J. K., J. M. Anderson, and N. P. Ziats. Cyclic-strain-induced endothelial cell expression of adhesion molecules and their roles in monocyte–endothelial interaction. J. Biomed. Mater. Res. 44(1):87–97, 1999.
Zarins, C. K., et al. Carotid bifurcation atherosclerosis. Quantitative correlation of plaque localization with flow velocity profiles and wall shear stress. Circ. Res. 53(4):502–514, 1983.
Zhang, S., I. Day, and S. Ye. Nicotine induced changes in gene expression by human coronary artery endothelial cells. Atherosclerosis 154(2):277–283, 2001.
Zhao, S., et al. Synergistic effects of fluid shear stress and cyclic circumferential stretch on vascular endothelial cell morphology and cytoskeleton. Arterioscler. Thromb. Vasc. Biol. 15(10):1781–1786, 1995.
Zhao, S. Z., et al. Inter-individual variations in wall shear stress and mechanical stress distributions at the carotid artery bifurcation of healthy humans. J. Biomech. 35(10):1367–1377, 2002.
Acknowledgments
The authors acknowledge support from the Program for Research in Third Level Institutions (PRTLI), administered by the Higher Education Authority (HEA). The project was carried out at the National Centre for Biomedical Engineering Science (NCBES), National University of Ireland, Galway, in association with University of Limerick, and Institute of Technology, Sligo. L. Breen acknowledges funding from the Irish Research Council for Science, Engineering and Technology (IRCSET) under the Embark Initiative Postgraduate Research Scholarship Scheme.
Author information
Authors and Affiliations
Corresponding author
Additional information
Associate Editor Scott I. Simon oversaw the review of this article.
Rights and permissions
About this article
Cite this article
Breen, L.T., McHugh, P.E. & Murphy, B.P. HUVEC ICAM-1 and VCAM-1 Synthesis in Response to Potentially Athero-Prone and Athero-Protective Mechanical and Nicotine Chemical Stimuli. Ann Biomed Eng 38, 1880–1892 (2010). https://doi.org/10.1007/s10439-010-9959-8
Received:
Accepted:
Published:
Issue Date:
DOI: https://doi.org/10.1007/s10439-010-9959-8