Abstract
The aim of this study was to evaluate the role of endoplasmic reticulum (ER) stress in diabetic retinopathy (DR) using in vitro and in vivo models. For the in vivo studies, diabetes was induced in rats, and retinal expression of glucose-regulated protein 78 (GRP78), activating transcription factor 4 (ATF4), C/EBP homologous protein (CHOP), and vascular endothelial growth factor (VEGF) in groups of rats at 1, 3, and 6 months was assessed. For the in vitro studies, human retinal capillary endothelial cells (HRCECs) were cultured in the presence of varying glucose concentrations, and the expression of mRNA and protein for GRP78, ATF4, CHOP, and VEGF was assessed. The chosen glucose concentrations were reflective of those apparent in diabetic patients. Expression of VEGF and CHOP mRNA levels were significantly increased in diabetic rats compared to control rats at 1, 3, and 6 months (P < 0.05). In HRCECs cultured in the presence of high as well as variable glucose concentrations, CHOP expression and apoptosis were significantly increased (P < 0.05). However, GRP78 and ATF4 expression levels were unchanged. Our findings suggest that early progression of DR may be mediated by ER stress, but probably does not involve changes in ATF4 or GRP78.
Similar content being viewed by others
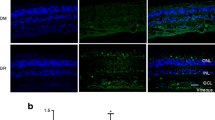
Avoid common mistakes on your manuscript.
Introduction
The incidence of diabetic retinopathy (DR), the major cause of blindness, has been increasing in recent times [1]. Indeed, more than 60% of patients with type 2 diabetes mellitus have DR [2]. Despite the significance of this problem, there currently exists no effective strategy to prevent or treat DR, which reflects the fact that the mechanisms underlying DR are incompletely understood.
In the past decade, the field of research concerning endoplasmic reticulum (ER) stress has expanded rapidly and enhanced our understanding of the molecular pathways that enable cellular adaptation to fluctuations in ER homeostasis [3]. ER stress is defined as “an imbalance between the cellular demand for ER function and ER capacity” [3], and results from accumulation of unfolded/mis-folded protein [4]. There is evidence suggesting that ER stress can lead to pancreatic β-cell apoptosis, inhibited insulin receptor expression, insulin resistance, and ultimately the development of type 2 diabetes [5, 6]. Given these findings, it is hardly surprising that ER stress has also been proposed to be an underlying mediator of DR [7]. Indeed, more recently, Ikesugi et al. [8] reported that ER stress in cultured human retinal pericytes was increased after hypoglycemia and when glucose concentrations were reduced from high to low. These findings are important as they imply that fluctuations in blood glucose concentrations (such as those that occur with diabetes) may lead to retinal ER stress.
Endoplasmic reticulum stress can lead to upregulated expression of glucose-regulated protein 78 (GRP78) [9, 10], activating transcription factor 4 (ATF4), and VEGF [11]. GRP78 is a heat shock protein involved in the unfolded protein response, while ATF4 plays an important role in cellular oxidative stress protection and is upregulated in response to diminished or impaired cellular protein synthesis. ATF4 has also been reported to regulate VEGF transcription [11], which in turn has been linked to the occurrence of DR [12].
Apoptotic pathways are ultimately induced if the ER stress can not be relieved by protein folding and degradation [3]. One factor involved in ER stress-mediated apoptosis pathways is C/EBP homologous protein (CHOP), also known as growth arrest and DNA damage-inducible gene 153 [13]. While it has been suggested that early pathological changes associated with DR may involve apoptosis, it is not clear whether CHOP expression is altered.
Given the reported findings, the aim of the present study was to further investigate the possible involvement of ER stress in mediating DR using both in vitro and in vivo models. For the in vivo studies, we induced diabetes and assessed retinal expression of GRP78, ATF4, CHOP, and VEGF in groups of rats at 1, 3, and 6 months. For the in vitro studies, human retinal capillary endothelial cells (HRCECs) were cultured in medium containing varying concentrations of glucose, and the expression of mRNA and protein for GRP78, ATF4, CHOP, and VEGF was assessed. The glucose concentrations used were chosen to reflect those apparent in diabetic patients.
Materials and methods
Animal model of diabetes
Sixty male Sprague–Dawley rats (2-month-old; weighing between 150 and 200 g) were obtained from the animal center of Huazhong University of Science and Technology and maintained in an optimal environment for 2 weeks before experimentation. Thirty rats were randomly selected and given a single intraperitoneal injection of streptozotocin (STZ: 65 mg/kg in 0.01 M citrate buffer at pH 4.5) after fasting for 12 h to induce diabetes. The remaining 30 rats were used as nondiabetic controls and were injected with phosphate-buffered saline only.
Fasting plasma glucose concentrations were assessed by Glucometer (Touch; Boehringer Mannheim Diagnostics, San Francisco, CA) 3 days after the STZ injection. Diabetes was confirmed if plasma glucose values were 16.7 mmol/l or higher. Retinas were then isolated from groups (with or without diabetes induction) of rats at 1, 3, and 6 months and expression of GRP78, ATF4, CHOP, and VEGF was determined.
All procedures involving animals were approved by the experimental animal management committee of Huazhong University of Science and Technology.
Quantitative real-time polymerase chain reaction
Total RNA from rat retinal tissue was obtained using Trizol reagent (Gibco, Inc., Los Angeles, CA). After purification and on-column DNase digestion using a kit (RNeasy Mini Kit; Qiagen, Inc., Hilden, Germany) was performed, the RNA was quantified and its quality assessed. cDNA was then synthesized using a SuperScript II cDNA synthesis kit (Invitrogen Inc., Carlsbad, CA).
Quantitative real-time polymerase chain reaction (qRT–PCR) was performed by using a kit (HotStart, Taq polymerase kit; Qiagen, Inc.) with SYBR Green technology (ABI Inc., Tampa, FL). The thermocycling program consisted of 32 cycles, with an initial cycle at 95°C for 15 min to activate the polymerase. Melting-curve analysis was performed at 72°C by monitoring FAM/SYBR fluorescence. PCR-specific products were determined by using the melting curves to show a clear single peak at a melting temperature of approximately 72°C. Expression of the GRP78, ATF4, CHOP, and VEGF genes was analyzed in normal and diabetic retinas, with β-actin used as an internal control. Table 1 shows the qRT–PCR primer pairs used for determining gene expression in rat retinal tissue.
Culture and characterization of human retinal capillary endothelial cells
Twelve donated human eyes were obtained from the Tongji Hospital Eye Bank (Wu Han, China). The donors were eight male and four female healthy accident victims with a mean age of 33.5 years. Informed consent was obtained from the relevant parties, and the hospital internal review board approved the study.
Cultured HRCECs were established as previously described [14]. In brief, the donor eyes were cut circumferentially 3 mm posterior to the limbus, and the retinas were harvested and homogenized by two gentle up/down strokes in a 15-ml Dounce homogenizer. The homogenate was subjected to centrifugation (1,000 g for 10 min) after filtration through an 88-μm sieve. The pellet was resuspended in human endothelial serum-free medium (SFM), basal growth medium (Gibco, Grand Island, NY) supplemented with 20% fetal bovine serum, 50 U/ml endothelial cell growth factor (Sigma, St. Louis, MO), and 1% insulin-transferrin-selenium.
Cells were then transferred to culture dishes coated with fibronectin and incubated at 37°C in a humidified atmosphere containing 5% CO2. The cultured cells were characterized for endothelial homogeneity by testing their activity against factor VIII antigen (von Willebrand factor) and by measuring Acetyl-LDL (Ac-LDL) receptors in the endothelial cells with added fluorescence-labeled Ac-LDL (Biomedical Technologies, Shanghai, China). Only cells from passages 3 to 7 were used in the experiments.
The HRCECs were treated with human endothelial SFM basal growth medium (containing 8.3 mM glucose) for 1 day and then divided into 4 groups and treated with medium containing varying concentrations of glucose as outlined in Table 2 (modified from a previous study by Ikesugi et al. [8]) for 2 days.
Analysis of cellular apoptosis
Cellular apoptosis was analyzed using propidium iodide to stain the damaged DNA. Cultured cells were trypsinized, pelleted, and resuspended in one volume of ice-cold phosphate-buffered saline. The suspension was gently agitated while three volumes of ice-cold 95% ethanol were slowly added. Cold reagents and a gradual addition of ethanol were used to reduce clumping of the cells, which were then pelleted and resuspended in equal volumes of 30 μg/ml propidium iodide and 100 μg/ml RNAse A (both in phosphate-buffered saline). Stained cells were stored overnight at 4°C and protected from light until analysis. Flow cytometry (FACSCAN, Beckman coulter Inc., Fullerton, CA) was performed to determine the percentage of cells in apoptosis.
TUNEL method
The slides to which cultured cells have been attached were fixed with 4% paraformaldehyde for 25 min, washed twice with PBS, dropped 100 μl of equilibration buffer, then incubated at the room temperature for 5–10 min. The slides were washed with 0.3% H2O2 for 3–5 min, then immersed and washed three times with PBS. After dropping TUNEL reaction mixture (Yuanxiang company, shanghai, China), the slides were then put into a wet box and incubated at 37°C for 1 h. Following washing the slides 3 times with PBS for 5 min, the DAB color reaction was performed. The cytoplasm with light-brown staining was considered to be positive. The number of apoptotic cells was calculated after the random observation of eight high-power fields under optical microscope.
RT–PCR
Total RNA from HRCECs for the four groups was isolated by RNeasy Mini Kit (Qiagen, Inc.) according to the manufacturer’s instructions. Reverse transcription (RT)-PCR were performed using an Omniscript RT kit (Qiagen, Inc.) according to the manufacturer’s instructions. After quantification, RNA was reverse transcribed into cDNA using a SuperScript II RT kit (Invitrogen Inc.). PCR conditions were initial denaturation at 95°C for 5 min, 30 cycles of 30 s denaturation at 95°C, 30 s of primer annealing at the primer-specific temperature (Table 3), and 30 s of elongation at 72°C. After 30 cycles, a final elongation step took place for 5 min. Equal amounts of the PCR products were separated on 1.5% agarose gels and visualized by means of ethidium bromide staining.
Western blotting
Total protein was extracted from transfected HRCECs using bicinchoninic acid. The protein was mixed with loading buffer, denatured for 6 min at 60°C, cooled, centrifuged for 5 min, and separated by means of sodium-dodecyl sulfate–polyacrylamide gel electrophoresis. To probe for the proteins, several antibodies were used: monoclonal anti-CHOP (Santa Cruz Biotechnology Inc., Santa Cruz, CA), polyclonal anti-ATF4 (Santa Cruz Biotechnology Inc), monoclonal anti-GRP78 (BD Bio Sciences, San Jose, CA), and monoclonal anti-VEGF (Santa Cruz Biotechnology Inc.). A secondary antibody, goat anti-mouse Ig (BD Biosciences, Franklin Lakes, NJ) diluted 1:1000, was applied; and the chemiluminescent signal was detected. The same polyvinylidene fluoride membrane was reused to detect β-actin (the internal control) by incubating it with mouse anti-human actin antibody (Gene Co., Hong Kong, China). Bands observed on the films were analyzed by automatic image analysis. The integrated optical density of each protein band was normalized to that of the corresponding β-actin band from the same sample.
Statistical analysis
Data are presented as the mean ± standard deviation (SD). Normally distributed data were compared by independent two-sample t-test or one-way analysis of variance where appropriate. When a significant between-group difference was detected, multiple comparisons of the means were made using the Ryan-Einot-Gabriel-Welsch (REGWQ) procedure, a multiple range test, by holding the total type-I error rate at a maximum of 0.05. Statistical significance was set at 0.05 for all analyses. All analyses were performed using SAS software (version 9.1.3 for Windows; SAS Institute Inc, Cary, NC).
Results
Expression of VEGF, ATF4, GRP78, and CHOP genes in retinal tissue of diabetic rats
These results are presented in Fig. 1. Retinal VEGF and CHOP mRNA levels were significantly higher in STZ-induced diabetic compared to controls rats at 1, 3, and 6 months (P < 0.05). There were also several between time point differences apparent for VEGF and CHOP expression levels in diabetic rats (see Fig. 1, P < 0.05). No differences were detected with regard to the expression of ATF4 and GRP78.
Expression of VEGF, ATF4, GRP78, and CHOP genes in retinal tissue of diabetic rats. VEGF (a), ATF4 (b), GRP78 (c), and CHOP (d) mRNA expressions in rat retinal cells 1, 3, or 6 months after induction of diabetes with streptozotocin were measured by qRT–PCR. Data were compared by independent two-sample t-test and analysis of variance (multiple comparisons of the means were made using the REGWQ test). Statistically significant between-group differences (P < 0.05) are indicated
Apoptosis in HRCECs cultured with different glucose concentrations
The levels of apoptosis in HRCECs with respect to glucose concentration are presented in Fig. 2. Apoptosis levels were significantly lower in Group 1 compared to all other groups. HRCECs treated with 25 mM glucose for 24 h, then with 8.3 mM glucose for 24 h exhibited the highest levels of apoptosis when compared with each individual group. Results of TUNEL assay were shown in Fig. 3. The number of apoptotic cells was 7.3 ± 1.1 in Group 1 (Fig. 3a), 12.3 ± 2.4 in Group 2 (Fig. 3b), 10.3 ± 1.5 in Group 3 (Fig. 3c), and 16.3 ± 1.7 in Group 4 (Fig. 3d). There were less apoptotic cells in Group 1 than the other three groups, while there were more apoptotic cells in Group 4 than other three groups. The difference between them was significant.
Apoptosis levels in HRCECs cultured with different glucose concentrations (n = 8). Groups 1, 2, and 3 represent cells grown in culture media containing 8.3, 25, and 50 mM glucose, respectively. Group 4 HRCECs were cultured in media containing 25 mM and then 8.3 mM glucose. Analysis of variance and multiple comparisons of means were performed using the REGWQ test. Statistically significant between-group differences (P < 0.05) are indicated
Gene expression and protein levels
Gene expression and protein levels of VEGF, ATF4, GRP78, and CHOP in HRCECs treated with different concentrations of glucose were examined. There were no significant differences found in the expression levels of the VEGF, ATF4, and GRP78 genes or proteins among the groups (Table 4; representative images are presented in Figs. 4, 5). In contrast, expression of the CHOP gene and protein was significantly higher in Groups 2, 3, and 4 compared to Group 1 (Table 4; representative images are presented in Figs. 4, 5).
RT-PCR for mRNA expression in HRCECs. VEGF (a), ATF4 (b), GRP78 (c), and CHOP (d) mRNA expression in HRCECs as evaluated by RT-PCR. Groups 1, 2, and 3 represent cells grown in culture media containing 8.3, 25, and 50 mM glucose, respectively. Group 4 HRCECs were cultured in media containing 25 mM and then 8.3 mM glucose
Western blot for protein levels in HRCECs. VEGF (a), ATF4 (b), GRP78 (c), and CHOP (d) protein levels in HRCECs as determined by Western blot. Groups 1, 2, and 3 represent cells grown in culture media containing 8.3, 25, and 50 mM glucose, respectively. Group 4 HRCECs were cultured in media containing 25 mM and then 8.3 mM glucose
Discussion
Increase and fluctuation in blood glucose concentrations are two major causes of retinal vascular injury in diabetic patients [15, 16]. The effect of ER stress on the pathogenesis of DR has gradually been revealed, but it is not yet entirely clear. Therefore, continued study of the relationship between changes in blood glucose concentrations and the expression of factors related to ER stress in retinal cells is warranted to improve our understanding of the importance of ER stress in DR.
In the present study, where the early stage of DR was studied, we demonstrated that expression of CHOP in the retina was upregulated in rats with STZ-induced diabetes. Further to this, we observed a significant increase in apoptosis in HRCECs cultured with high and variable glucose concentrations. These changes paralleled those in CHOP expression. Given that CHOP is a known ER-stress protein and that ER stress has been demonstrated to occur as a consequence altered glucose concentrations [17], our findings suggest that the increased CHOP expression observed may have been a consequence of ER stress. Ikesugi et al. [8] also reported glucose concentration related changes in CHOP expression and apoptosis in a series of similar studies using retinal pericytes. It was also noted that CHOP expression was upregulated when cells were cultured in the absence of glucose.
Our in vivo studies were short-term, examining several of the initial mechanisms possibly related to ER stress in DR. Expression levels of both GRP78 and ATF4 were not significantly upregulated in our rat model of diabetes. Furthermore, HRCECs cultured in the presence of high glucose concentrations did not exhibit increased GRP78 and ATF4 expression, despite the previously mentioned increases in apoptosis and CHOP. In agreement with this finding, Ikesugi et al. [8] also found that GRP78 expression did not increase in retinal pericytes exposed to high glucose concentrations. It would appear that GRP78 is not an early mediator of ER stress in DR and that the apoptosis observed is independent of the unfolded protein response. Interestingly, Ikesugi et al. [8] also found that GRP78 expression was induced when glucose concentrations were reduced from high to below normal levels, indicating that the mechanisms underlying apoptosis differ with different prevailing glucose environments.
There are three predominant pathways thought to be activated during ER stress. These include those involving PKR-like endoplasmic reticulum kinase (PERK), ATF6, and immunoreactive elastase (IRE). PERK activation has been shown to result in downstream activation of eIF2α, ATF4, and CHOP [13, 18–20]. However, given that ATF4 was not found to be upregulated in the present study, it would seem that increased activation of one or both of the other pathways may underlie the increased CHOP expression observed in response to glucose. Further studies are warranted to determine whether expression levels of other markers of ER stress such as IRE, ATF6, and indeed PERK are altered by glucose.
It has previously been demonstrated that expression of VEGF associated with ER stress is due to activation of the ATF4 pathway [21]. In the current study, retinal expression of VEGF was increased in diabetic rats despite the lack of change in ATF4 expression. Thus, it would appear that factors aside from ATF4 regulate VEGF expression at this time.
In summary, our findings suggest that the early progression of DR may well be mediated by ER stress, but that this likely does not involve changes in ATF4 or GRP78. Future studies should aim to explore the possible involvement of other regulatory sensors involved in ER stress. In addition, in vitro and in vivo studies are warranted to determine how more prolonged changes in glucose homeostasis influence ER stress and whether ER stress is more apparent in middle/later stages of diabetes in rats.
References
Mohamed Q, Gillies MC, Wong TY (2007) Management of diabetic retinopathy: a systematic review. JAMA 298:902–916
Fong DS, Aiello L, Gardner TW, King GL, Blankenship G, Cavallerano JD et al (2004) Retinopathy in diabetes. Diabetes Care 27(Suppl 1):S84–S87
Ni M, Lee AS (2007) ER chaperones in mammalian development and human diseases. FEBS Lett 581:3641–3651
Kim R, Emi M, Tanabe K, Murakami S (2006) Role of the unfolded protein response in cell death. Apoptosis 11:5–13
Cardozo AK, Ortis F, Storling J, Feng YM, Rasschaert J, Tonnesen M et al (2005) Cytokines downregulate the sarcoendoplasmic reticulum pump Ca2+ ATPase 2b and deplete endoplasmic reticulum Ca2+, leading to induction of endoplasmic reticulum stress in pancreatic beta-cells. Diabetes 54:452–461
Ozcan U, Cao Q, Yilmaz E, Lee AH, Iwakoshi NN, Ozdelen E et al (2004) Endoplasmic reticulum stress links obesity, insulin action, and type 2 diabetes. Science 306:457–461
Gariano RF, Gardner TW (2005) Retinal angiogenesis in development and disease. Nature 438:960–966
Ikesugi K, Mulhern ML, Madson CJ, Hosoya K, Terasaki T, Kador PF et al (2006) Induction of endoplasmic reticulum stress in retinal pericytes by glucose deprivation. Curr Eye Res 31:947–953
Misra UK, Pizzo SV (2005) Up-regulation of GRP78 and antiapoptotic signaling in murine peritoneal macrophages exposed to insulin. J Leukoc Biol 78:187–194
Rao RV, Ellerby HM, Bredesen DE (2004) Coupling endoplasmic reticulum stress to the cell death program. Cell Death Differ 11:372–380
Roybal CN, Hunsaker LA, Barbash O, Vander Jagt DL, Abcouwer SF (2005) The oxidative stressor arsenite activates vascular endothelial growth factor mRNA transcription by an ATF4-dependent mechanism. J Biol Chem 280:20331–20339
Oskolkova OV, Afonyushkin T, Leitner A, von Schlieffen E, Gargalovic PS, Lusis AJ et al (2008) ATF4-dependent transcription is a key mechanism in VEGF up-regulation by oxidized phospholipids: critical role of oxidized sn-2 residues in activation of unfolded protein response. Blood 112:330–339
Oyadomari S, Mori M (2004) Roles of CHOP/GADD153 in endoplasmic reticulum stress. Cell Death Differ 11:381–389
Li B, Tang SB, Hu J, Gao Y, Zhang G, Lin SF et al (2006) Protective effects of transcription factor HESR1 on retinal vasculature. Microvasc Res 72:146–152
Santos KG, Tschiedel B, Schneider JR, Souto KE, Roisenberg I (2005) Prevalence of retinopathy in Caucasian type 2 diabetic patients from the South of Brazil and relationship with clinical and metabolic factors. Braz J Med Biol Res 38:221–225
UK Prospective Diabetes Study (UKPDS) Group (1998) Intensive blood-glucose control with sulphonylureas or insulin compared with conventional treatment, risk of complications in patients with type 2 diabetes (UKPDS 33). Lancet 352:837–853
Welihinda AA, Tirasophon W, Kaufman RJ (1999) The cellular response to protein misfolding in the endoplasmic reticulum. Gene Expr 7:293–300
Harding HP, Novoa I, Zhang Y, Zeng H, Wek R, Schapira M et al (2000) Regulated translation initiation controls stress-induced gene expression in mammalian cells. Mol Cell 6:1099–1108
Wang XZ, Lawson B, Brewer JW, Zinszner H, Sanjay A, Mi LJ et al (1996) Signals from the stressed endoplasmic reticulum induce C/EBP-homologous protein (CHOP/GADD153). Mol Cell Biol 16:4273–4280
Oyadomari S, Koizumi A, Takeda K, Gotoh T, Akira S, Araki E et al (2002) Targeted disruption of the chop gene delays endoplasmic reticulum stress-mediated diabetes. J Clin Invest 109:525–532
Roybal CN, Yang S, Sun CW, Hurtado D, Vander Jagt DL, Townes TM et al (2004) Homocysteine increases the expression of vascular endothelial growth factor by a mechanism involving endoplasmic reticulum stress and transcription factor ATF4. J Biol Chem 279:14844–14852
Acknowledgments
This research was supported by the National Nature Science Foundation, People’s Republic of China (grant no. 30672278 and 30872823).
Author information
Authors and Affiliations
Corresponding author
Rights and permissions
About this article
Cite this article
Li, B., Wang, H.S., Li, G.G. et al. The role of endoplasmic reticulum stress in the early stage of diabetic retinopathy. Acta Diabetol 48, 103–111 (2011). https://doi.org/10.1007/s00592-009-0170-z
Received:
Accepted:
Published:
Issue Date:
DOI: https://doi.org/10.1007/s00592-009-0170-z