Abstract
The degree to which behaviour, vertical movement and horizontal transport, in relation to local hydrodynamics, may facilitate secondary dispersal in the water column was studied in post-larval Sillaginodes punctata in Port Phillip Bay, Australia. S. punctata were captured in shallow seagrass beds and released at the surface in three depth zones (1.5, 3 and 7 m) off-shore at each of two sites to mimic the re-entrainment of fish. The behaviour, depth and position of S. punctata were recorded through time. The direction and speed of local currents were described using an S4 current meter and the movement of drogues. Regardless of site, fish immediately oriented toward the bottom, and into the current after release. In shallow water (1.5 m), 86% of fish swam to the bottom within 2 min of release. At one site, the net horizontal displacement of fish was largely unrelated to the speed and direction of local currents; at a second site, fish could not maintain their position against the current, and the net horizontal displacement was related to the speed and direction of currents. In the intermediate depth zone, wide variability in depths of individual fish through time led to an average depth reached by fish that was between the shallow and deep zones. Based on daily increments in the otoliths, however, this variability was not related significantly to the time since entry of fish into Port Phillip Bay. In the deepest depth zone, 81% of fish remained within 1 m of the surface and their horizontal displacement was significantly related to the direction and speed of currents. Secondary dispersal of post-larval fish in the water column may be facilitated by the behaviour and vertical movements of fish, but only if fish reach deeper water, where their displacement (direction and distance) closely resembles local hydrodynamic regimes. In shallow water, fish behaviour and vertical migration actually reduce the potential for secondary dispersal.
Similar content being viewed by others
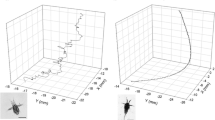
Avoid common mistakes on your manuscript.
Introduction
By the time they settle, the individuals of most species of marine fish have survived a pelagic phase, the search for a settlement site, and radical transitions in morphology and environment (Leis 1991). Settlement-stage larval processes, including predation, disturbance, food availability and competition, influence recruitment patterns in juvenile fish soon after settlement (Doherty and Williams 1988; Jones 1991; Caley et al. 1996; Hixon 1998). Planktonic dispersal and larval supply is widely implicated in shaping patterns in recruitment at the settlement stage (Milicich et al. 1992; Jenkins et al. 1998), but, until fairly recently, secondary dispersal in the pelagic environment has been largely ignored. Secondary dispersal is the process whereby settlement-stage larval fish are re-entrained into the water column as a result of environmental disturbance or behaviour, and transported to another location by currents.
Drifting by post-settlement macrofauna is considered to be an important dispersal mechanism (Cummings et al. 1995), and initial recruitment patterns of invertebrates can be altered considerably by the re-entrainment and dispersal of post-larvae (Christy and Morgan 1998). Episodic disturbance events are one mechanism by which settled individuals can be re-entrained. Etherington and Eggleston (2000) found that post-settlement juvenile Callinectes sapidus were probably dispersed by storm-driven transport. Secondary dispersal in the pelagic environment has been little studied in fish, however, and whether or not it influences settlement patterns in temperate fish larvae will depend on how early settlement-stage fishes respond to re-entrainment.
Fish larvae have often been modelled as passive particles when simulating dispersal patterns and recruitment dynamics (Heath et al. 1998; Proctor et al. 1998; Fowler et al. 2000; Jenkins et al. 2000), often resulting in accurate predictions of actual dispersal. Studies in tropical waters, however, have shown that the transport of late-stage larval reef fish is far from passive (Leis et al. 1996; Leis and Carson-Ewart 1999). They are competent swimmers, able to actively modify their dispersal (Stobutzki and Bellwood 1997), and some tropical reef fish larvae are capable of high swimming speeds, up to 30 cm s-1 (Leis et al. 1996), and prolonged swimming activity (Stobutzki and Bellwood 1997). They appear to have taxon-specific depth preferences (Leis et al. 1996) that can vary between locations (Leis and Carson-Ewart 2000) and diel cycles (Leis 1986), and several species show highly non-random orientation while swimming (Leis et al. 1996; Leis and Carson-Ewart 1999). Attributes of larval fish behaviour such as swimming ability are increasingly incorporated in mathematical models to improve the predictability of dispersal-recruitment relationships (Armsworth 2000). Much of this research has been restricted to tropical reef fishes, although recent work by Dudley et al. (2000) has found that several species of reef fish in temperate New Zealand waters were strong swimmers—individuals of Scorpis lineolatus (Scorpidae) swam for up to 559 h (271 km). These studies suggest that settlement-stage and small juvenile fish should not be treated as passive particles. Conversely, Jenkins and Welsford (2002) found that the weak swimming ability of post-larval King George whiting (Sillaginidae: Sillaginodes punctata, Cuvier) is, despite some vertical migration, consistent with passive dispersal driving early recruitment patterns (Jenkins et al. 1997, 1999). Much is still to be learnt about the potential for behaviour and swimming ability of temperate fishes to alter recruitment patterns.
Post-larvae of S. punctata enter Port Phillip Bay each spring (Jenkins 1986). Fish range in length from 17 to 20 mm, and are between 80 and 150 days of age (Jenkins and May 1994; Jenkins et al. 1996). We define post-larvae as late-stage larvae with a full compliment of fin elements, but without the darker pigmentation, gut coiling and scale formation seen in early juvenile fish (Bruce 1995). Fish enter the bay in pulses, which are correlated with low pressure systems and strong westerly winds causing a net flow of oceanic water into the bay (Jenkins and Black 1994; Jenkins et al. 1997). Fish then settle into shallow reef/algal and seagrass habitats (Jenkins and Wheatley 1998). At this size, compared with coral reef fishes, post-larvae are relatively weak swimmers (Jenkins and Welsford 2002). Sites closest to the entrance of Port Phillip Bay initially "collect" relatively large numbers of post-larval S. punctata. Through time, however, abundances of fish decrease, until, towards the end of spring, there are high numbers of fish well inside Port Phillip Bay and very few at the sites closer to the heads (Jenkins et al. 1996; Jenkins and Wheatley 1998). Oceanographic modelling of the bay suggests that around 70% of the variability in the abundances of fish between sites through time can be explained by currents and local disturbance regimes, such as those caused by wave action as a result of on-shore winds (Jenkins et al. 1997, 1999). Fish abundance is best explained in the southern half of the bay, where tidal currents dominate, but further inside the bay, predictions are less accurate, and re-suspension of fish during on-shore winds, followed by further dispersal, has been suggested as a mechanism that might explain this disparity (Jenkins et al. 1999). Secondary dispersal in the water column may, therefore, influence recruitment patterns of S. punctata.
For secondary dispersal to be important, fish must actually be re-entrained, and fish behaviour must allow or facilitate dispersal. Recent research has supported the first requirement. During on-shore winds, the numbers of post-larval S. punctata are relatively greater in the plankton than in the seagrass beds, the isotopic signatures of some fish in the plankton are consistent with seagrass as a base for nutritional support, and 70% of fish placed in cages within patches of seagrass are re-caught on the seaward side of the enclosures (S. Moran, in preparation). Alternatively, in offshore or light winds, the numbers of fish are relatively higher in the seagrass than they are in the plankton, no fish caught in the plankton have isotopic signatures consistent with seagrass, and fish enclosed in cages within patches of seagrass are evenly distributed around the sides of the enclosures (S. Moran, in preparation). Here, we determine whether the behaviour, vertical movement and horizontal transport of fish in relation to local currents facilitates the secondary dispersal of post-larval S. punctata.
In this study, we re-entrained post-larvae of S. punctata in three different depth zones and followed them on SCUBA. We measured their vertical position in the water column through time to assess whether fish remained on the surface or swam towards the bottom. We also measured their net horizontal displacement (both direction and distance), and compared this to predictions from currents (as estimated by the movement of drogues and predicted by a S4 current meter). This is the first study to observe the behaviour of, and measure the movement by, temperate post-larval fish re-entrained in the water column, and provides an opportunity to assess the potential for secondary dispersal to modify recruitment patterns.
Materials and methods
Study sites
Our research was done at two sites on the western coast of Port Phillip Bay: Indented Heads and Grassy Point (Fig. 1). During spring, large numbers of post-larval Sillaginodes punctata recruit to shallow (0.5 m below MLWS) seagrass beds that form bands parallel to the shoreline in the vicinity of each site. Heterozostera tasmanica (Zosteraceae) den Hartog grows over the subtidal banks, and Zostera muelleri (Zosteraceae) Irmish ex Ascherson grows predominantly in the intertidal. Three main depth profiles offshore from the beds of seagrass at Grassy Point were chosen. The shallow and intermediate zones had depths of 1.5 and 3 m respectively (below MLWS). The deep zone had a maximum depth of 7 m (below MLWS). The substratum offshore at Grassy Point is composed of a mosaic of patches of reef/macroalgae with smaller patches of unvegetated sand. The depth of water at Indented Heads did not exceed 3.5 m directly offshore from the beds of seagrass, so our study was restricted to the shallow and intermediate depth zones at this site. The substratum at Indented Heads was unvegetated sand.
Collection of fish
Fish were caught in shallow seagrass beds at Grassy Point with a beach seine net (10 m long × 2 m high with 1 mm mesh). The net was set parallel to the shoreline so that each of two, 10 m long ropes attached to the sides of the net were fully extended. The net was then hauled gently into a large bucket, and the fish were collected and placed, alive, in a container filled with aerated seawater.
SCUBA "follows"
Fish were followed by a single diver to avoid excessive disturbance caused by diver movements. Each fish was released from a jar 50 cm below the surface of the water. Divers oriented in a variety of directions to release fish to ensure that their release point was not always in the same direction with reference to the shore-line or the local currents. On release, the diver raised the empty jar above his head to notify the boat crew to begin the follow period. The diver then followed the fish at a distance of between 1.5 and 3 m, depending on the visibility, for 10 min. Fish which showed behaviour indicative of ill health or injury were abandoned.
Six fish were released at each of two depth zones within a site on a particular sampling day (replicate). Releases were undertaken on 5 different days in each depth zone at Grassy Point, and on 3 different days in each depth zone at Indented Heads. Water at Indented Heads was too shallow to allow a depth zone equivalent to the deep zone at Grassy Point. Fish were re-caught at the end of each follow so that the influence of their size [standard length (SL) in mm] and time since entering Port Phillip Bay on behaviour and swimming ability could be assessed.
At 1-min intervals after (and including) the time of release, the horizontal position of the diver was recorded with a GARMIN geographical positioning system fitted with a differential beacon (±1 m). The DGPS aerial, which was fixed to an arm extending 1.5 m off the side of the boat, was positioned over a buoy that was attached to the diver by a 7-m-long rope. By measuring the position of the buoy, and therefore the relative position of the diver, we were able to minimise the effects of any boat-related disturbance to the fish and the diver. The horizontal direction and displacement of the fish were calculated in ArcView.
The depth (vertical) profile of each fish was logged automatically with a wrist-mounted dive computer at 20-s intervals (Mosquito, SUUNTO). The diver ensured that their wrist was at approximately the same depth as the fish. Depths less than 1.2 m were recorded as 'surface' intervals by the computer, but the diver informed the crew of the vertical position of fish within this layer at end of the dive.
At the beginning of the last fish-follow in each depth zone on a particular sampling day, two drogues were released. One drogue sat just below the surface of the water (shallow drogue); a second drogue was set at a depth of 1.5 m (deep drogue). Drogues were made from 2 mm galvanised wire covered with thin polythene wrapping. Each drogue was arranged in the shape of a cross, with four square panels (0.3×0.3 m) set at 90° to one another. Drogues were slightly negatively buoyant, but were 'hung' in the water column from a small float with 1 kg monofilament fishing line. The floats also enabled drogues to be located after deployment. An InterOcean Systems S4-AHDW current meter (hereafter referred to as S4) was deployed 1 m above the substratum on a stainless steel frame in each depth zone while fish were being followed to record current speed (cm s-1) and direction (degrees magnetic). If gross water movement was an accurate predictor of fish movement, we predicted that the displacement of fish would be similar to that estimated from current directions and speed. The S4 was also the point around which all fish were released. The predicted displacement of a passive particle was calculated by multiplying the current speed measured by the S4 with the time of a fish-follow (10 min).
Otolith analysis
In many recruits, otoliths contain a transition in microstructure, signified by a rapid increase in increment width, that occurs at approximately the time of entry to Port Phillip Bay (Jenkins and May 1994). The number of days post this transition mark was measured in fish from the intermediate depth zone to assess whether the time spent in Port Phillip Bay influenced their behaviour; fish from the intermediate depth zone had the most varied swimming depths. Otoliths were dissected from fish and examined under a compound microscope with attached video system using the techniques described by Jenkins and May (1994). The number of increments 'outside' the transition was counted to provide an estimate of the time period since the post-larvae entered the bay. Increment counts were done twice, the second reading blind with respect to the result of the first. Both readings were done blind with respect to the fish. If there was a discrepancy of more than two increments, a third reading was done. If all three readings differed as described, the otolith was rejected; if two of the counts were within the specified range, then the average of those two counts was used.
Statistical analysis
All data used in one- and two-factor analyses of variance (ANOVA) were checked for normality and homogeneity of variances using box plots and plots of residuals. Data used in analysis of covariance (ANCOVA) were tested to ensure homogeneity of slopes. Data that failed to meet these criteria were transformed and reassessed. Tukey's tests were used to assess where differences between main effects existed. In all analyses of variance, replicates are at the level of day, and follows of fish within a day are averaged.
The variation in vertical position (depth profile) of S. punctata between depth zones at Grassy Point was initially analysed with a two-factor repeated measures ANOVA. Depth (of release) and time (minutes after release) were treated as fixed factors. In a second analysis, site (random factor) was included in our model, but depth was restricted to shallow and intermediate because deep water releases could not be done at the second site. Probabilities associated with the within-subjects terms had the Greenhouse-Geisser correction applied to reduce the likelihood of a type 1 error, the rate of which increases if the assumption of sphericity is not met. A third analysis, regression of average depth attained by S. punctata versus the time (days) since entering Port Phillip Bay, was done to tease-out the contribution of the time that S. punctata had been in Port Phillip Bay to the variability in average depths at which fish swam. This analysis was restricted to fish recaptured in the intermediate depth zone, because this was the depth zone in which fish showed the greatest variability in swimming depths.
Variability in the net distance moved by fish was analysed using analyses of covariance. Depth zone and site were treated as fixed factors. The distances moved by the shallow and deep drogues, and that predicted by the S4, were treated as covariates in separate ANCOVAs.
We considered that fish would move in a mean direction that was influenced by local currents and their directions could be non-random. We therefore used a V-test (Zar 1974) to assess whether the direction moved by fish was (1) oriented in a particular direction, and (2) different to the directions of currents (measured by the S4) and of the deep and shallow drogues.
Results
A total of 132 fish was followed during the study, 109 of which were recaptured, and there was little difference in the standard lengths of fish among sites or depth zones (Table 1).
Fish behaviour
Fish actively swam throughout each follow. Immediately after release, fish oriented and began swimming towards the bottom. Fish which swam to the bottom, swam approximately 30 cm above the substratum until they reached small (≈10–20 cm in diameter) patches of unvegetated sand or rubble. Within these patches, fish 'held' their position within the boundary layer by swimming continuously into a current, which, close to the substratum, appeared to be reduced by the arborescent macroalgae, larger rocks and sponges surrounding the patch of sand. At Indented Heads, where there was very little vertical relief, fish moved to the bottom soon after release, particularly at the shallow location. They continued to swim with a great deal of effort into the prevailing current, but after a few minutes, not having found any type of shelter in the form of algae or rocks, all fish returned to the surface, once more orienting into the current, but reducing their swimming activity.
Regardless of the site or the depth of water into which they were released, fish oriented into the current within 3–5 s of release. Fish continued to swim into the current for the duration of each follow. During the only period of our study when tidal streaming was reduced (during a period of tidal-change), fish did not appear to orient in any particular direction, and certainly not into any current that was discernible to the diver. In deep water, where the diver could not see the bottom, the direction of the current could be determined from the largely passive movement of the diver and support boat relative to fixed landmarks. The bottom in the shallow and intermediate depth zones was always visible to the diver, albeit not always clearly in the intermediate zone, but the bottom was never visible to the diver in the deep depth zone.
Fish that remained in the water column appeared to be attracted to a variety of inanimate floating objects, but generally only stayed close to seagrass fronds that were hanging vertically in the water column as they drifted with the currents. They rarely stayed with these for more than 2 min. Fish did not aggregate with other S. punctata post-larvae that happened to swim through the diver's field of view.
Vertical movement
At least some fish at all sites went to the bottom, while others stayed at the surface (see the example profiles in Fig. 2). Those fish that swam to the bottom generally did so within the first minute after release, although several fish approached the bottom only during the last 2 min. Several fish also approached the bottom (especially at the unvegetated site) and then returned to the surface. This pattern was most common in the intermediate depth zone.
At Grassy Point, the average depth chosen by fish varied negatively with the maximum depth of the zone in which fish were released, and there were large differences among depths (F 2,13=14.278, P=0.001; Fig. 3). The average depth of fish in the deep depth zone was significantly less than the average in either the intermediate (P=0.031) or shallow zones (P<0.001), but there was no difference in the average depth of fish between the intermediate and shallow zones (P=0.090). The vertical positions of fish through the observation periods were independent of depth (time × depth interaction, Greenhouse-Geisser Epsilon=0.1888, F 18,117=0.906, adjusted P=0.465), but their time-averaged positions were very different (Greenhouse-Geisser Epsilon=0.1888, F 9,117=4.013, adjusted P=0.038). In the shallow zone, 86% of fish swam directly to the bottom within 2 min, where they stayed for the remainder of the follow. Fish released over water of intermediate depth moved throughout the water column, although individuals spent most (>60%) of their time in one particular depth zone. Similar numbers of fish moved to the surface, in mid water and close to the bottom. In deep water, where divers could not see the bottom from the surface, 81% of fish stayed within the top 1.2 m of water. Diver observations indicated that within this surface layer, however, fish actually remained within 10 cm of the surface.
To determine whether patterns were consistent between sites, we excluded the deep zone from Grassy Point, and compared patterns in the other two zones at both sites. In this combined analysis, the vertical movement of fish through time was independent of both site (time × site interaction: Greenhouse-Geisser Epsilon=0.2252, F 9,72=0.775, adjusted P=0.479) and depth zone (time × depth-zone interaction: Greenhouse-Geisser Epsilon=0.2252, F 9,72=0.683, adjusted P=0.521), as was the overall depth (F 1,8=1.155, P=0.314; Fig. 3) and site (F 1,8=1.015, P=0.343; Fig. 3).
The fish from the intermediate depth zone at Grassy Point showed the most marked variation in vertical movements, but very little of the variability in average depths chosen by the fish in this zone could be explained by the time (days) since the fish had entered Port Phillip Bay (df 1,14, F=0.016, P=0.903, R 2=0.001, Fig. 4). One of the data points had considerable leverage (0.466), but re-analysis without this data point (df 1,13, F=0.003, P=0.958, R 2<0.001) made no difference to the conclusion that the depth attained by fish was largely unrelated to the time (days) since fish had entered Port Phillip Bay.
Horizontal movement
Distance
The distances moved by fish varied significantly between depth zones at Grassy Point (F 2,13=14.830, P<0.001; Fig. 5); distances moved by fish in the deep zone were significantly greater than those moved in the shallow zone (P<0.001), but not the intermediate depth zone (P=0.142). Fish moved further in the intermediate zone than they did in the shallow zone (P=0.017; Fig. 5). The variation in distance moved was not clearly related to the three possible predictor variables. For the S4 data, the covariate effect varied significantly with depth (F 2,11=4.793, P=0.032). In the deep zone, there was a significant and positive relationship between the distances moved by fish and those predicted from the S4 (F 1,4=11.053, P=0.029, R 2=0.734; Fig. 6). In the intermediate zone, only 38% of the variability in distances moved by fish could be explained by the relationship with the distance predicted from the S4, and this was not significantly different from 0 (F 1,3=1.802, P=0. 270; Fig. 6). In the shallow zone, little of the variability in distances moved by fish could be explained by the relationship with the distance predicted from the S4 (F 1,3=0.006, P=0.0.942, R 2=0.002; Fig. 6). The shallow drogue was a slightly more consistent predictor (depth × covariate interaction F 2,12=3.656, P=0.061), but explained only 6% of the variability in distance moved by fish (F 1,14=0.925, P=0.352). Little of the variability in the distance moved by fish could be explained by the distance moved by the deep drogue (F 1,12=0.404, P=0.537).
When both sites were included in the model and depth zones were restricted to shallow and intermediate zones, fish moved significantly greater distances at Indented Heads than Grassy Point (F 1,8=8.117, P=0.022), and in the intermediate rather than shallow depth zones (F 1,8=8.016, P=0.022; Fig. 5). None of the covariates interacted significantly with either depth zone or site (P>0.05), nor explained a significant amount of the variability in the distance moved by fish; shallow drogue (F 1,7=0.371, P=0.562), deep drogue (F 1,7=0.038, P=0.854), distance predicted from the S4 (F 1,7=0.810, P=0.398).
Direction
The directions moved by fish at each site depended on whether they remained in the water column or swam to the bottom, which in turn depended on the depth of the water into which they were released. In deep water, fish moved in a non-random direction, and on most occasions, there was little difference between the direction moved by fish and that moved by the shallow/deep drogues, or given by the S4 (Table 1). In the shallow depth zone, and on most occasions in the intermediate depth zone, however, fish moved in random directions, which were little related to the movement of the drogues or currents (Table 1).
In the deep zone at Grassy Point, fish moved in directions significantly different from random on five out of six sampling occasions (Rayleigh's z-test, df=6, P<0.01; Table 1). In each set of follows, the mean direction moved by fish was significantly related to the direction moved by the shallow and deep drogues during the last follow (Rayleigh's R-test, df=6, P<0.05; Table 1). The mean direction moved by fish in deep water was similar to that measured by the S4 on all but one occasion (Rayleigh's R-test, df=6, P<0.05; Table 1), when the mean bearing measured by the S4 was 185º, while fish moved at a bearing of 100°. In the intermediate depth zone, fish moved in random directions on two out of five sampling occasions (Rayleigh's z-test, df=6, P>0.1; Table 1); fish moved in directions significantly different from random on 3 occasions (Rayleigh's z-test, df=6, P<0.01; Table 1). When fish moved in directions different from random, the directions moved by the shallow and deep drogues were similar on the first and second sampling occasions (Rayleigh's R-test, df=6, P<0.05; Table 1). Non-random directional movements by fish were similar to the mean bearing recorded by the S4 on the first and third sampling occasions. On these occasions where the direction of fish displacement was significantly related to the direction of the shallow or deep drogues, or the predictions from the S4, fish were less than 1 m from the surface for most of the 10 min follow. In the shallowest depth zone, fish direction was highly significantly random on four out of five sampling occasions (Rayleigh's z-test, df=6, P>0.2; Table 1) and less significantly random on the fifth (Rayleigh's z-test, df=6, P>0.05; Table 1). At no time was the bearing of the shallow or deep drogues, or the S4 similar to the bearing of the fish (Rayleigh's R-test, df=6, P>0.05; Table 1).
At Indented Heads, fish moved in non-random directions only once in the intermediate zone, but on all three occasions in shallow water (Table 1). There did not appear to be any consistent pattern, however, in whether the direction moved by fish, if non-random, varied with currents (Table 1).
Discussion
Secondary planktonic dispersal is considered to be an important process structuring assemblages of some invertebrates soon after settlement (Cummings et al. 1995; Christy and Morgan 1998; Etherington and Eggleston 2000), but it has been little studied with respect to how it may alter initial settlement patterns in fish. In our study, there appeared to be a degree of plasticity in the vertical positions "chosen" by fish amongst different depth zones. In shallow water, Sillaginodes punctata commonly swam directly to the bottom. This type of vertical movement has also been found in species of pomacentrids, lethrinids and serranids (Leis et al. 1996). Conversely, in the deepest depth zone (7 m), fish would orient and begin swimming toward the bottom directly after release, but cease descent at a depth of around 1.5 m and return to within 30 cm of the surface. This is in contrast to work by Leis and Carson-Ewart (2000), who found differences in vertical distribution between a lagoon and an atoll in four species of coral reef fish, three of which swam deeper in the ocean (a deep site) than in the lagoon (shallower water). At present, we have no way of knowing why fish did not swim to the bottom at deeper sites, and what depth (if ever) they could actually sense the bottom at. Fish use a variety of senses to determine their position in the water column and larvae are thought to be able to sense coral reefs up to the scale of km's, possibly by hearing, smell or chemical cues (Leis et al. 1996). Perhaps they can sense the bottom in deep water, but avoid it because there is likely to be a greater risk of mortality. Hindell et al. (2002) have found that post-larval S. punctata are more abundant in areas without predatory fish, and the few post-larvae that moved to the bottom in deep water were pursued by predatory odacids (J. Hindell, personal observation). Conversely, perhaps because fish were collected from a demersal environment, they were in some way habituated to the bottom and fish simply swim down for a fixed time and, if they don't hit the bottom, turn around. This is purely speculative, however, and further research is needed to explain the behavioural observations. Regardless of the mechanism/s generating this behaviour, there is a significant degree of plasticity in their response, and this has important implications as to the likelihood of secondary dispersal.
Several species of invertebrate have coastal planktonic phases that enter estuaries to settle by 'riding' on flood tides; during ebb tides the animals vary their position in the water so that their movement out of the estuary is minimised (Christy and Morgan 1998; Etherington and Eggleston 2000). This type of movement has been termed saltatory recruitment (Christy and Morgan 1998), short periods of settlement punctuated by episodic dispersal of the post-larvae. In these studies, re-entrainment is via behaviourally driven vertical migration, but it is possible that disturbance events, particularly wave action, may also play a significant role in the re-entrainment of post-larval fishes. Episodic disturbance by wave action in shallow seagrass beds is known to translocate post-larval S. punctata offshore after re-entraining them into the water column; during conditions of off-shore winds and little wave action (calm conditions), S. punctata post-larvae are less susceptible to offshore movement (S. Moran et al., unpublished data). The depth profiles of fish in our study show that whether or not these fish are then dispersed to other regions depends strongly on their vertical behaviour and how far they are transported offshore. The vertical positions 'chosen' by fish in our study facilitated dispersal in the deep zone but prevented dispersal in the shallow zone. All but two fish in the deepest zone remained within 1 m of the surface and were displaced over much greater distances than those in the shallowest depth zone, which swam directly to the bottom, oriented into a current that was greatly reduced by vertical relief from the reef, and remained in approximately one position. Unless a disturbance event, in our case wave action, can transport fish into deeper regions, fish are able to resist further dispersal. If translocated into deeper water, however, they can be dispersed large distances by currents in what appears to be a largely passive manner.
Over the past decade, tropical and temperate reef fish larvae have been shown to be far from passive. They have swimming speeds which allow directional motion and control over their vertical position in the water column (Kingsford 1988; Leis 1991). The direction and magnitude of horizontal displacement of post-larvae in relation to that of the currents in our study suggested that dispersal was passive. The directions moved by drogues, and the direction predicted from the S4 current meter, were not significantly different from the directions moved by fish in the deepest depth zone, where fish moved the greatest distances. On several occasions, however, the magnitude of displacement of fish was significantly less than that predicted by the drogues or S4 current meter, even though the directions moved by each were similar. Our results suggest that the net movement of fish was the product of currents and swimming.
Larval displacement in the water column results from the interactive effects of hydrodynamics and larval swimming (Metaxas 2001), but few of the coral reef studies of fish behaviour have attempted to tease-out the contribution of current direction on fish movement in-situ, although Leis and Carson-Ewart (2000) have measured the current speed (based on the drifting velocity of a diver) in relation to the swimming speed of fish. The net movement observed in coral reef fish larvae in-situ is commonly attributed to active swimming, but in our study, the net movement of fish was a product of the current versus swimming, the directions of which were diametrically opposed. Diver observations in the deepest depth zone showed that fish actively swam through the water column, but this had little influence on the direction they moved because they swam directly into a current whose velocity was larger than that generated by the swimming of fish. Given the similarity in the depth of the shallow drogue and that at which fish swam in the deep zone, the shallow drogue should have been the best predictor of distance moved. But that the average net distance moved by fish was less than that moved by the shallow drogue on four of the five sampling occasions, is consistent with diver observations that fish swam into the current, and did not simply drift along as passive particles. While Leis and colleagues have shown that fish orient in directions relative to potential settlement sites (Leis et al. 1996; Leis and Carson-Ewart 1999), orientation in our fish was driven by local currents. How fish determine which direction the current is flowing in, or why they swim into it are unknown. Leis et al. (1996, and references therein) have speculated at length about the various cues and senses that fish might be using to detect movement of the water column, and clearly, this is an area of great uncertainty. More work like that of Leis and his colleagues (Leis et al. 2002), who are attempting to separate the relative contributions of visual, olfactory and auditory cues in shaping the movement and behaviour of settlement-stage larval fish, is needed to better understand how fish can determine their relative orientation with respect to currents.
The recruitment of S. punctata into patches of shallow seagrass around the periphery of Port Phillip Bay has been modelled by Jenkins and his colleagues (Jenkins and Black 1994; Jenkins et al. 1999). In these models, fish have been treated as largely passive particles, although scenarios of vertical migration and weak tidal migration were also considered. Although correlations with observed larval distributions were high, there were patterns in the field that could not be explained by the model (Jenkins et al. 1999). Modelling of larval dispersal into Port Phillip Bay was unable to produce the observed recruitment pattern deep within the Geelong Arm (Jenkins et al. 1997, 1999). If, however, Grassy Point was designated as the larval source, a very good correlation between model predictions and actual recruitment within the Geelong Arm was observed (Jenkins et al. 1999). This study shows that S. punctata post-larvae in seagrass, if re-entrained into the pelagic environment by on-shore weather conditions and increased disturbance, can possibly be dispersed further into Port Phillip Bay. Re-entrainment of post-larvae from seagrass to the water column may also explain the higher than expected numbers of settlement-stage larvae near to the western-side of the Bay (Jenkins et al. 1999). The entrainment of post-larvae into the water column would create a diffusive gradient offshore from the seagrass.
An important assumption of this type of study is that the behaviour of fish is not altered by the SCUBA diver (for review of tropical fish studies see Leis and McCormick 2002). In our study, several lines of evidence support this assumption. Firstly, during the SCUBA follows, fish swam at speeds much less than their maximum. Only when the diver attempted to catch the fish at the end of the SCUBA follow did the fish alter their behaviour by swimming erratically and quickly away from the diver. Secondly, on several occasions, divers observed S. punctata post-larvae that were in the area without the diver putting them there. These 'natural' fish behaved in much the same ways as the fish that were released. Thirdly, fish were frequently observed to be feeding, and they often changed their behaviour to suit different situations (e.g. swimming toward blades of seagrass floating in the water column and staying with it for short periods of time). We would not have expected to observe these subtle behaviours if the fish were reacting to SCUBA divers rather than their immediate environment.
Conclusions
Dispersal is often modelled with larvae acting as passive particles, but our study has shown that, depending on the depth of the water into which they are released, this is likely to be problematic for two reasons. Firstly, in shallow water, S. punctata have strong vertical movements towards the bottom, where they can reduce their horizontal displacement by staying out of the current. Secondly, even where S. punctata remain in the water column, they swim continuously against the direction of water movement, and the displacement of drogues (or that predicted from currents) sometimes overestimates the actual displacement of fish. Alternatively, in spite of their swimming ability, when fish stay at the surface, the horizontal direction in which they move is usually similar to the directions moved by drogues and currents, and is thus more consistent with their treatment as passive particles. Episodic disturbance events can re-entrain post-larval fish in the water column, and, depending on how quickly fish are translocated into regions of deep water, secondary dispersal in the pelagic environment is potentially an important source of variability in initial recruitment patterns.
References
Armsworth PR (2000) Modelling the swimming response of late stage larval reef fish to different stimuli. Mar Ecol Prog Ser 195:231–247
Bruce BD (1995) Larval development of King George whiting, Sillaginodes punctata, school whiting, Sillago bassensis, and yellow fin whiting, Sillago schomburgkii (Percoidei: Sillaginidae), from South Australian waters. Fish Bull 93:27–43
Caley MJ, Carr MH, Hixon MA, Hughes TP, Jones GP, Menge BA (1996) Recruitment and the local dynamics of open marine populations. Annu Rev Ecol Syst 27:477–500
Christy J, Morgan S (1998) Estuarine immigration by crab postlarvae: mechanisms, reliability and adaptive significance. Mar Ecol Prog Ser 174:51–65
Cummings V, Pridmore R, Thrush S, Hewitt J (1995) Post-settlement movement by intertidal benthic macroinvertebrates: do common New Zealand species drift in the water column. NZ J Mar Freshw Res 29:59–67
Doherty PJ, Williams DM (1988) The replenishment of coral reef fish populations. Ocean Mar Biol Annu Rev 26:487–551
Dudley B, Tolimieri N, Montgomery J (2000) Swimming ability of the larvae of some reef fishes from New Zealand waters. Mar Freshw Res 51:783–787
Etherington LL, Eggleston DB (2000) Large-scale blue crab recruitment: linking postlarval transport, post-settlement planktonic dispersal, and multiple nursery habitats. Mar Ecol Prog Ser 204:179–198
Fowler AJ, Black KP, Jenkins GP (2000) Determination of spawning areas and larval advection pathways for King George whiting in southeastern Australia using otolith microstructure and hydrodynamic modelling. II. South Australia. Mar Ecol Prog Ser 199:243–254
Heath M, Zenitani H, Watanabe Y, Kimura R, Ishida M (1998) Modelling the dispersal of larval Japanese sardine, Sardinops melanostictus, by the Kuroshio Current in 1993 and 1994. Fish Oceanogr 7:335–346
Hindell J, Jenkins G, Keough M (2002) Variability in the numbers of post-settlement King George whiting (Sillaginidae: Sillaginodes punctata, Cuvier) in relation to predation, habitat complexity and artificial cage structure. J Exp Mar Biol Ecol 268:13–31
Hixon MA (1998) Population dynamics of coral-reef fishes: controversial concepts and hypotheses. Aust J Ecol 23:192–201
Jenkins GP (1986) Composition, seasonality and distribution of ichthyoplankton in Port Phillip Bay, Victoria. Aust J Mar Freshw Res 37:507–520
Jenkins GP, Black KP (1994) Temporal variability in settlement of a coastal fish (Sillaginodes punctata) determined by low-frequency hydrodynamics. Limnol Oceanogr 39:1744–1754
Jenkins GP, May HMA (1994) Variation in settlement and larval duration of King George Whiting, Sillaginodes punctata (Sillaginidae), in Swan Bay, Victoria, Australia. Bull Mar Sci 54:281–296
Jenkins GP, Welsford D (2002) The swimming abilities of recently settled post-larvae of Sillaginodes punctata. J Fish Biol 60:1043–1050
Jenkins GP, Wheatley MJ (1998) The influence of habitat structure on nearshore fish assemblages in a southern Australian embayment: Comparison of shallow seagrass, reef-algal and unvegetated sand habitats, with emphasis on their importance to recruitment. J Exp Mar Biol Ecol 221:147–172
Jenkins GP, Wheatley MJ, Poore AGB (1996) Spatial variation in recruitment, growth and feeding of post-settlement King George whiting, Sillaginodes punctata, associated with seagrass beds of Port Phillip Bay, Australia. Can J Fish Aquat Sci 53:350–359
Jenkins GP, Black KP, Wheatley MJ, Hatton DN (1997) Temporal and spatial variability in recruitment of a temperate, seagrass-associated fish is largely determined by physical processes in the pre- and post-settlement phases. Mar Ecol Prog Ser 148:23–35
Jenkins GP, Keough MJ, Hamer PA (1998) The contribution of habitat structure and larval supply to broad-scale recruitment variability in a temperate zone, seagrass-associated fish. J Exp Mar Biol Ecol 226:259–278
Jenkins GP, Black KP, Keough MJ (1999) The role of passive transport and the influence of vertical migration on the pre-settlement distribution of a temperate, demersal fish: model predictions compared with field sampling. Mar Ecol Prog Ser 184:259–271
Jenkins GP, Black KP, Hamer PA (2000) Determination of spawning areas and larval advection pathways for King George whiting in southeastern Australia using otolith microstructure and hydrodynamic modelling. I. Victoria. Mar Ecol Prog Ser 199:231–242
Jones GP (1991) Postrecruitment processes in the ecology of coral reef fish populations: a multifactorial perspective. In: Sale PF (ed) The ecology of fishes on coral reefs. Academic Press, San Diego, Calif., pp 294–328
Kingsford MJ (1988) The early life history of fish in coastal waters of northern New Zealand: a review. NZ J Mar Freshw Res 22:463–479
Leis JM (1986) Vertical and horizontal distribution of fish larvae near coral reefs at Lizard Island, Great Barrier Reef. Mar Biol 90:505–516
Leis JM (1991) The pelagic stage of reef fishes: the larval biology of coral reef fishes. In: Sale PF (ed) The ecology of fishes on coral reefs. Academic Press, New York, pp 183–230
Leis JM, Carson-Ewart BM (1999) In situ swimming and settlement behaviour of larvae of an Indo-Pacific coral-reef fish, the coral trout Plectropomus leopardus (Pisces: Serranidae). Mar Biol 134:51–64
Leis JM, Carson-Ewart BM (2000) Behaviour of pelagic larvae of four coral-reef fish species in the ocean and an atoll lagoon. Coral Reefs 19:247–257
Leis JM, McCormick MI (2002) The biology, behaviour and ecology of the pelagic, larval stage of coral-reef fishes. In Sale PF (ed) Coral reef fishes: dynamics and diversity in a complex ecosystem. Academic Press, San Diego, Calif., pp 171–199
Leis JM, Sweatman HPA, Reader SE (1996) What the pelagic stages of coral reef fishes are doing out in blue water: daytime field observations of larval behavioural capabilities. Mar Freshw Res 47: 401–411
Leis JM, Carson-Ewart B, Webley J (2002) Settlement behaviour of coral-reef fish larvae at subsurface artificial-reef moorings. Mar Freshw Res 53:319–327
Metaxas A (2001) Behaviour in flow: perspectives on the distribution and dispersion of meroplankton larvae in the water column. Can J Fish Aquat Sci 58:86–98
Milicich MJ, Meekan MG, Doherty PJ (1992) Larval supply: a good predictor of recruitment of three species of reef fish (Pomacentridae). Mar Ecol Prog Ser 86:153–166
Proctor R, Wright PJ, Everitt A (1998) Modelling the transport of larval sand eels on the north-west European shelf. Fish Oceanogr 7:347–354
Stobutzki I, Bellwood DR (1997) Sustained swimming abilities of the late pelagic stages of coral reef fishes. Mar Ecol Prog Ser 149:35–41
Zar JH (1974). Biostatistical analysis. Prentice-Hall, Englewood Cliffs, N.J.
Acknowledgments
Earlier versions of this manuscript were improved by comments from J. Leis, T. Trnksi and anonymous reviewers. D. Ball calculated the directions and distances moved by fish. S. Blake, S. Berrie and G. Werner helped in the field. This research was funded by the Australian Research Council and was done using the facilities at the Marine and Freshwater Resources Institute.
Author information
Authors and Affiliations
Corresponding author
Rights and permissions
About this article
Cite this article
Hindell, J.S., Jenkins, G.P., Moran, S.M. et al. Swimming ability and behaviour of post-larvae of a temperate marine fish re-entrained in the pelagic environment. Oecologia 135, 158–166 (2003). https://doi.org/10.1007/s00442-003-1180-0
Received:
Accepted:
Published:
Issue Date:
DOI: https://doi.org/10.1007/s00442-003-1180-0