Abstract
Drought is the main meteorological threat to plants and limits plant growth, development, and adaptation to environmental changes. However, root-shoot communication plays a vital role in improving tomato plant drought tolerance, especially when cultivars are grafted onto drought-tolerant rootstock. In this study, the relationship between photosynthetic capacity and reactive oxygen species (ROS) in response to drought stress was studied in tomato grafted with different drought-resistant tomato seedlings. To determine the drought-relieving effect of drought-tolerant rootstocks, we measured the effects of grafting on plant growth, net photosynthetic rate (Pn), ROS accumulation, and antioxidant enzyme activities in tomato leaves and roots under drought stress. Plant growth and Pn were significantly inhibited by drought, but ROS accumulation and antioxidant enzyme activities were significantly increased. Treatment with drought-tolerant tomato seedlings significantly increased plant growth and increased Pn under water-deficit conditions compared with those grafted with drought-susceptible rootstock. In addition, the plants grafted with drought-tolerant seedlings had increased activities of partial antioxidant enzymes, leading to decreased ROS production. Our results indicate that tomato grafted with drought-tolerant seedlings alleviated the phytotoxicity and oxidative damage caused by drought by regulating antioxidant enzymes under drought stress.
Similar content being viewed by others
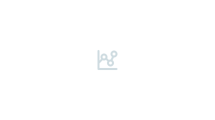
Avoid common mistakes on your manuscript.
Introduction
Drought is one of the most common abiotic stresses for plants around the world that inhibits plant growth and is becoming a major limitation to agricultural production (Łabędzki and Bąk 2017; Ray et al. 2018). In addition, the uneven distribution of rainfall caused by global climate change and the improper use of water resources caused by population are increasing the frequency and severity of drought in many regions (Bacon 2004; IPCC 2007). When subjected to water-deficit conditions, plants respond with a range of phenotypes through physiological and biochemical changes, such as plant wilting, reduced stomatal opening, and overproduction of reactive oxygen species (ROS) and malondialdehyde (MDA) in plant cells (Shinozaki and Yamaguchi-Shinozaki 2007; Liu et al. 2014). However, in response to the oxidative damage that results from the accumulation of ROS, mainly superoxide anion (O2·−), hydroxylradical (·OH), and hydrogen peroxides (H2O2), plants have evolved many protective mechanisms, such as the enzymatic antioxidant defense system and nonenzymatic antioxidant defense system (Kawasaki et al. 2001; Liu et al. 2016; Nahar et al. 2016). Improving the drought tolerance of crops and improving the water use efficiency of plants under drought conditions are a key goal of global agriculture.
According to records, when grafting was developed in the late 1920s, the main purpose of employing this technology was to offset soil pathogens; however, after decades of development, grafting now has a wide range of uses (Ke and Saltveit 1988; Lee et al. 2010). Grafting has promoted horticultural improvement and development by combining the excellent traits of rootstocks and scions to produce new plants, and it is used as a tool for vegetative propagation. It is a common practice, for example, in Japan, Korea, China, the Mediterranean basin, and several European countries, to graft vegetables onto appropriate rootstocks. In recent years, the grafting technique has been widely used not only to treat soil-borne pests and diseases but also to achieve a higher tolerance to abiotic stress conditions such as drought, salinity, nutrient stress, chilling stress, thermal stress, heavy metal, organic pollutants, and alkalinity (Martinez-Rodriguez et al. 2008; Rouphael et al. 2008; Savvas et al. 2009; Colla et al. 2010; Schwarz et al. 2010; Huang et al. 2013; Li et al. 2014b; Silva et al. 2018). Moreover, in terms of drought resistance, it has been confirmed that grafted apples, grapevines, cucumbers, and tomatoes have improved responses to drought (Liu et al. 2012; Liu et al. 2016; Yao et al. 2016; Pagliarani et al. 2017). However, the effect of scion/rootstock interaction on the adaptation of plants to stress remains controversial (Gambetta et al. 2012), and the mechanisms by which ROS metabolism contributes to differential drought resistance among tomato rootstocks are not well understood. Thus, the relative contribution of ROS metabolism to drought resistance and the differences obtained by the grafted combination of tomatoes need further research.
Tomato (Lycopersicon esculentum Mill.) is one of the most common vegetable crops cultivated worldwide and a model horticultural crop; it has a large growth volume, high yield, and relatively advanced root system. However, under water stress conditions, the photosynthetic rate (Pn) and the light saturation point of tomato decreased, while the light compensation point increased, resulting in decreased light energy use efficiency (Weerasinghe et al. 2003). Under severe water stress, tomato roots have a shallow distribution in the soil, and plant growth is inhibited, which eventually leads to a significant decrease in fruit weight and yield (Ozbahce and Tari 2010). Several studies have shown that grafting tomato onto stress-tolerant rootstocks might increase the tolerance of tomato plants to chilling or salinity (Ruiz et al. 2010; Ntatsi et al. 2014); however, relatively little is known about how Pn and ROS metabolism are influenced by rootstock. Our preliminary experiments showed that the tomato plants grafted onto drought-tolerant rootstocks showed higher water utilization efficiency than those grafted onto drought-susceptible rootstocks under water stress (Zhang et al. 2017). To further explore the feasibility of biological water savings, the response of plants grafted onto drought-tolerant and drought-susceptible seedlings to drought stress was compared by investigating Pn, Tr, ROS, and antioxidant enzymes.
Materials and methods
Plant materials and growth conditions
The experiment was conducted in a greenhouse located on the experimental farm of Shan Dong Agricultural University in Tai-An (36° 09′ N, 117° 09′ E) in eastern China from September to October 2017. Two different tomato genotypes (Zhang et al. 2016), drought-tolerant (“606,” T) and drought-susceptible (“112,” S), were used as plant materials. The seeds were immersed in water and then transferred to 28 °C for 72 h in darkness to germinate. After germination, the seeds of both species were sown directly into 50-cell plug trays filled with a mixture of substrate (peat:vermiculite:perlite = 1:1:1, by volume). When the cotyledons of the tomato had grown to the stage with two to three true leaves (3–4 weeks after sowing), an “oblique cutting grafting method” was performed. The resulting four groups of seedlings were designated as follows based on the tomato species: T/T (the combination of tolerant scion and tolerant rootstock), T/S (the combination of tolerant scion and susceptible rootstock), S/T (the combination of susceptible scion and tolerant rootstock), and S/S (the combination of susceptible scion and susceptible rootstock). When the grafted plants were well established, they were transferred into plastic pots (diameter 10 cm, height 12 cm; one seedling per pot) containing 1.5 kg substrate composed of sandy loam-soil/peat mixture (1:1, v/v) under the following environmental conditions: natural light conditions, photosynthetic photon flux density (PPFD) of 1400–1800 μmol m−2 s−1 (sunny day), and temperature of 28~37/18~21 °C (day/night). The plants were watered daily and fertilized with Hoagland’s nutrition solution at 2-day intervals.
Water-deficit treatments
To evaluate the scion-rootstock interaction under drought, the four tomato grafting combinations remained 40% of soil water content (40%SWC) as drought treatment; at the same time, the other plant group with well-watered (80% SWC) was used as control. Measuring soil moisture twice a day at 8:00 and 17:00 and irrigated by weighing method achieve the target water content. Each treatment was replicated three times, each of which included ten seedlings.
Growth analysis and analysis of chlorophyll fluorescence
After 15 days of treatment, every three plants were randomly selected from each treatment. The impact on the growth of tomato plants of different treatments was assessed by determining leaf and root fresh weight. The total leaf area was measured with a Li 3100 leaf area meter (LI-3100C; Lincoln, USA). The leaf water content (LWC) was measured by the drying method. Determining the fresh weight (FW) and dry weight (DW) of tomato leaves was calculated according to the following formula: LWC = [(FW−DW)/FW] × 100%. The chlorophyll content was assayed by the Arnon (1949) method.
The leaf relative electrolyte leakage (REL) was measured by the extravasation conductance method. The fresh samples were first rinsed with tap water and then rinsed twice with distilled water. Using a hole punch to take the leaf disc into the tube and put 10 in each. Each tube was filled with 20-ml distilled water and placed in a vacuum desiccator for 20 min. After standing for 1 h, the conductivity of the distilled water and the initial leaf solution were measured and recorded as S0 and S1, respectively. Then, the tube was placed in a 100 °C boiling water bath for 10 min. After cooling, the final conductivity S2 of the leaf solution was determined. Calculating REL was according to the formula: REL = [(S1−S0) / (S2−S0)] × 100%.
The photosynthetic and chlorophyll fluorescence parameters were measured at 0, 1, 5, 10, and 15 days after drought treatment and selected the third of the full-expansion function leaves from top to bottom. Photosynthetic parameters in fully expanded leaves, including net CO2 assimilation (Pn) and transpiration (Tr), were determined within the time period of 8:30 am to 10:30 am using a CIRAS-3 Portable Photosynthesis System (CIRAS-3; PP Systems, USA). The fluorescence parameters, including maximum photochemical efficiency of photosystem II (PSII) (Fv/Fm), effective PSII quantum yield (ΦPSII), and effective quantum use efficiency of PSII in the light-adapted state (Fv′/Fm′), were measured using an imaging-PAM chlorophyll fluorimeter equipped with a computer-operated PAM-control unit (IMAG-MIN/B; Heinz Walz, Effeltrich, Germany).
Determination of MDA, H2O2, and O2· − contents
The contents of MDA and hydrogen peroxide (H2O2) in leaves and roots were determined by the method of Xu et al. (2012). O2·− generation was measured following the method of Rauckman et al. (1979). The accumulation of H2O2 and O2·− in the leaves and roots was also visualized by 3,3′-diaminobenzidine 4HCl (DAB) and nitroblue tetrazolium (NBT) staining techniques (Xu et al. 2012), respectively.
Antioxidant enzyme activity assay
Approximately 0.5 g of frozen leaves and roots were extracted for antioxidant enzyme activity assays. Superoxide dismutase (SOD), catalase (CAT), and peroxidase (POD) activities were measured according to the methods described by Xu et al. (2012).
Statistical analysis
The data are presented as the mean of three replications and corresponding standard errors. Except for the data of photosynthetic and chlorophyll fluorescence parameters that were statistically analyzed by a two-way ANOVA, all results were submitted to one-way ANOVA using the statistical software package SPSS (SPSS 10 for Windows, 2001). The differences between the means were determined by Tukey’s least significant difference (LSD) test at P ≤ 0.05.
Results
Impact of grafting on tomato plant growth traits
To study whether plant tolerance to drought is altered by rootstock or scion, we performed reciprocal grafting between drought-tolerant (T) and drought-susceptible (S) seedlings. As shown in Fig. 1, after 15 days, plant growth status was suppressed with the SWC deficit for all grafting combinations. Without water stress treatment, all plants had similar leaf fresh weight and leaf area per plant except T/T (Fig. 2a, c). However, compared with their corresponding controls, all plants exposed to drought (Fig. 2a–c) resulted in significant reductions in the plant leaf and root fresh weight and leaf area per plant. Additionally, under well-watered conditions, all plants showed no significant differences in leaf water content, leaf relative electrolyte leakage (REL), and chlorophyll content (Fig. 2d–f). Drought-induced plants marked decreases in the leaf water content and chlorophyll content for both T/S and S/S plants. In contrast, under water deficit conditions, the REL of T/S and S/S was significantly higher than that of T/T plants, suggesting a lower degree of damage to the plant membrane system in T/T. Taken together, these phenotypic and physiological data suggested that drought-tolerant (T) seedling-grafted plants significantly attenuated drought-induced growth inhibition.
Responses of various grafting tomato seedlings to drought stress. a The leaf fresh weight. b Root fresh weight. c Leaf area per plant. d Leaf water content. e Relative electrolyte leakage (REL). f Chlorophyll content. All data were determined 15 days after drought treatment. The data are mean of three replicates ± SD and the different letters indicate a significant difference at P ≤ 0.05 according to Tukey’s test
Impact of grafting on photosynthesis and chlorophyll fluorescence
According to multiple comparisons, the photosynthetic and chlorophyll fluorescence parameters of tomato significantly differed among the four grafting combinations with drought stress and increasing treatment time (Figs. 3 and 4).
Dynamic changes of photosynthesis and transpiration in different grafting tomato leaves during 15 days of drought stress. Net photosynthetic rate (Pn, a, and b) and transpiration rate (Tr, c, and d) of tomato grafted plants under well-watered and drought stress conditions. Data in a and c are presented as the Pn and Tr in different grafting combinations and b and d are grafted tomato plants under well-watered and drought stress conditions. The data are mean of multiple comparison ± SD (n = 3)
Response of chlorophyll fluorescence parameters in various grafting tomato seedlings to drought stress. a The maximum photochemical efficiency of PSII (Fv/Fm) was determined 15 days after drought treatment. The underneath color code depicted in the image ranges from 0 (black) to 1 (purple). b, c The maximal photochemical efficiency (Fv/Fm). d, e The effective PSII quantum yield (ΦPSII). f, g Effective quantum use efficiency of PSII in the light-adapted state (Fv′/Fm′). Data are mean of multiple comparison ± SD (n = 3)
To further investigate the underlying photoprotective mechanisms in grafted tomato plants, the plants were exposed to drought stress and found to have similar Pn and Tr trends that gradually decreased with increasing processing time (Fig. 3a, c). Water deficit resulted in a more significant decrease in Pn and Tr for the S/S and T/S plants than for the T/T and S/T plants. The Pn of T/T, T/S, S/T, and S/S plants decreased abruptly after 10 days of water stress and reached the minimum values at the end of the experiment, with inhibition ratios of 25.63%, 31.23%, 29.07%, and 36.05%, of the Pn before water stress, respectively. Similarly, under drought stress, the Pn and Tr were significantly decreased and showed similar trends of sharp decline with increasing processing time (Fig. 3b, d). At the end of the experimental period, the Pn and Tr decreased to 43.44% and 45.07%, respectively, compared with the watered treatment. These results strongly suggested that the drought-tolerant (T) rootstock-grafted plants maintained the Pn and Tr parameters at relatively high levels and accordingly slightly promoted plant growth, particularly under water-deficit conditions.
Corroborating the previously described results for Pn, S/S plants displayed significantly decreased Fv/Fm, ΦPSII, and Fv′/Fm′ compared with T/T plants throughout the whole experiment (Fig. 4 a, b, d, f). At day 15 of treatment, the other plants also exhibited a strong decrease in these indices; compared with T/T, the Fv/Fm, ΦPSII, and Fv′/Fm′ were decreased by 1.74%, 20.23%, and 3.47%, respectively, in T/S plants; 0.93%, 9.83%, and 2.14%, respectively, in S/T plants; and 3.72%, 29.90%, and 6.54%, respectively, in S/S plants. Additionally, the results in Fig. 4 c, e, and g showed that Fv/Fm, ΦPSII, and Fv′/Fm′ decreased significantly with increasing drought treatment time compared with the control. This demonstrated that under drought stress, the absorption and utilization of light energy by PSII of tomato leaves were unbalanced and the photochemical efficiency was reduced. However, grafting to drought-tolerant rootstocks effectively restored the physiological function of PSII under water stress and maintained high photosynthetic capacity. These results suggest that the S rootstock had a poor ability to adapt to soil drought, while the T rootstock could enhance drought tolerance and water content in response to water deficit by decreasing transpiration.
Impact of grafting on MDA content and ROS accumulation
To evaluate the alleviating role of T/T and S/T on drought tolerance in grafted tomato seedlings, the effects of different grafted combinations on the contents of MDA, H2O2, and O2·− in the leaves and roots under drought stress were studied (Fig. 5). The results showed that there were no significant differences in the MDA content, H2O2 accumulation, and O2·− release rate of tomato leaves and roots in T/T and S/T plants or in S/S and T/T plants under well-watered conditions. However, in response to water deficit, all plants presented increased in MDA content and accumulation of H2O2 and O2·− in both tomato leaves and roots. In addition, in leaves, T/T and S/T plants had significantly reduced production of MDA with drought stress; the MDA content was increased by 21.87% and 43.97% in the T/T and S/T plants, respectively, compared with the same grafting combinations under watered conditions. The MDA content of T/S and S/S under drought stress increased by 49.04% and 95.32%, respectively, compared with the watered condition (Fig. 5b). Furthermore, T/T and S/T plants also had significantly decreased ROS accumulation (H2O2 and O2·−) in the leaves and roots of tomato compared with the S/S treatment, and they showed trends similar to that of MDA under drought conditions compared with watered conditions. Unlike the accumulation of H2O2, the MDA content and O2·− release rate of leaves were assumed to be higher than those of roots. For example, under water stress, the MDA content and O2·− release rate in S/S plant leaves were 9.69 nmol g−1 and 6.83 μmol g−1 min−1, respectively, while the MDA content and O2·− release rate were 2.73 nmol g−1 and 2.68 μmol g−1 min−1, respectively, in tomato roots (Fig. 5b, c, f, g).
Effect of different grafting tomato seedlings on the content of MDA and ROS accumulation under drought stress. a Accumulation of H2O2 (3,3′-diaminobenzidine (DAB) staining, upper panel) and O2·− (nitroblue tetrazolium (NBT) staining, lower panel). b, c Malondialdehyde (MDA) content. d, e Hydrogen peroxide (H2O2) accumulation. f, g Superoxide radical (O2·−) release rate. All data were determined 15 days after drought treatment. The data are means of three replicates (± SD). Means denoted by the same letter do not differ significantly at P ≤ 0.05 according to Turkey’s test. FW, fresh weight
Impact of grafting on the activities of antioxidant enzymes
To study the decrease in drought-induced oxidative stress observed with the T rootstock in the leaves and roots of tomato, a series of antioxidant enzymes responsible for ROS scavenging were quantified. These antioxidant enzymes, including SOD, CAT, and POD, had markedly increased activity in both the leaves and roots of grafted plants under drought treatment up to 10 days and then their levels decreased with further increasing the treatment time (Fig. 6). For example, under water stress, the T/T, S/T, T/S, and S/S plants had higher SOD activities in leaves at 10 days than at 5 days, which were increased by 27.53%, 27.48%, 31.12%, and 51.90%, respectively. Under drought stress, the T/T plant showed increased activities of antioxidant enzymes compared with the S/T, T/S, and S/S pants. Although the roots and leaves showed similar changes in antioxidant enzyme activity under water stress conditions, apart from the SOD activities, the CAT and POD activities in roots were higher than those in leaves. These findings demonstrated that the T rootstock plays a positive role in drought stress adaptation in grafted tomato plants by modulating antioxidant enzyme activities, thus alleviating ROS damage in plants.
Changes of antioxidant enzymes in different grafting tomato leaves and roots under drought stress. a, b Superoxide dismutase (SOD). c, d Catalase (CAT). e, f Peroxidase (POD). The values are means of three replicates (± SD). Different letters indicate a significant difference at P ≤ 0.05 according to Tukey’s test. FW, fresh weight
Discussion
Grafting onto stress-tolerant rootstocks could improve plant resistance to a variety of abiotic stresses; however, the fundamental mechanisms of such enhanced tolerance are largely unknown (Marguerit et al. 2012; Sánchez-Rodríguez et al. 2012; Wang et al. 2012; Lucas et al. 2013). Noticeably, in this study, we found that plants grafted with drought-tolerant tomato seedlings exhibited an effective strategy to cope with water deficit for 15 days, as indicated by the high level of biomass accumulation and the maintenance of good water status in the leaves under drought stress. These results are in accordance with Liu et al. (2016), who reported that cucumber plants with drought-resistant luffa as rootstocks had an increased tolerance against drought, such as increased biomass accumulation under drought stress. Rootstock could alter the tolerance of shoots against adversity stresses (Rivero et al. 2003; Schwarz et al. 2010; Li et al. 2014a; Li et al. 2014b); however, among different drought-resistant rootstocks, whether the rootstock or the scion is more influential is largely unknown. In the current study, by comparing the growth of grafted plants, we found that the rootstock indeed changed the growth status of the scions and that the T rootstock (drought-tolerant genotype) enhanced plant growth much more than the S rootstock (drought-susceptible genotype) reduced the capacity for growth. This finding was consistent with Liu et al. (2014), who suggested that grafting with drought-tolerant rootstocks in tobacco could improve drought tolerance by enhancing the activities of antioxidant enzymes and the expression of stress-responsive genes. In addition, Alan et al. (2007) reported that the grafting effect was dependent on the rootstocks, which determined the grafting effect on plant growth and yield. Venema et al. (2008) suggested that at low growth temperatures, tomato plants grafted onto cold-tolerant rootstocks still experienced significantly improved plant shoot growth. Tramontini et al. (2013) proposed that rootstocks play a major role in grapevine tolerance to water stress by controlling and adjusting the water supply to shoot transpiration demand.
The role of drought-tolerant grafting in the alleviation of the drought-induced effects on Pn, Tr, Fv/Fm, ΦPSII, and Fv′/Fm′ was evident under drought stress in tomato seedlings. Generally, the chlorophyll content reduction under drought is known as a typical sign of oxidative stress, which might be due to photooxidation and chlorophyll degradation of the pigment (Ahmedi et al. 2009; Dias et al. 2018). Thus, a reduction in the chlorophyll content is considered a drought response mechanism to minimize light absorption by chloroplasts. In the present work, we observed a significant decrease in chlorophyll content in the four grafted combination plants under water-deficit conditions, whereas in the drought-tolerant grafted plants, especially in T/T, a high chlorophyll content was maintained. Moreover, our results showed that S/S plants did not have an increased ability to absorb water, as indicated by the low water content under drought. These results indicated that the drought-tolerant rootstock could alleviate the inhibition of Pn and Tr induced by drought stress. In support of this notion, Liu et al. (2011) proposed that grafting muskmelon onto interspecific rootstocks enhances photosynthesis and sugar translocation in muskmelon leaves, and Marguerit et al. (2012) have shown that the rootstock control of scion transpiration and its acclimation to water deficit are controlled by different genes. Under normal conditions, the light energy absorbed by chloroplasts is mainly consumed in three ways: photosynthetic electron transport, chlorophyll fluorescence emission, and heat dissipation. Measuring the chlorophyll fluorescence can reflect the light energy absorption and utilization of photosystem II (PSII) (Iersel et al. 2016). The maximum photochemical efficiency of PSII (Fv/Fm) is often used as an indicator of PSII photoinhibition or stress damage (Calatayud and Barreno 2004). In our study, Fv/Fm, ФPSII, and Fv′/Fm′ all decreased with prolonged water stress, and the values in the plants grafted with T rootstock was higher than in other plants under drought conditions. This indicates that water stress led to a decrease in the opening of the PSII reaction center, the conversion efficiency of light and the energy used for carbon assimilation accumulation. Moreover, part of the excitation energy is consumed through the heat dissipation by the antenna pigments, which is consistent with the review from Mathobo et al. (2017).
Drought stress disrupts the balance of light energy capture and light energy utilization in the photosynthesis of plants and then affects photosynthetic activity (Chaitanya et al. 2003). Additionally, as the stomatal closure and photosynthesis decline, the Calvin cycle reduces the need for the reducing force NADPH, causing the excessive reduction of the photosynthetic electron transport chain and obstructing electron transport (Mittler et al. 2004). The excitation energy that causes excess light energy to be converted into the Mehler reaction is transferred to molecular oxygen, which becomes O2·−, a type of ROS (Wiese et al. 1998). In general, the production and elimination of ROS in plant cells are in a dynamic equilibrium. However, this balance is easily disrupted when plants are subjected to drought stress, resulting in excessive accumulation of ROS and aggravation of membranous peroxidation (Xing et al. 2016; Yi et al. 2016). As a result, the MDA content, membrane permeability, and electrolyte leakage increased, and the damage to the membrane of the plant became more severe. To counteract this stress, the antioxidant enzyme SOD composes the first line of cellular defense by detoxifying O2·− and producing H2O2. Together with SOD, the CAT and POD enzymes are considered to be the most effective antioxidant enzymes for preventing cellular damage (Menconi et al. 1995; Wang et al. 2009), and the activities of these enzymes increased at the early stage of water-deficit conditions in grafted plants, but declined in the later drought stage. In our study, the SOD, CAT, and POD activities of T/T grafted plants were much higher than those of the T/S, S/T, and S/S plants under drought stress conditions. This indicates that in the T/T grafted plants, the antioxidant enzyme system functions efficiently to scavenge overproduced H2O2 and thereby protects plants from ROS toxicity under drought stress. Accordingly, grafted plants with drought-tolerant rootstock acquire greater tolerance to water stress by developing an improved antioxidant system, which in turn leads to improved growth performance.
Conclusion
We present phenotypic and physiological evidence that the T rootstock is the key factor that determines the drought tolerance of tomato. As shown in Fig. 7, under drought stress, grafted plants with drought-tolerant tomato seedlings may enhance Pn, Fv/Fm, ФPSII, and Fv′/Fm′ by increasing antioxidative enzyme activity, decreasing ROS production rate, reducing chlorophyll decomposition, and decreasing chloroplast membrane damage. Therefore, the drought-tolerant rootstocks maintained an increased PSII photochemical activity, alleviated the inhibitory effect of water deficit stress on the growth of seedlings, and improved drought tolerance of tomato plants. The present study also showed the complexity of ROS metabolism in the leaves and roots. Further investigation is still needed to understand the mechanisms by which the scion and rootstock interaction is involved in plant tolerance to stress conditions.
The possible model for the antioxidative system in tomato plants under drought stress. Under drought stress conditions, upregulated SOD, CAT, and POD enzyme activity induces the amounts of ROS that can be scavenged then results in reducing the damage on chlorophyll and the reaction center of PSII, thus enhancing photosynthesis and improving stress tolerance. In this case, drought-tolerant roots can scavenge excessive ROS that protects plant cells against stress condition. H2O2, hydrogen peroxide; O2·−, superoxide radical; SOD, superoxide dismutase; CAT, catalase; POD, peroxidase; Chl, chlorophyll; PSII, photosystem II; Pn, net photosynthetic rate.
Abbreviations
- APX:
-
Ascorbate peroxidase
- CAT:
-
Catalase
- H2O2 :
-
Hydrogen peroxide
- Fv/Fm:
-
maximal photochemical efficiency
- Fv′/Fm′:
-
Effective quantum use efficiency of (photosystem II) PSII in the light-adapted state
- MA:
-
Malic acid
- MDA:
-
Malondialdehyde
- O2· − :
-
Superoxide anion
- Pn:
-
Net photosynthetic rate
- POD:
-
Peroxidase
- PSII:
-
Photosystem II
- REL:
-
Relative electrolyte leakage
- ROS:
-
Reactive oxygen species
- SOD:
-
Superoxide dismutase
- Tr:
-
Transpiration rate
References
Ahmedi CB, Rouina BB, Sensoy S, Boukhris M, Abdallah FB (2009) Changes in gas exchange, proline accumulation and antioxidative enzyme activities in three olive cultivars under contrasting water availability regimes. Environ Exp Bot 67:345–352
Alan Ö, Özdemir N, Günen Y (2007) Effect of grafting on watermelon plant growth, yield and quality. J Agron 6:362–365
Arnon DI (1949) Copper enzymes in isolated chloroplasts: polyphenoloxidase in Beta vulgaris. Plant Physiol 24:1–15
Bacon MA (2004) Water use efficiency in plant biology. Water Use Effic Plant Biol 45:329
Calatayud A, Barreno E (2004) Response to ozone in two lettuce varieties on chlorophyll a, fluorescence, photosynthetic pigments and lipid peroxidation. Plant Physiol Biochem 42:549–555
Chaitanya KV, Jutur PP, Sundar D, Reddy AR (2003) Water stress effects on photosynthesis in different mulberry cultivars. Plant Growth Regul 40:75–80
Colla G, Rouphael Y, Cardarelli M, Salerno A, Rea E (2010) The effectiveness of grafting to improve alkalinity tolerance in watermelon. Environ Exp Bot 68:283–291
Dias MC, Correia S, Serôdio J, Silva AMS, Freitas H, Santos C (2018) Chlorophyll fluorescence and oxidative stress endpoints to discriminate olive cultivars tolerance to drought and heat episodes. Sci Hortic 231:31–35
Gambetta GA, Manuck CM, Drucker ST, Shaghasi T, Fort K, Matthews MA, Walker MA, Mc E, A.J. (2012) The relationship between root hydraulics andscion vigour across Vitis rootstocks: what role do root aquaporins play? J Exp Bot 63:6445–6455
Huang Y, Li J, Hua B, Liu Z, Fan M, Bie Z (2013) Grafting onto different rootstocks as a means to improve watermelon tolerance to low potassium stress. Sci Hortic 149:80–85
Iersel MWV, Weaver G, Martin MT, Ferrarezi RS, Mattos E, Haidekker M (2016) A chlorophyll fluorescence-based biofeedback system to control photosynthetic lighting in controlled environment agriculture. J Am Soc Hortic Sci 141:169–176
IPCC (2007) Contribution of working groups I, II and III to the fourth assessment report of the intergovernmental panel on climate change Climate Change 2007: Synthesis report104 (Geneva, Switzerland)
Kawasaki S, Borchert C, Deyholos M, Wang H, Brazille S, Kawai K, Galbraith D, Bohnert HJ (2001) Gene expression profiles during the initial phase of salt stress in rice. Plant Cell 13:889–905
Ke D, Saltveit ME (1988) Plant hormone interaction and phenolic metabolism in the regulation of russet spotting in iceberg lettuce. Plant Physiol 88:1136–1140
Łabędzki LŁ, Bąk B (2017) Impact of meteorological drought on crop water deficit and crop yield reduction in polish agriculture. J Water Land Dev 34:181–190
Lee JM, Kubota C, Tsao SJ, Bie Z, Hoyos Echevarria P, Morra L, Oda M (2010) Current status of vegetable grafting: diffusion grafting techniques, automation. Sci Hortic 127:93–105
Li H, Liu SS, Yi CY, Wang F, Zhou J, Xia XJ, Shi K, Zhou YH, Yu JQ (2014a) Hydrogen peroxide mediates abscisic acid-induced hsp70 accumulation and heat tolerance in grafted cucumber plants. Plant Cell Environ 37:2768–2780
Li H, Wang F, Chen XJ, Shi K, Xia XJ, Considine MJ, Yu JQ, Zhou YH (2014b) The sub/supra-optimal temperature-induced inhibition of photosynthesis and oxidative damage in cucumber leaves are alleviated by grafting onto figleaf gourd/luffa rootstocks. Physiol Plant 152:571–584
Liu YF, Qi HY, Bai CM, Qi MF, Xu CQ, Hao JH, Li Y, Li TL (2011) Grafting helps improve photosynthesis and carbohydrate metabolism in leaves of muskmelon. Int J Biol Sci 7:1161–1170
Liu B, Li M, Cheng L, Liang D, Zou Y, Ma F (2012) Influence of rootstock on antioxidant system in leaves and roots of young apple trees in response to drought stress. Plant Growth Regul 67:247–256
Liu J, Li J, Su X, Xia Z (2014) Grafting improves drought tolerance by regulating antioxidant enzyme activities and stress-responsive gene expression in tobacco. Environ Exp Bot 107:173–179
Liu S, Li H, Lv X, Ahammed GJ, Xia X, Zhou J, Shi K, Asami T, Yu J, Zhou Y (2016) Grafting cucumber onto luffa improves drought tolerance by increasing ABA biosynthesis and sensitivity. Sci Rep 6:20212
Lucas WJ, Groover A, Lichtenberger R, Furuta K, Yadav SR, Helariutta Y, He XQ, Fukuda H, Kang J, Brady SM, Patrick JW, Sperry J, Yoshida A, López-Millán AF, Grusak MA, Kachroo P (2013) The plant vascular system: evolution, development and functions. J Integr Plant Biol 55:294–388
Marguerit E, Brendel O, Lebon E, Van Leeuwen C, Ollat N (2012) Rootstock control of scion transpiration and its acclimation to water deficit are controlled by different genes. New Phytol 194:416–429
Martinez-Rodriguez MM, Estan MT, Moyano E, Garcia-Abellan JO, Flores FB, Campos JF, Al-Azzawi MJ, Flowers TJ, Bolarin MC (2008) The effectiveness of grafting to improve salt tolerance in tomato when an ‘excluder’ genotype is used as scion. Environ Exp Bot 63:392–401
Mathobo R, Marais D, Steyn JM (2017) The effect of drought stress on yield, leaf gaseous exchange and chlorophyll fluorescence of dry beans (Phaseolus vulgaris, l). Agric Water Manag 180:118–125
Menconi M, Sgherri CLM, Pinzino C, Navari-Izzo F (1995) Activated oxygen production and detoxification in wheat plants subjected to a water deficit programme. J Exp Bot 46:1123–1130
Mittler R, Vanderauwera S, Gollery M, Van BF (2004) Reactive oxygen gene network of plants. Trends Plant Sci 9:490–498
Nahar K, Hasanuzzaman M, Alam MM, Rahman A, Suzuki T, Fujita M (2016) Polyamine and nitric oxide crosstalk: antagonistic effects on cadmium toxicity in mung bean plants through upregulating the metal detoxification, antioxidant defense and methylglyoxal detoxification systems. Ecotoxicol Environ Saf 126:245–255
Ntatsi G, Savvas D, Kläring HP, Schwarz D (2014) Growth, yield, and metabolic responses of temperature-stressed tomato to grafting onto rootstocks differing in cold tolerance. J Am Soc Hortic Sci 139:230–243
Ozbahce A, Tari AF (2010) Effects of different emitter space and water stress on yield and quality of processing tomato under semi-arid climate conditions. Agric Water Manag 97:1405–1410
Pagliarani C, Vitali M, Ferrero M, Vitulo N, Incarbone M, Lovisolo C, Valle G, Schubert A (2017) Accumulation of microRNAs differentially modulated by drought is affected by grafting in grapevine. Plant Physiol 173:2180–2195
Rauckman EJ, Rosen GM, Kitchell BB (1979) Superoxide radical as anointer mediate in the oxidation of hydroxylamines by mixed function amine oxidase. Mol Pharmacol 15:131–137
Ray RL, Fares A, Risch E (2018) Effects of drought on crop production and cropping areas in Texas. Agric Environ L 3(1)
Rivero RM, Ruiz JM, Romero L (2003) Role of grafting in horticultural plants. J Food Agric Environ 1:70–74
Rouphael Y, Cardarelli M, Rea E, Colla G (2008) Grafting of cucumber as a means to minimize copper toxicity. Environ Exp Bot 63:49–58
Ruiz JM, Blasco B, Rivero RM, Romero L (2010) Nicotine-free and salt-tolerant tobacco plants obtained by grafting to salinity-resistant rootstocks of tomato. Physiol Plant 124:465–475
Sánchez-Rodríguez E, Rubio-Wilhelmi MM, Blasco B, Leyva R, Romero L, Ruiz JM (2012) Antioxidant response resides in the shoot in reciprocal grafts of drought-tolerant and drought-susceptible cultivars in tomato under water stress. Plant Sci 188:89–96
Savvas D, Papastavrou D, Ntatsi G, Ropokis A, Olympios C, Hartmann H, Schwarz D (2009) Interactive effects of grafting and manganese supply on growth yield, and nutrient uptake by tomato. Hortic Science 44:1978–1982
Schwarz D, Rouphael Y, Colla G, Venema JH (2010) Grafting as a tool to improve tolerance of vegetables to abiotic stresses: thermal stress, water stress and organic pollutants. Sci Hortic 127:162–171
Shinozaki K, Yamaguchi-Shinozaki K (2007) Gene networks involved in drought stress response and tolerance. J Exp Bot 58:221–227
Silva VA, Prado FM, Antunes WC, Paiva RMC, Ferrão MAG, Andrade AC, Mascio PD, Loureiro ME, DaMatta FM, Almeida AM (2018) Reciprocal grafting between clones with contrasting drought tolerance suggests a key role of abscisic acid in coffee acclimation to drought stress. Plant Growth Regul:1–9
Tramontini S, Vitali M, Centioni L, Schubert A, Lovisolo C (2013) Rootstock control of scion response to water stress in grapevine. Environ Exp Bot 93:20–26
Venema JH, Dijk BE, Bax JM, Hasselt PRV, Elzenga JTM (2008) Grafting tomato (Solanum lycopersicum) onto the rootstock of a high-altitude accession of Solanum habrochaites improves suboptimal-temperature tolerance. Environ Exp Bot 63:359–367
Wang WB, Kim YH, Lee HS, Kim KY, Deng XP, Kwak SS (2009) Analysis of antioxidant enzyme activity during germination of alfalfa under salt and drought stresses. Plant Physiol Biochem 47:570–577
Wang S, Liang D, Li C, Hao Y, Ma F, Shu H (2012) Influence of drought stress on the cellular ultrastructure and antioxidant system in leaves of drought-tolerant and drought- susceptible apple rootstocks. Plant Physiol Biochem 51:81–89
Weerasinghe OR, De Costa WAJM, Perera ALT (2003) Evaluation of different genotypes of tomato under well watered and water stressed conditions on the basis of yield and some selected physiological parameters. Tropical Agricultural Research Vol, 144–156
Wiese C, Shi LB, Heber U (1998) Oxygen reduction in the Mehler reaction is insufficient to protect photosystems I and II of leaves against photoinactivation. Physiol Plant 102:437–446
Xing XH, Fang CW, Long LI, Jiang HQ, Qin Z, Jiang HD, Wang SH (2016) Improved drought tolerance by α-naphthaleneacetic acid-induced ROS accumulation in two soybean cultivars. J Integr Agric 15:1770–1784
Xu J, Zhu Y, Ge Q, Li Y, Sun J, Zhang Y, Liu X (2012) Comparative physiological responses of Solanum nigrum and Solanum torvum to cadmium stress. New Phytol 196:125–138
Yao X, Yang R, Zhao F, Wang S, Li C, Zhao W (2016) An analysis of physiological index of differences in drought tolerance of tomato rootstock seedlings. J Plant Biol 59:311–321
Yi X, Burgess P, Zhang X, Huang B (2016) Enhancing cytokinin synthesis by overexpressing ipt alleviated drought inhibition of root growth through activating ROS-scavenging systems in Agrostis stolonifera. J Exp Bot 67:1979–1992
Zhang ZH, Han M, Zhang Y, Wang Y, Xu K (2016) Identification and evaluation of tomato rootstock seedlings for drought tolerance. Chin J Ecol 35:719–725 (in Chinese)
Zhang ZH, Han M, Zhang Y, Wang Y, Liu CY, Cao BL, Xu K (2017) Effect of water stress on development and H2O and CO2 exchange in leaves of tomato grafted with different drought resistant rootstocks. Sci Agric Sin 50:391–398 (in Chinese)
Funding
This work was supported by the Special Foundation for Modern Agricultural Industry Technology System of Shandong Province (no. SDAIT-05-05) and the Double First-Class Discipline Construction Project of Shandong Province (no. SYL2017YSTD06).
Author information
Authors and Affiliations
Contributions
Zhihuan Zhang and Kun Xu designed the experiments; Zhihuan Zhang, Bili Cao, and Song Gao performed the experiments; and Zhihuan Zhang and Kun Xu wrote the manuscript.
Corresponding author
Ethics declarations
Conflict of interest
The authors declare that they have no conflict of interest.
Additional information
Handling Editor: Peter Nick
Publisher’s note
Springer Nature remains neutral with regard to jurisdictional claims in published maps and institutional affiliations.
Rights and permissions
About this article
Cite this article
Zhang, Z., Cao, B., Gao, S. et al. Grafting improves tomato drought tolerance through enhancing photosynthetic capacity and reducing ROS accumulation. Protoplasma 256, 1013–1024 (2019). https://doi.org/10.1007/s00709-019-01357-3
Received:
Accepted:
Published:
Issue Date:
DOI: https://doi.org/10.1007/s00709-019-01357-3