Abstract
Tunisian microalgae are diverse and rarely been studied. This study reports a first investigation of thermophile Chlorophyta isolated from mats community colonizing the geothermal springs in the north of Tunisia at water temperature 60 °C. In the study, the combined effect of temperature and light intensity was investigated on the cell growth, the mother and daughter cells abundance and the extracellular polymeric substances synthesis in batch culture of the isolated species. Three levels were tested for each factor, 20, 30, 40 °C for temperature; and 20, 70, 120 μmol photons m−2 s−1 for light intensity, using full factorial design and response surface methodology. The thermophile strain was identified as a genus Graesiella and showed 99.8 % similarity with two Graesiella species: Graesiella emersonii and Graesiella vacuolata based on the 18S rDNA molecular identification. The optimal growth condition was found at 30 °C and 120 µmol photons m−2 s−1 (7 MC mL−1 day−1), with the abundance of vegetative cells (daughter cells). In contrast, the number of mother cells increased significantly as the growth decreased; consequently, the highest ratio of auto spore mother cells versus daughter cells (19.4) was obtained at 20 °C and 20 µmol photons m−2 s−1. The highest yield of EPS production (11.7 mg L−1 day−1) was recorded at the highest temperature (40 °C) and lowest light intensity (20 µmol photons m−2s−1). These results revealed how the species respond to high and low temperatures and suggest that the species should be considered as facultative thermophile.
Similar content being viewed by others
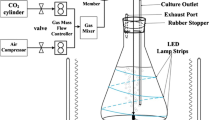
Avoid common mistakes on your manuscript.
Introduction
Hot spring mat communities have long attracted the attention of microbial ecologists because of the unique adaptation of the microbial flora under the influence of heat stress [1]. Their thermo tolerance makes them potential producers of high-value thermo stable bioactive compounds and providing a valuable resource not only for exploitation in novel biotechnological processes but also for investigating metabolic pathways implied in species adaptation to these harsh thermal environments. The thermal tolerance of some strict or facultative thermophilic microorganisms is due to the specific pathway of specific intra and extracellular polymers synthesis. The latter was ascribed to Extracellular Polymeric Substances (EPS). EPS are often thought to have a protective function including shielding cells from environmental extremes of temperature [2], desiccation [3], and pH fluctuation [4]. Over 99 % of microorganisms on the earth live within EPS [5]. Not only are these EPS thermo stable, they also have a wide range of bioactivities, including antioxidant [6] anti-inflammatory [7], antiviral [8], metabolic regulation [9] and antitumor activities [10].
To the best of our knowledge, and particularly in temperatures above 40 °C, prokaryotic strains (Cyanobacteria) are considered the most globally widespread in thermal springs [11]. Nevertheless, some eukaryotic microalgae such as unicellular Chlorophyta (green algae) can withstand these extreme temperatures [12]. Although, some thermo tolerant eukaryotic species have been isolated from hot springs, very few have been extensively studied to date [13]. As many species occurring in many hot springs worldwide not yet been identified and/or physiologically characterized, their potential is expected and desired in the biotechnological manufacturing of high-value biomolecules or deliberately enriched biomass [14]. The hot springs have proved ideal environments for the identification of novel microalgae [15]. The main disadvantage in using thermophiles as EPS producers is low biomass accumulation and correspondingly low EPS production. The main attention in thermophile processes is oriented to the optimization of physicochemical conditions for growth [16]. Light and temperature are effectively the two major processing factors that affect gross biomass productivity and metabolic pathways in phototrophic cultures [17].
Tunisian microalgae are diverse and numerous, however, they have rarely been studied. The present work reports a first investigation of thermophile eukaryotic species isolated from mats community of geothermal springs in the north of Tunisia, at water temperature 60 °C. The goal of this work is to favor the increasing of growth rate as much as possible or to push the metabolic route to follow the EPS synthesis direction across combinations of light and temperature factors. In this study, we first identified the species Graesiella. Secondly, we determined the life cycle growth (daughter and mother cells) by means of the size distribution using a deconvolution method. Thirdly, we evaluated the kinetics of growth rate, number of mother cells per daughter cells and EPS production in relation with controlled laboratory variation of light intensity and temperature. Finally, we examined by means of these later factors and their interaction on the optimization of the growth rate, mother cells ratio and maximum EPS productivity using full factorial design. The use of this experimental design methodology has been successfully applied to production of primary and secondary metabolites in different process culture [18].
Methods
Isolation and cultivation
Samples were taken from ‘Ain Echffa’, a hot spring located in the northern part of Tunisia (36°49′ N, 10°34′ E) at water temperature of 60 °C. Sampling materials were composed of microbial mats anchored to submerged stones. Mats collected were treated by filtration, centrifugation and dilution techniques according to standard microbiological protocols [19]. The purified strain was cultivated in batch culture under sterile conditions in Bold’s Basal Medium (BBM) [20] modified according to the Elser concept for freshwater microalgae with C:N:P ratio equal to 166:20:1 [21]. The initial pH was adjusted to the BBM pH (6.8) according to Bischoff and Bold [20]. The strain was acclimated to BBM before the experiment. Cultivation was carried out in photo bioreactors consisting of 1L sterilized flasks containing 500 mL medium and equipped with a device for aseptic removal of samples. Each culture was inoculated with an initial biomass concentration of 0.08 g L−1. Experiments were conducted under controlled light in a temperature-programmable chamber with continuous illumination. Phytoclaude halogen lamps (400 W) were used to illuminate the chamber. The intensity of incident light was measured using a silicon sensor HD 8366. All experimental cultures were exposed separately to different temperature (T) levels (20,30,40 °C) and light intensity (L) levels ranging from 20 to 120 µmol m−2 s−1, as shown in Table 1.
Nucleic acid extraction and 18S rDNA sequencing
Genomic DNA was extracted from the isolated strain using the hexadecyltrimethyl ammonium bromide (CATB) method described by Lefranc et al. [22]. The primers EukA (5′-AACCTGGTTGATCCTGCCAGT-3′) and EukB (5′ TGATCCTTCTGCAGGTTCACCTAC-3′) were used to amplify the 18S rRNA gene [23]. The PCR reaction was performed on a Thermocycler GeneAmp® PCR System 9700 (Applied Bio systems) in a 50 μL reaction mixture containing 0.2 mM each dNTP, 0.2 μM each primer, 50 ng DNA template, and 2.5 U TOP-Taq DNA polymerase (BIORON) with reaction buffer supplied by the manufacturer. The PCR program was as follows: denaturation for 3 min at 94 °C and subjected to 30 cycles for 45 s at 94 °C, 1 min at 55 °C and 2 min at 72 °C, followed by a final elongation step for 10 min at 72 °C. Reactions without template DNA were performed as negative controls. PCR products were purified and sequenced by society Biotools—Tunisia. The primers used for sequencing were the same as those used for amplification. Target sequences were analysed using BLAST online [ 24, 25]. Multiple alignments were generated with the MUSCLE program [26] and phylogenetic trees were constructed with MEGA program version 4 [27] on the basis of evolutionary distances that were calculated with a Neighbor-Joining method [28] with Maximum Composite Likelihood model. The bootstrap re-sampling analysis was performed for 1000 replicates to estimate degrees of confidence in tree topologies [29].
Cell concentration and cell size distribution
Cell concentration and cell size distribution were determined using a Coulter counter Multisizer III (Coulter corporation Miammi USA) with a 20 µm diameter capillary and a capacity to process 100 μL of the cell suspension in 0.9 % NaCl previously filtered through 0.2 μm. Before measurement, samples were fixed with Lugol’s solution and diluted to 1/50 with sterile seawater. The cell counts of each sample were determined in triplicate and the average was calculated. Calculation and graphical presentation were done with commercially available computer software (Multisizer III Biosoft, Excel Microsoft). Cell size distribution results [versus number of cells per milliliter (nb mL−1)] were typically expressed as a function of the equivalent spherical diameter (µm).
A 10 mL culture sample at exponentially growth phase was centrifuged (2,000 rpm for 5 min). The big cells (mother cells) settled to the bottom of the tubes, while small cells (daughter cells) remained in the supernatant solution. The two separated fractions were counted separately and the resulting matrices obtained were fitted with Gaussian distribution using ORIGIN software. The fitted solution matrices were then considered as representatives of daughter and mother cells distributions and used as reference cohorts for samples deconvolution.
The expression of each cell type in the sample can be modeled as a linear combination of the two discrete size classes representing reference cohorts of daughter and mother cells [30]. Each experimental sample matrix can be considered as a set of linear equation (Eq. 1):
In this case, B is the cell matrix distribution in the sample. X 1 and X 2 are the solution vectors which contain the unknown proportions of the reference cohorts in B. A 1 and A 2 are the known matrices of cell distribution of the mother and daughter cells. E represents the random measurement error in B. This matrix equation incorporates the reproduction cell type’s signature. It was solved using standard linear least-squares fitting for X 1 and X 2, which are the relative amounts of the underlying reference cohorts in the mixture of interest B. The resulting modes in each sample were carefully tested using correlation-coefficient and Student test. When cohorts and modes could not be assigned unambiguously (R 2 < 0.5 or T test non-significant P > 5 %), the cell distribution was counted, however, it was not considered in the analysis of growth parameters.
EPS determination
EPS concentration was determined using the method described in Trabelsi et al. [18]. Briefly, samples (50 mL) were taken daily from each condition of each experimental culture, and being centrifuged (4,000 rpm, 10 min, at 4 °C) to separate the cells from the culture medium. The resulting culture filtrate containing the culture medium and released EPS. The EPS was concentrated by a tangential ultra-filtration cell (Millipore, Bedford. MA) in Millipore membranes with a 30 kDa pore size, and then washed three times with deionized water to eliminate low molecular weight substances. Lastly, the EPS was freeze-dried and lyophilized.
Experimental design
Full factorial design was utilized to evaluate the effects of two factors (i.e., temperature and light intensity) on the Maximum Growth Rate (MGR), the ratio of mother cells versus daughter cells [Mother Proportion (MP)] and the Maximum EPS Productivity (MPEPS). The increase, or decay in cell number into two populations, was used as an index of growth in this work.
The max growth rate (MGR) and the max EPS productivity (MPEPS) were calculated through the derivate form of the growth rate (GR) (Eq. 2) and the EPS synthesis (PEPS) (Eq. 3) curves, calculated from the daily, growth and EPS data using the following formula:
where x is the total number of cells per milliliter and t represents the time. The unit of GR is in number of cells per milliliter per day (nb cells mL−1 day−1) and the unit of PEPS is in milligram per liter per day (mg EPS mL−1 day−1).
MP response was based on the cohort deconvolution results. It represents the maximum value of mother cells per daughter cell obtained during the course time of each experimental culture.
The two factors (i.e., temperature and light intensity) were examined at three levels 20, 30 and 40 °C of temperature and 20, 70 and 120 µmol photons m−2 s−1 of light intensity (Table 1).
The design contained 12 experiments with nine unique combinations and three replications at the central point to evaluate the pure error.
A response surface modeling (RSM) is a detailed modeling and optimization using quadratic and cubic models. A second order poly-nominal model has been defined to fit the responses (Eq. 4):
where Y is the response, β 0 is the constant coefficient, β i is linear coefficient, β ii is the quadratic coefficient, β ij is the second order interaction coefficient, and e is the random error. Analyses of the results and the effect of each factor and their interactions were performed with MODDE.7®. The statistical significance of these two factors was determined by the analysis of variance at 90 % confidence level.
Results
Strain identification
The 18S rDNA from the isolate was sequenced using the primer pair EukA and EukB (1677 bases pair). The phylogenetic tree based on the sequence of 18S rDNA can be seen in Supplementary Fig. S1. Phylogenetic analysis revealed that the isolated strain was closely related to the genus Graesiella and showed 99.8 % similarity with two Graesiella species: Graesiella emersonii [31], synonym: Chlorella emersonii [32] and Graesiella vacuolata [31], synonym: Chlorella emersonii var.globosa [32]. It belongs to the Chlorophyceae class and it is grouped within the Chlamydomonadales order.
Cell counting and deconvolution
The reference cohorts of daughter and mother cells were plotted separately as number of cells per mL versus diameter (Fig. 1). Comparison of five independent experiments used for estimation of each cell type distribution did not show significant differences (mean of ±2.3 %). Therefore, the values obtained for these cohorts were presented as the mean of 5 runs in total. Both distributions were well fitted by Gaussian functions (R 2 > 0.9) and showed mode diameters of 4.16 µm for daughter cells and 5.79 µm for mother cells with width values equal to 1.46 ± 0.036 and 2.07 ± 7.7 E-11, respectively.
All mixed sample distributions were deconvoluted by considering their maxima and their positions relative to the reference cohorts already identified as shown in Fig. 2.
Kinetics
Given the dynamic behavior of the cell growth and cell composition under these culture conditions, day 5 was chosen as the end point of batch culture for the purpose of analysis. For days 6 to 15, cohorts and modes could not be assigned unambiguously (data not shown), most likely caused by cell lysis observed in most of the experimental runs.
The kinetics of cell growth, number of mother cells divided per daughter cells and EPS productivity of the 12 individual runs showed relatively wide differences under different conditions (data not shown). For instance, the time courses of these responses are shown for cultures grown at three different combinations of temperature and light intensity (Fig. 3).
The most pronounced curve of growth rates (Fig. 3a) was at T30 °C and L120 µmol photons m−2 s−1. The maximum growth rate was then estimated to be 7 MC mL−1 day−1. It was less pronounced when the temperature and light were reduced T20 °C and L20 µmol photons m−2 s−1 (1.65 MC mL−1 day−1). Under extreme conditions, particularly under extreme temperatures (T40 L20), the growth curve deviated considerably from the exponential shape and the growth rate was strongly decreased (0.05 MC mL−1 day−1).
The proportion of mother cells versus daughter cells (Fig. 3b) varied inversely to the growth rate as determined by the mean of temperature and light intensity. At maximal growth rate conditions (T30 L120), the number of mother cells was 50 % less than the daughter cells particularly at the steady state phase of growth. On the contrary, at low and high temperatures, the number of mother cells was 7 and 4-fold, respectively, higher then daughter ones.
Similarly, EPS production showed a relatively wide variation under different conditions (Fig. 3c). The highest EPS production was recorded at high temperature (40 °C). The lowest EPS concentration was found at low temperature (20 °C).
By comparing the EPS productivity kinetics to growth rate time course, it was clear that for the cultures grown at 40 °C and 20 μmol photons m−2 s−1, the whole metabolic pathway was shifted to produce EPS. Indeed, the growth rate was null after 2 days of cultivation but the EPS rate showed the highest value (11.5 mg L−1 day−1 at day 4). At 20 °C, the growth rate was less inhibited and the EPS production showed the lowest value (0.5 mg L−1 day−1 on day 3).
Model accuracy
The influence of temperature and light intensity on MGR, MP and MPEPS was investigated, using response surface methodology (RSM). The results are summarized in Table 2.
Analysis of variance for the three modulated processes indicated that all the responses can be well described by the polynomial models (Table 2). For the three models, there was no lack-of-fit (P < 0.05) and there was a satisfactory coefficient of determination (R 2 = 0.98).
The statistical analysis showed that the two factors (temperature and light intensity) had significant effects on the three responses. The P values for MGR coefficients indicate that only quadratic effect of light intensity (L 2) was no significant ( P > 0.05). For MP coefficients, only interactive effect of temperature and light intensity (TL) was no significant while for MPEPS all terms of the model showed significant effects.
After elimination of no significant terms, the unscaled coefficients of the three models can be expressed as follows (Eqs. 5, 6, 7):
The relationships between the reaction factors and responses could be best understood when examining the isoresponse surfaces. The isoresponse representations are generated by plotting contours of the responses versus the two factors.
As shown in Fig. 4a, the growth rate was strongly dependent on the temperature. Light levels had less effect. Optimal growth can be obtained in the temperature range 27–30 °C and at high level of light intensity (120 µmol photons m−2 s−1). At low and high temperature levels, predicted growth rate strongly decreased from 7.66 to 1.23 and 0.09, respectively.
In contrast, the number of mother cells (Fig. 4b) was strongly increased as the growth decreased. Maximum proportion of MP was obtained at 20 °C (20-fold greater than daughter cells) and also at 40 °C (7-fold greater than daughter cells). This proportion was inferior to the number of daughter cells when growth was optimal. The effect of light intensity was less pronounced, and when this factor increased from minimal to maximal values, the variation of MP value did not exceed 8 %.
An increase in EPS production was mainly achieved by raising the temperature (Fig. 4c). The model predicted the maximum EPS productivity of 11.7 mg L−1 day−1 that appeared at 40 °C of temperature and at light intensity 20 µmol photon m−2 s−1. The lowest EPS values (1 mg L−1 day−1) were observed at low temperature levels (20 °C) independent of light intensity.
Discussion
In this work, the strain was isolated from the Tunisian hot spring at 60 °C water temperature. Based on 18S rRNA data, it was affiliated to the genus Graesiella. Nevertheless, the differing taxonomic assignments to two Grasiella species Graesiella emersonii and Graesiella vacuolata suggest that further investigations are needed for accurate identification. Indeed, although the vast majority of the data available is currently represented by 18S rRNA gene sequences, the substitution rate of this gene is usually not sufficient to clarify relationships at genus and species level. For this purpose, the use of other, more variable molecular markers is necessary [33].
To the best of our knowledge, the genus Graesiella is rarely signaled in hot springs worldwide and particularly at temperatures above 40 °C. It is evident that the patterns of geographical distribution of green algae in general are still poorly understood, mainly because the diversity of these organisms itself is roughly assumed.
Otherwise, both Graesiella emersonii and Graesiella vacuolata were affiliated to the genus Chlorella (as C. emersonii and C.emersonii var.Globosa) and then recently transferred to the genus Graesiella [31]. The taxonomy of the genus Chlorella is being gradually dismantled and subdivided into several separate genera [34]. It is likely that detailed surveys conducted in other hot springs will reveal a wider geographical distribution for many species of the genus Graesiella.
Current results show that the growth rate was strongly dependent on the temperature. An optimal growth condition was obtained at 30 °C. The growth rate decreased dramatically in extreme conditions at lowest temperatures (20 °C) and especially at highest temperatures (40 °C). This is consistent with previous studies of thermophilic Chlorophyta species in laboratory culture (C. emersonii, C. saipanensis, Klebsormidium sp. and Hantzschia sp.) which showed that all behaved similarly and grew better at 30 °C with a preference to lower temperatures [35].
It has to be emphasized that, in this case, as in all experimentally controlled laboratory cultures, lethal temperatures did not exceed 40 °C (result not shown) which is much lower than that measured in the hot spring (60 °C).This trend was observed in many of the thermophilic algae species investigated in laboratory culture [36]. In fact, in field conditions, a mixture of physiologically different groups of microorganisms (e.g., filamentous and chroococcales Cyanobacteria, Bacillariophyta, Chlorophyta) are agglomerated, with mineral deposits, and organized in multi layers into dense mats. The complex interrelation between different species in the mat community (symbiosis, mutualism and commensalism) could explain the enhanced ability of some moderate thermophilic species (including Graesiella) to adapt to these harsh ecologic conditions by avoiding direct contact with the highest water temperature. However, this could not be reproduced in laboratory cultures. Indeed, since the optimal growth rate (30 °C) was recorded at lower temperature than in field conditions, this strain could be considered as facultative thermophile (also called moderate thermophile) according to the definition of Walsh and Seckbach [37]. Apart from temperature, the effect of light intensity on growth rate was also significant but with only linear effect which means that the growth rate could be further enhanced by increasing light intensity over 120 µmol photons m−2 s−1. Furthermore, the impact of the interaction between light intensity and temperature was identified. This interaction plays an important role and should be considered when optimizing micro algal growth as highlighted by several authors [38, 39]. The effect of temperature and light intensity also affected the life cycle of this strain in the same way.
Optimal growth conditions led vegetative cells to differentiate from auto spore mother cells, consequently the decrease of auto spore mother cells percentage [40]. In contrast, under stressful conditions, the ratio of auto spore mother cells to vegetative cells increased. The latter was probably caused by the blockage or failure of auto spore mother cells to dehisce [40]. Similar results have been reported in previous studies for Chlorella species under different stress factors [41, 42].
For EPS production, a dominant effect of temperature was mainly observed since the effect of light intensity was weakly present at low temperature levels, whereas, it was quasi-absent at high temperature values. As far as we know, no data are available concerning the effect of temperature on EPS synthesis by Graesiella strains. However, such effects have been studied for other microalgae [2, 18]. Such studies have shown similar behavior to that observed in this study. Furthermore, the current results also revealed that the optimal conditions for EPS production differ from those for optimal growth. EPS production is maximal at 40 °C, at which growth rate strongly decreases. The capacity to sustain the large fluxes of carbon and energy required for rapid EPS production appears to be inversely related to the growth efficiency of microorganisms [43]. It was established that, when cells growth was inhibited, phototrophic cells involve a drain of extra energy to synthesis of the EPS as a view to avoid oxidative damage. In addition, it is widely believed that the synthesis of EPS plays a major role in protecting cells from various stresses in severe habitats, although the experimental evidence for the functions of EPS in green algae is limited [44]. Moreover, in the hot spring conditions, the adhesive propriety of EPS demonstrated by many authors improved the ability of species to attach firmly to their solid substratum which is essential to resist against high flow rates commonly observed in hot springs [45]. Nevertheless, at suboptimal temperature, the growth decreased with the increase of mother cell proportion and the decrease of EPS synthesis. This result suggests that the mechanisms that control the destiny of fixed carbon between internal and external metabolic pools [44] are mainly stimulated at high temperature above optimal growth conditions. Also, these results revealed that at the lowest suboptimal temperature, intracellular stress metabolic pathways should be implied. Indeed, irrespective of any other effect, Smith-Bädorf et al. [35] showed that for the thermophilic Chlorophyta species, temperature reduction at 20 °C resulted in recovery of a larger quantity of methyl fatty acids.
Conclusions
In conclusion, the thermophilic Chlorophyta strain isolated from the hot spring was related to the genus Graesiella. This strain revealed high adaptive capacities against temperature and light intensity variations. The optimal growth temperature was recorded at 30 °C, which was lower than field temperature condition. This strain can adapt to high temperature by enhancing EPS synthesis as a protector metabolic pathway. At low temperatures, the dehisce of auto spore mother cells was blocked, but EPS synthesis was at its lowest level, which suggests an intracellular adaptation pathway mechanism that requires further investigation.
References
Zakaria AM, Abdulrahman MA (2007) Cyanobacteria and their toxins in treated-water storage reservoirs in Abha city, Saudi Arabia. Toxicon 50:75–84
Wolfstein K, Stal LJ (2002) Production of extracellular polymeric substances (EPS) by benthic diatoms: effect of irradiance and temperature. Mar Ecol Prog Ser 236:13–22
McKew JD, Taylor TJ, Mcgenity, Underwood GJC (2011) Resilience of benthic biofilm communities from a temperate salt marsh to desiccation and rewetting. ISME J 5:30–41
Thornton DCO (2009) Effect of low pH on carbohydrate production by a marine planktonic diatom (Chaetoceros muelleri). Res. Lett. in Ecol. 2009, Article ID 105901 doi:10.1155/2009/105901
Vu B, Chen M, Russell JC, Ivanova EP (2009) Bacterial extracellular polysaccharides involved in biofilm formation. Molecules 14:2535–2554
Chen LQ, Hou BH, Lalonde S, Takanaga H, Hartung ML, Qu XQ, Guo WJ, Kim JG, Underwood W, Chaudhuri B, Chermak D, Antony G, White FF, Somerville SC, Mudgett MB, Frommer WB (2010) Sugar transporters for intercellular exchange and nutrition of pathogens. Nature 468:527–532
Guzmán S, Gato A, Lamela M, Freire-Garabal M, Calleja JM (2003) Anti-inflammatory and immunomodulatory activities of polysaccharide from Chlorella stigmatophora and Phaeodactylum tricornutum. Phytother Res 17:665–670
Zheng W, Chen C, Cheng Q, Wang Y, Chu C (2006) Oral administration of exopolysaccharide from Aphanothece halophytica (Chroococcales) significantly inhibits influenza virus (H1N1)-induced pneumonia in mice. Int Immunopharmacol 6:1093–1099
Dvir I, Chayoth R, Sod-Moriah U, Shany S, Nyska A, Stark AH (2000) Soluble polysaccharide and biomass of red micro algae Porphyridium sp. alter intestinal morphology and reduce serum cholesterol in rats. Br J Nutr 84:469–476
Gardeva E, Toshkova R, Minkova K, Gigova L (2009) Cancer protective action of polysaccharide derived from micro algae Porphyridium cruentum—a biological background. Biotechnol Equip 23:783–787
Seckbach J (2007) Algae and Cyanobacteria in extreme environments. Springer, Dordrecht
Jonker CZ, Van Ginkel C, Olivier J (2013) Association between physical and geochemical characteristics of thermal springs and algal diversity in Limpopo Province, South Africa. Water S.A 39: 95−104
Ratha SK, Prasanna R (2012) Bioprospecting microalgae as potential sources of ‘green energy’, challenges and perspectives. Appl Biochem Microbiol 48:109–125
Guedes AC, Amaro HM, Malcata FX (2011) Microalgae as sources of high added-value compounds—a brief review of recent work. Biotechnol Prog 27:597–613
Mutanda T, Ramesh D, Karthikeyan S, Kumari S, Anandraj A, Bux F (2011) Bioprospecting for hyper-lipid producing micro algal strains for sustainable biofuel production. Bioresource Technol 102:57–70
Radchenkova N, Tomova A, Kambourova M (2011) Biosynthesis of an exopolysaccharide produced by Brevibacillus thermoruber 438. Biotechnol Biotechnol Equip 25:77–79
Carvalho AP, Malcata FX (2003) Kinetic modeling of the autotrophic growth of Pavlova lutheri: study of the combined influence of light and temperature. Biotechnol Prog 19:1128–1135
Trabelsi L, Ben Ouada H, Bacha H, Ghoul M (2009) Combined effect of temperature and light intensity on growth and extracellular polymeric substance production by the Cyanobacterium Arthrospira platensis. Appl Phycol 21:405–412
Guillard RRL (2005) Purification methods for microalgae. In: Andersen RA (ed) Algae culturing techniques. Academic Press, Elsevier Science, San Diego
Bischoff HW, Bold HC (1963) Phycological studies IV. some soil algae from enchanted rock and related algal species. University of Texas Publication 6318:1–95
Elser JJ, Sterner RW, Gorokhova E, Fagan WF, Markow TA, Cotner JB, Harrison JF, Hobbie SE, Odell GM, Weider LJ (2000) Biological stoichiometry from genes to ecosystems. Ecol Lett 3:540–550
Lefranc MP, Clément O, Kaas Q, Duprat E, Chastellan P, Coelho I, Combres K, Ginestoux C, Giudicelli V, Chaume D, Lefranc G (2005) IMGT-Choreography for immunogenetics and immunoinformatics. In Silico Biology 5:45–60
Medlin L, Elwood HJ, Stickel S, Sogin ML (1988) The characterization of enzymatically amplified eukaryotic 16S-like rRNA-coding regions. Gene 71:491–499
Altschul SF, Madden TL, Schaffer AA, Zhang J, Zhang Z, Miller W, Lipman DJ (1997) Gapped BLAST and PSI-BLAST: a new generation of protein database search programs. Nucl. Acids Res 25:3389–3402
Edgar RC (2004) Local homology recognition and distance measures in linear time using compressed amino acid alphabets. Nucl. Acids Res 32:380–385
Tamura K, Dudley J, Nei M, Kumar S (2007) MEGA4: Molecular Evolutionary Genetics Analysis (MEGA) software version 4.0. Mol Biol Evol 24:1596–1599
Saitou N, Nei M (1987) The neighbor-joining method: a new method for reconstructing phylogenetic trees. Mol Biol Evol 4:406–425
Felsenstein J (1985) Confidence limits on phylogenies: an approach using the bootstrap. Evolution 39:783–791
Abbas AR, Wolslegel K, Seshasayee D, Modrusan Z, Clark HF (2009) Deconvolution of blood microarray data identifies cellular activation patterns in systemic Lupus Erythematosus. PLoS one 4:1–16
Nozaki H, Katagiri M, Nakagawa M, Aizawa K, Watanabe MM (1995) Taxonomic re-examination of two strains labeled ‘Chlorella’ in the microbial culture collection at the National Institute for Environmental Studies (NIES-Collection). Microbial Culture Collections 11:11–18
Shihira I, Krauss RW (1965) Chlorella. Physiology and taxonomy of forty-one isolates. University of Maryland, College Park, Maryland, pp 1–97
Rindi F, Lam DW, López-Bautista JM (2009) Phylogenetic relationships and species circumscription in Trentepohlia and Printzina (Trentepohliales, Chlorophyta). Mol Phylogenet Evol 52:329–339
Zhang JM, Huss VAR, Sun XP, Chang KJ, Pang DB (2008) Morphology and phylogenetic position of a trebouxiophycean green alga (Chlorophyta) growing on the rubber tree, Hevea brasiliensis, with the description of a new genus and species. Eur J Phycol 43:185–193
Smith-Bädorf HD, Chuck CJ, Mokebo KR, MacDonald H, Davidson MG, Scott RJ (2013) Bioprospecting the thermal waters of the Roman baths : isolation of oleaginous species and analysis of the FAME profile for biodiesel production. AMB Express 3:9–14
Jackson JEJ, Castenholz RW (1975) Fidelity of thermophilic blue-green algae to hot spring habitats. Limnol Oceanogr 20:305–322
Walsh MM, Seckbach J (1999) The versatility of microorganisms. In: Seckbach J (ed) Enigmatic microorganisms and life in extreme environments. Kluwer Academic Publishers, Dordrecht, The Netherlands, pp 153–162
Sorokin C, Krauss RW (1962) Effects of temperature & illuminance on Chlorella growth uncoupled from cell division plant physiol 37:37–42
AVonshak G Torzillo (2004) Environmental stress physiology. In: Richmond A (ed) Microalgal culture: biotechnology and applied phycology. Blackwell Science, pp 5782
Agrawal SC, Manisha (2007) Growth, survival and reproduction in Chlorella vulgaris and C.variegata with respect to culture age and under different chemical factors. Folia Microbiol 52:399–406
Guckert JB, Cooksey KE (1990) A Triglyceride accumulation and fatty aid profile changes in Chlorella (Chlorophyta) during high pH- induced cell cycle inhibition. J Phycol 26:72–79
Rioboo C, O’Connor JE, Prado R, Herrero C, Cid A (2009) Cell proliferation alterations in Chlorella cells under stress conditions. Aquat Toxicol 94:229–237
Linton JD (1991) Metabolite production and growth efficiency. Antonie Van Leeuwenhoek 60:293–311
Otero A, Vincenzini M (2003) Extracellular polysaccharide synthesis by Nostoc strains as affected by N source and light intensity. J Biotechnol 102:143–152
Noffke N, Gerdes G, Klenke T (2003) Benthic cyanobacteria and their influence on the sedimentary dynamics of peritidal depositional systems (siliciclastic, evaporitic salty, and evaporitic carbonatic). Earth-Sciences Reviews 62:163–176
Acknowledgments
The authors would like to give special thanks to Prof. Sami Sayadi (Head of Laboratory of Environmental Bioprocesses) and Dr. Fatma Karray from the Center of Biotechnology of Sfax of Tunisia for their helpful assistance and their collaboration. Also, the authors express their gratitude to Dr. Jun Zhao (Post-doctoral researcher) and Mrs. Haifa Ben Romdhane (Research assistant) at Masdar Institute of Science and Technology, Abu Dhabi, UAE for their critical review of the manuscript.
Author information
Authors and Affiliations
Corresponding author
Electronic supplementary material
Below is the link to the electronic supplementary material.
449_2014_1204_MOESM1_ESM.tif
Fig. S1 Phylogenetic tree based on the sequence of 18S rDNA (Bootstrap values are given at the nodes, Scale bar represents the substitution percentage, GenBank accession numbers follow species name in parenthesis) (TIFF 11,137 kb)
Rights and permissions
About this article
Cite this article
Mezhoud, N., Zili, F., Bouzidi, N. et al. The effects of temperature and light intensity on growth, reproduction and EPS synthesis of a thermophilic strain related to the genus Graesiella . Bioprocess Biosyst Eng 37, 2271–2280 (2014). https://doi.org/10.1007/s00449-014-1204-7
Received:
Accepted:
Published:
Issue Date:
DOI: https://doi.org/10.1007/s00449-014-1204-7